the Creative Commons Attribution 4.0 License.
the Creative Commons Attribution 4.0 License.
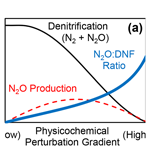
Physicochemical perturbation increases nitrous oxide production from denitrification in soils and sediments
Nathaniel B. Weston
Cynthia Troy
Patrick J. Kearns
Jennifer L. Bowen
William Porubsky
Christelle Hyacinthe
Christof Meile
Philippe Van Cappellen
Samantha B. Joye
Atmospheric concentrations of nitrous oxide (N2O), a potent greenhouse gas that is also responsible for significant stratospheric ozone depletion, have increased in response to the intensified use of agricultural fertilizers and other human activities that have accelerated nitrogen cycling processes. Microbial denitrification in soils and sediments is a major source of N2O, produced as an intermediate during the reduction of oxidized forms of nitrogen to dinitrogen gas (N2). Substrate availability (nitrate and organic matter) and environmental factors such as oxygen levels, temperature, moisture, and pH influence rates of denitrification and N2O production. Here we describe the role of physicochemical perturbation (defined here as a change from the ambient environmental conditions) in influencing rates of denitrification and N2O production. Changes in salinity, temperature, moisture, pH, and zinc in agricultural soils induced a short-term perturbation response characterized by lower rates of total denitrification and higher rates of net N2O production. The ratio of N2O to total denitrification (N2O : DNF) increased strongly with physicochemical perturbation. A salinity press experiment on tidal freshwater marsh soils revealed that increased N2O production was likely driven by transcriptional inhibition of the nitrous oxide reductase (nos) gene and that the microbial community adapted to altered salinity over a relatively short time frame (within 1 month). Perturbation appeared to confer resilience to subsequent disturbance, and denitrifiers from an environment without salinity fluctuations (tidal freshwater estuarine sediments) demonstrated a stronger N2O perturbation response than denitrifiers from environments with more variable salinity (oligohaline and mesohaline estuarine sediments), suggesting that the denitrifying community from physicochemically stable environments may have a stronger perturbation response. These findings provide a framework for improving our understanding of the dynamic nature of N2O production in soils and sediments, in which changes in physical and/or chemical conditions initiate a short-term perturbation response that promotes N2O production that moderates over time and with subsequent physicochemical perturbation.
- Article
(3685 KB) - Full-text XML
- BibTeX
- EndNote
Human activities continue to accelerate the global nitrogen (N) cycle through the industrial fixation of dinitrogen gas (N2) for use as an agricultural fertilizer, increased cultivation of N-fixing crops, and combustion of fossil fuels (Galloway et al., 2004). As a result, the availability of reactive N continues to increase in terrestrial and aquatic systems worldwide. Because many ecosystems are N-limited (Vitousek and Howarth, 1991), increased levels of reactive N in the biosphere can have deleterious impacts, including the eutrophication of inland and coastal waters (Nixon, 2009). Denitrification is an anaerobic pathway of microbial respiration that removes reactive N through the reduction of inorganic nitrogen (nitrate, NO, or nitrite, NO) to unreactive dinitrogen gas (N2; Payne, 1973; Knowles, 1982; Seitzinger, 1988). The complete reduction of NO to N2 occurs in several steps that require the reduction of the intermediate gases nitric oxide (NO) and nitrous oxide (N2O) and is accomplished by a series of enzymatic reactions catalyzed by NO reductase (Nar), NO reductase (Nir), NO reductase (Nor), and N2O reductase (Nos; Knowles, 1982). N2O is produced transiently during denitrification, and some N2O escapes reduction and is emitted from zones of active denitrification to overlying waters and/or the atmosphere (Seitzinger, 1988). The increase in global reactive N fuels greater rates of denitrification, resulting in increased emissions of N2O from soils, sediments, and waters (Denman et al., 2007; Beaulieu et al., 2011; Tian et al., 2020). N2O is also produced through fungal denitrification (Maeda et al., 2015); microbial nitrification (Davidson et al., 1986); chemodenitrification (abiotic denitrification; Grabb et al., 2017; Robinson et al., 2021); and, possibly, dissimilatory nitrate reduction to ammonia (Butterbach-Bahl et al., 2013). The contribution of these processes to global N2O budgets is less clear, but in many instances where direct comparisons have been made, bacterial denitrification is often the dominant N2O source from soils and sediments (Mathieu et al., 2006; Vilain et al., 2014; Hu et al., 2015). More recently, however, Bahram et al. (2022) found that archaeal nitrifiers may play a more important role in N2O production in soils than previously recognized. In the troposphere, N2O is a potent greenhouse gas with a global warming potential 298 times that of carbon dioxide over a 100-year time frame (Forster et al., 2007). Concentrations of N2O in the atmosphere have risen by more than 18 %, with an estimated increase of roughly 0.26 % per year from 1980 through 2005 (Forster et al., 2007). In addition, N2O is currently the single most important ozone-depleting atmospheric trace gas and is expected to remain so throughout the 21st century (Ravishankara et al., 2009). Given the potency of N2O as a greenhouse gas and ozone-depleting substance, a better understanding of N2O production dynamics in the geosphere is needed (Wuebbles, 2009).
Despite the importance of N2O to climate change and stratospheric ozone dynamics, the factors that regulate net N2O production from soils and sediments during denitrification (DNF; defined here as the sum of N2O and N2 production) remain unclear, and we do not yet know why the ratio of N2O production to total denitrification (N2O : DNF) varies in denitrifying environments. Denitrification rates are spatially and temporally heterogeneous in soils and sediments, resulting in “hotspots” and “hot moments” of activity (McClain et al., 2003; Groffman et al., 2009). Likewise, N2O emissions from soils vary considerably over space and time, and our ability to predict this variation is limited (Huang et al., 2011; Henault et al., 2012; Harrison-Kirk et al., 2013; Weitzman et al., 2021). Several environmental variables impact rates of denitrification and N2O emissions, including the availability of substrates (NO, labile organic matter, and other electron donors such as ferrous iron). In general, the proportion of N2O released from soils increases with increasing NO availability (Firestone et al., 1980; Barnard et al., 2005; Bao et al., 2012), and since denitrification is an anaerobic respiration process, it can be sensitive to oxygen (O2) concentrations and soil moisture levels, which affects O2 diffusion into soils (Firestone et al., 1980; Seitzinger, 1988; Conrad, 1996; Wang et al., 2023). Although rates of denitrification generally decline as oxygen concentrations increase (Knowles, 1982; Rosamond et al., 2012), the N2O : DNF ratio can increase with higher O2 availability (Firestone et al., 1980; Betlach and Tiedje, 1981; Burgin and Groffman, 2012).
In addition to substrate availability and O2, other soil/sediment physicochemical factors can influence rates of denitrification and N2O production. Soil pH exerts a potential control on rates of denitrification and N2O : DNF ratios (Firestone et al., 1980; Weslien et al., 2009; Baggs et al., 2010). Typically, under more acidic conditions, rates of denitrification are lower and the N2O : DNF ratio is higher (van den Heuvel et al., 2011; Raut et al., 2012). Liu et al. (2014) suggest that post-transcriptional inhibition of the nitrous oxide reductase enzyme under lower pH conditions was responsible for the greater relative N2O production. Similarly, increasing concentrations of heavy metals inhibit the reduction of N2O, leading to higher N2O fluxes (Magalhaes et al., 2007; Ruyters et al., 2010). Temperature (Seitzinger, 1988; Larsen et al., 2011; Billings and Tiemann, 2014), hydrogen sulfide (Porubsky et al., 2009), and salinity (Giblin et al., 2010; Teixeira et al., 2013) can also exert control over denitrification and N2O production. While physicochemical conditions can clearly influence denitrification, our understanding of how environmental controls impact denitrification coupled with N2O production remains limited (Butterbach-Bahl et al., 2013), and the resilience of microbial communities to changes in physicochemical conditions is not straightforward (Griffiths and Philippot, 2013). We addressed this knowledge gap by investigating whether pulse and press disturbances (Bender et al., 1984) that arise from changing physicochemical conditions elicit a perturbation response from the denitrifying community. We define perturbation as a deviation from ambient physicochemical conditions encountered by the denitrifying microbial community in soils or sediments. We explore how perturbation alters rates of denitrification, N2O production, N2O : DNF production ratio, and changes in the gene expression of nitrite reductase (nirS) and nitrous oxide reductase (nosZ) genes that code for key enzymes that mediate N2O production and consumption.
Several experiments were conducted to evaluate environmental controls on denitrification (defined as N2+ N2O production), N2O production, and the ratio of N2O to total denitrification (N2O : DNF). We conducted three discrete experiments (Table 1) that addressed (1) the short-term perturbation response induced by the manipulation of the physicochemical (salinity, temperature, pH, soil moisture, and zinc toxicity) status of agricultural soils, (2) the short-term response of denitrifying communities from environments experiencing a range in one parameter (salinity in estuarine sediments) to changes in that parameter, and (3) the long-term response (changes in process rates and gene expression) of the denitrifying community to a change in a single parameter (salinity in estuarine sediments). We elected to focus on changes in salinity in the second and third experiments because it is a parameter that changes over daily (tidal) and seasonal timescales in estuarine environments and is therefore ecologically relevant; it is a parameter that will be altered in some environments in response to climate change, and it is relatively easy to manipulate and to measure. We did not have the resources to investigate additional physicochemical parameters beyond the first experiment (Table 1). In all experiments, soils/sediments were incubated in oxygen-free, gas-tight headspace vials, and the production of N2O was measured with and without acetylene (Balderston et al., 1976; Yoshinari and Knowles, 1976; Groffman et al., 2006). N2O production rates without acetylene reflect N2O produced by the microbial community. Acetylene inhibits N2O reductase and thus blocks the final step in the denitrification process, resulting in the buildup of N2O rather than N2 (Balderston et al., 1976; Yoshinari and Knowles, 1976). N2O production rates with acetylene therefore reflect the total rate of denitrification (N2+ N2O; DNF). Headspace gas samples were taken several times (typically three to four times) during the incubation (see the Appendix, for example), and rates of denitrification and N2O production were calculated from the linear increase in N2O over time. Incubation times were relatively short (typically < 12 h) to avoid changes in denitrifier population, longer-term adaptation of the denitrifying community to changes in physicochemical disturbance, and changes in substrate concentrations. In the instances where nonlinearity in the production of N2O was observed due to these (or other) factors, the data from later time points were not used and only the linear portion of the time course incubations was used to calculate N2O production. Rates are reported as micromoles of N2+ N2O (DNF) or N2O per gram of fresh soil/sediment per day (µmol g−1 d−1). The ratio of N2O produced to total denitrification (N2O : DNF) was calculated on a per-mole basis.
Table 1Summary of the three experiments in which the response of denitrification and nitrous oxide production to physicochemical perturbation was investigated (experiment number corresponds to the subsections in the Methods section).
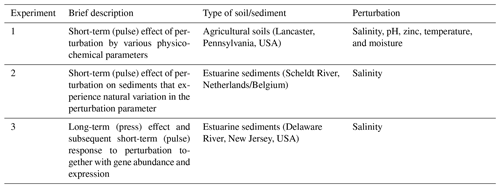
2.1 Agricultural soils – salinity, pH, zinc, temperature, and moisture pulse perturbations
Experiments were conducted on agricultural soil samples collected from two sites in July 2011, one farmed conventionally (40.07464° N, 76.212008° W) and one farmed using organic practices (40.069779° N, 76.238079° W), in Lancaster, PA. Surface soils (0–2 cm) were collected from each site. The temperature of the surface soils (37 °C) was measured at the time of collection. The soils were returned to the laboratory and homogenized, and visible roots were removed. The soil water content (0.48 g g−1 for the conventional soil and 0.46 g g−1 for the organic soil) was determined by the loss of weight upon drying at 80 °C for 48 h, and soil pH (7.37 in the conventional soil and 7.09 in the organic soil) was measured with a pH probe after mixing 20 g of soil with 25 mL of deionized water. For each perturbation assay, approximately 20 g of soil was placed into a 410 mL headspace jar, and treatments with varying salinity, pH, zinc, temperature, and moisture were performed to evaluate changes in N2O production and denitrification rates (Table 1). All experiments except for the moisture treatment received 10 mL of water, and all treatments were amended with 1 mM NO and 2 mM glucose.
A series of jars were amended to achieve various salinities (0, 1, 3, 5, 10, and 30 g kg−1) using an artificial saltwater solution (350 mM NaCl, 45.5 mM MgCl2, 24.2 mM Na2SO4, 8.9 mM CaCl2, 2 mM NaHCO3, and 0.5 mM KCl for salinity of 30 g kg−1 and diluted as appropriate with deionized water for other salinities). Similarly, a series of jars were amended to obtain various zinc concentrations (0, 0.05, 0.1, 0.25, 0.5, and 1.0 g Zn L−1 in deionized water) by addition of a zinc chloride solution. pH treatments were achieved by amending the pH of the soil solution by additions of dilute hydrochloric acid or sodium hydroxide (in deionized water) to achieve deviations from ambient pH to +1, +2, +3, −1, −2, −3, and 0 (reference). For the temperature treatments, 10 mL of deionized water was added to jars which were incubated at a range of temperatures (20, 30, 37, 43, and 52 °C) to achieve positive and negative deviations from the ambient temperature (37 °C). Moisture treatments were achieved by air-drying soils for several days and adding various amounts of deionized water to the dry soil to achieve soil moisture treatments with approximately 0.0, 0.05, 0.09, 0.17, 0.33, and 0.50 g water per gram of soil (weight : weight).
Six jars were prepared for each treatment for each of the two soils. All jars were purged with N2 gas to remove oxygen, and three jars of each treatment received acetylene (10 %). Jars were incubated for approximately 12 h at ambient temperature (37 °C; except for the temperature treatments), and the headspace was sampled several times to determine N2O production rates as described above. For the temperature treatments, jars were incubated for times ranging from 8 h (43 and 52 °C treatments) to 24 h (20 °C treatment) to allow for adequate biogeochemical activity and N2O production across the range of temperatures. The laboratory incubations for each of the five perturbation parameters were conducted on different days over a period of several weeks, and therefore the rates in the reference treatment for each perturbation assay should not be compared. Headspace samples were taken using 10 mL syringes with a gas-tight valve, and N2O was determined on an Agilent Technologies 6850 Series II electron capture gas chromatograph within a day of collection.
2.2 Estuarine sediments – salinity pulse perturbation
We examined the denitrifier perturbation response to pulse disturbance induced by a single physicochemical parameter (salinity) in environments that naturally experience a range in that parameter (estuarine sediments; Table 1). Sediments were sampled from three locations along the salinity gradient (ambient salinities of 0, 5, and 24 g kg−1) in the Scheldt River estuary in Brussels and the Netherlands, and we assessed rates of sediment denitrification and N2O production across a range of salinities (from 0 to 30 g kg−1). Intact sediment cores were collected from freshwater (Appels; salinity 0 g kg−1 at time of collection; 51.030309° N, 4.041905° E), oligohaline (Waarde; salinity 5 g kg−1 at time of collection; 51.410664° N, 4.068669° E), and mesohaline (Rattekaai; salinity 24 g kg−1 at time of collection; 51.449888° N, 4.195477° E) sites. The freshwater Appels site occupies the tidal freshwater region of the Scheldt River which is uniformly fresh (van Damme et al., 2005). The oligohaline Waarde site is in the Westerschelde Estuary, into which the Scheldt River drains, with salinities ranging from 2 to 25 g kg−1 (van Damme et al., 2005). The mesohaline Rattekaai site is located in the Oosterschelde, which Gerringa et al. (1998) report has higher salinities (around 30 g kg−1) that are less variable because of little freshwater input.
Sediment cores were sectioned, and approximately 2 g of surface (0–2 cm) sediment and 10 mL of water were placed into 38 cm3 headspace vials. The salinity of the water added to the vials was amended by mixing 0.7 µm filtered freshwater (salinity = 0 g kg−1; collected from the Appels site) and seawater (salinity = 30 g kg−1; collected from the Scheldt Estuary). Sediments from the freshwater Appels sites were incubated under salinities of 0 (ambient), 1, 3, 5, 10, 15, and 30 g kg−1; the oligohaline Waarde site was incubated under salinities of 0, 1, 3, 5 (ambient), 10, 15, and 30 g kg−1; and the mesohaline Rattekaai site was incubated under salinities of 0, 3, 5, 10, 24 (ambient), and 30 g kg−1 (n=6 for each sediment and salinity treatment). All treatments also received 4 mM glucose and 2 mM NO.
After purging each vial with He to remove oxygen, the headspace of three vials for each treatment was amended with 10 % acetylene. Vials were then incubated for approximately 24 h at room temperature (20 °C). Gas samples from the headspace of each vial were removed at several time points during the incubation into 10 mL evacuated headspace vials. The concentration of N2O was determined by electron capture gas chromatography on a Shimadzu GC8 gas chromatograph within 1 month of collection.
2.3 Estuarine soils – press–pulse salinity perturbation
We investigated the response to long-term changes (over ∼6 months) in physicochemical conditions (press perturbation) to contrast with the pulsed perturbation response described above (Table 1). Surface (0–2 cm) soils from a tidal freshwater marsh on the Delaware River estuary (Rancocas Creek; 39.9888002° N, 74.84483° W) were collected, and 0.75 L of soil was mixed with 0.75 L of either artificial freshwater or saline water (using salts as described in Sect. 2.1) to achieve long-term incubation (press) salinities of S = 0 g kg−1 (control) or S = 20 g kg−1 (press treatment). Duplicates of each treatment were incubated for 6 months in stoppered flasks with an oxygen-free headspace (purged with N2 gas) with gentle mixing. To alleviate any substrate limitation over the incubation period, the jars were amended with 0.4 mM NO and 0.8 mM glucose weekly.
On days 0, 7, 14, 21, 35, 49, 70, 110, and 181, the long-term incubations were subsampled into smaller vials for short-term assays of denitrification and N2O production. In total, 10 mL of the soil solution was subsampled into a 410 mL headspace vial, and 10 mL of the appropriate salinity water was added to each vial along with 0.4 mM NO and 0.8 mM glucose. Due to differences in starting salinity from the press treatments, the salinities after amendment that were assayed for the S = 0 and S = 20 press treatment were 0.0, 4.3, 7.6, 16.9, and 25.6 g kg−1 (for S = 0) and 3.5, 7.4, 11.6, 20.0, and 28.3 g kg−1 (for S = 20). Four oxygen-free vials for each press–pulse combination were prepared by purging the headspace with N2, and acetylene (10 % final volume) was added to two vials. Vials were incubated for < 12 h, and the production of N2O was determined by subsampling the vials using 10 mL syringes with a gas-tight valve, which were analyzed by gas chromatography (Agilent Technologies 6850 Series II electron capture gas chromatograph) within a day of collection.
On days 7, 35, and 110, immediately following the final headspace sampling for N2O, the S = 0 and S = 20 press treatment soils that represented no-pulse (salinity of 0 g kg−1 for S = 0 and salinity of 20.0 g kg−1 for S = 20) and pulse (salinity of 25.6 g kg−1 for S = 0 and salinity of 3.5 g kg−1 for S = 20) conditions were frozen at −80 °C (samples without acetylene addition only). Nitrite reductase (nirS) and standard nitrous oxide reductase (nosZ) gene abundance (DNA), transcription products (cDNA), and expression (cDNA : DNA ratios) were measured on these soil samples. From each sample, DNA was extracted using the MO BIO PowerSoil DNA isolation kit and RNA was extracted following a modification of the extraction methods described by Mettel et al. (2010) and Kearns et al. (2016), which uses Q Sepharose chromatography and is optimized for soils with high humic acid content. After extraction, the RNA was reverse-transcribed to cDNA using Invitrogen's SuperScript III reverse transcriptase following the manufacturer's instructions. The concentration of DNA and cDNA was measured using Quant-iT PicoGreen and RiboGreen, respectively, following the manufacturer's instructions, and nucleic acids were normalized to 3 ng µL−1 prior to amplification via quantitative PCR (qPCR) on a Stratagene MX-3005P quantitative thermocycler using nirS primers from Braker et al. (1998) and nosZ primers from Henry et al. (2006) following previously described protocols (Bowen et al., 2011; Kearns et al., 2015). Standards for both genes were derived from purified PCR products, and standard curves had slopes > 0.99 and amplification efficiencies of ∼ 85 %.
Five mechanisms of pulsed physicochemical perturbation elicited a short-term response (observed within the first day following perturbation) that resulted in reduced rates of denitrification. Both conventionally farmed and organically farmed agricultural soils subjected to gradients of temperature, pH, toxicity (zinc), ionic strength (salinity), and moisture demonstrated reductions in rates of denitrification as the physicochemical variable deviated further from ambient conditions (Fig. 1). Rates of N2O production, in contrast, generally increased with increasing levels of perturbation (with the exception of zinc in the conventionally farmed soils; Fig. 1b) though N2O production rates exhibited a parabolic relationship with salinity (both soil types; Fig. 1a, f), zinc (for organically farmed soils; Fig. 1g), and soil moisture (both soils; Fig. 1e, j) such that, at the highest levels of salinity and zinc and the lowest soil moisture treatments, we observed declines in net N2O production. There were no observed declines in N2O production with increasing deviation away from ambient temperature (for conventionally farmed soils; Fig. 1d) or pH (for both soil types; Fig. 1c, h). N2O production declined with increasing zinc in conventionally farmed soils (Fig. 1b) and declined with lower temperatures in organically farmed soils (Fig. 1i). In all cases, the N2O : DNF ratio increased with increasing physicochemical perturbation, with N2O accounting for between less than 10 % (temperature) and nearly 100 % (soil moisture and salinity) of total denitrification at the highest level of disturbance (Fig. 1).
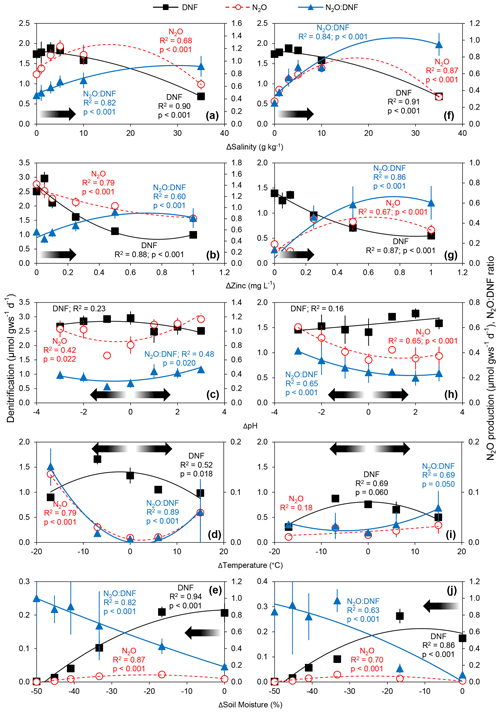
Figure 1Average (± standard deviation; n = 3) rates of total denitrification (DNF), nitrous oxide production (N2O), and N2O : DNF ratios in conventionally farmed (a–e) and organically farmed (f–j) agricultural soils as a function of changes in salinity (a, f), zinc concentration (b, g), pH (c, h), temperature (d, i), and soil moisture (e, j). Quadratic equations have been fit through all the data, and significant relationships are indicated. The arrows denote increasing deviation away from in situ conditions (i.e., perturbation).
In the experiment in which a salinity perturbation was imposed on estuarine sediments from three sites that had varying ambient salinities (0, 5, and 24 g kg−1), we found the highest rates of denitrification at the ambient salinity, with declining denitrification with deviation in salinity (Fig. 2). The lowest N2O production rates and N2O : DNF ratios were likewise observed at ambient salinities (Fig. 2). N2O production rates and N2O : DNF ratios increased with deviation in salinity away from ambient conditions. The highest N2O production rate and N2O : DNF ratios for freshwater sediments were observed at the highest salinity. In contrast, the highest N2O production and N2O : DNF ratio were found in the lowest salinity for the mesohaline sediment (Fig. 2).
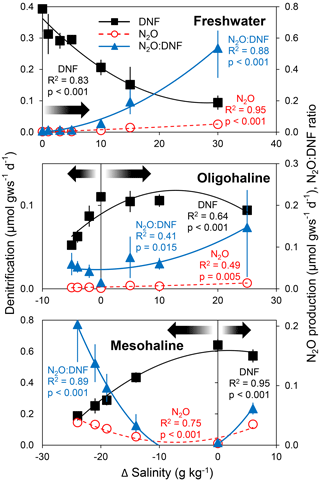
Figure 2Average (± standard deviation; n = 3) rates of total denitrification (DNF), nitrous oxide production (N2O), and N2O : DNF ratios in estuarine soils from tidal freshwater (in situ salinity = 0 g kg−1), oligohaline (5 g kg−1), and mesohaline (24 g kg−1) sites in the Scheldt Estuary as a function of changes in salinity. The arrows denote deviation away from in situ salinity.
We investigated the response to long-term changes (over ∼ months) in physicochemical conditions (press perturbation) to contrast to the pulsed perturbation responses described above. Soils from a tidal freshwater marsh (0 g kg−1 ambient salinity) in the Delaware River estuary were incubated under anaerobic conditions for 6 months after adjusting the salinity to 20 g kg−1 (the press treatment) along with a set of freshwater controls. Soils from these long-term incubations were subsampled throughout the 6-month period and assayed for rates of denitrification and N2O production when exposed to a range of salinities (approximately 0, 5, 10, 15, and 25 g kg−1). The pulse perturbation response to this range of salinities in both freshwater control and saltwater-amended treatments was similar immediately following the initiation of the experiment (on day 0) and remained consistent in the freshwater controls throughout the 6-month experiment (as indicated by N2O : DNF ratios; Fig. 3, black lines). In contrast, the response in soils subjected to the long-term press disturbance (the salinity-amended treatment) changed markedly over the 6-month period (Fig. 3, red lines). After 7 d at a salinity of 20 g kg−1, the press treatment soils did not respond to changes in salinity (N2O : DNF ratios were similar across pulse salinity treatments) though the consistently elevated N2O : DNF ratios (compared to the controls at a salinity of 0 g kg−1) indicated a perturbation response across all pulsed salinity levels (Fig. 3). At 1 month, the microbial community had adjusted to the higher salinity in the press treatment and exhibited a pulse perturbation response at lower salinities. This pattern was maintained for at least 6 months (Fig. 3).
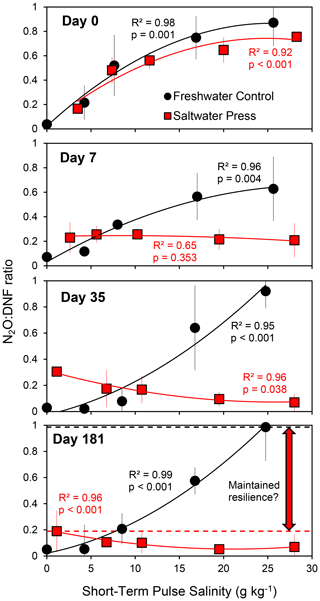
Figure 3Average N2O : DNF ratios (± standard deviation; n = 2) in a long-term (6-month) laboratory experiment in which tidal freshwater marsh soils were incubated under freshwater control (S = 0) or salinity-amended press conditions (S = 20). The soils were assayed for their short-term (pulse) salinity perturbation response on days 0 (immediately following salinity amendment), 7, 35, and 181 (results from sampling on days 14, 21, 49, 70, and 110 are available in Weston, 2024). The horizontal dashed lines in the day 181 panel indicate the maximum N2O : DNF ratios observed in the two treatments.
The denitrifier gene expression in the press–pulse experiment demonstrated that nirS expression was not correlated with either N2O production or N2O : DNF ratio in either the controls or the press treatment (p>0.05; Fig. 4). In contrast, the standard nosZ expression was negatively correlated with the N2O : DNF ratio in soils subjected to the press treatment (p = 0.026; Fig. 4). The relationship between nosZ expression and the N2O : DNF ratio in control soils was similar though the relationship was not significant (p = 0.10; Fig. 4). There was very little nosZ expression in any of the soils that experienced a pulsed change in salinity, i.e., either an increase in salinity for the controls or a decrease in salinity for the press treatment (Fig. 4).
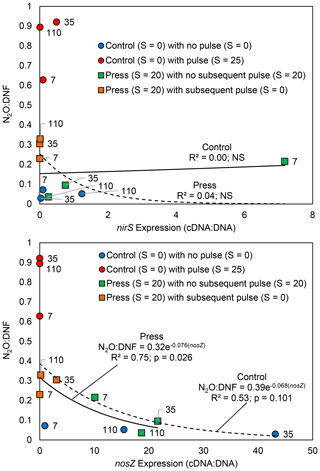
Figure 4The relationship between N2O : DNF ratios and nosZ enzyme expression from tidal freshwater marsh soils incubated under long-term freshwater control (S = 0) or saline press (S = 20) conditions and assayed on days 7, 35, and 110 with pulse or no-pulse salinity conditions. The timing of sampling (in days) is noted.
We found that changes in ionic strength (salinity), metal toxicity (zinc concentration), pH, temperature, and soil moisture all resulted in declines in denitrification, increased rates of N2O production (with decreased N2O production at higher levels of perturbation in some instances), and increased N2O : DNF ratios (Fig. 1). There was a relatively consistent short-term response in rates of denitrification and N2O production in response to a wide range of physicochemical perturbations (Fig. 1). We propose that changes in physicochemical conditions can induce a generalized short-term perturbation response from the soil denitrifying community, with higher N2O : DNF ratios and increased net N2O production and with reductions in N2O production at higher levels of perturbation for some parameters (Fig. 5a). Physicochemical perturbation is defined here as a shift from the ambient physical and/or chemical conditions experienced by a soil microbial community.
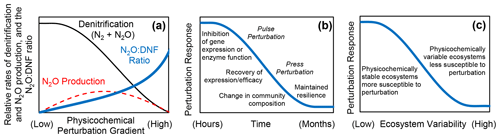
Figure 5Conceptual model based on the results of this study that shows the (a) relative rates of total denitrification (DNF), nitrous oxide (N2O) production, and N2O : DNF ratio in sediments and soils as a function of a physicochemical perturbation gradient; (b) response of the denitrifying microbial community to physicochemical perturbation over time; and (c) hypothesized relationship between the ecosystem physicochemical variability and the perturbation response.
There exists ample evidence that physical and chemical conditions influence denitrification and N2O production on the ecosystem scale. Temperature (Seitzinger, 1988; Larsen et al., 2011; Billings and Tiemann, 2014), salinity (Giblin et al., 2010; Teixeira et al., 2013), pH (Firestone et al., 1980; Weslien et al., 2009; Baggs et al., 2010), toxic heavy metals (Magalhaes et al., 2007; Ruyters et al., 2010), organic pesticides (Yang et al., 2023), and soil moisture (Teh et al., 2011; Brown et al., 2012; Wang et al., 2023) have all been posited as important in controlling denitrification and N2O emissions in soils and/or sediments. Our findings, except for the press–pulse salinity experiment, are not applicable for elucidating the long-term controls of these environmental factors on denitrification and N2O production. Rather, this study expands our understanding of the short-term response of the denitrifying community to alterations in the environment. Field measurements of N2O emissions have found pulses of N2O following physical disturbance of soils (Wang et al., 2005; Elder and Lal, 2008; Xu et al., 2015), soil thawing (Goodroad and Keeney, 1984; Chen et al., 2018), soil drying (Hou et al., 2012), clear-cutting, and hurricane disturbance (Steudler et al., 1991). Our findings suggest a framework (Fig. 5) for a better understanding of the response of the denitrifying community to physicochemical perturbation.
While some of the physicochemical variables investigated here may have long-lasting effects on the denitrifying community and N2O production, such as low soil pH (Liu et al., 2010, 2014), there are others that might not exert impacts on denitrification and N2O production indefinitely. For instance, high or low salinity does not inherently induce a perturbation response. Rather, the deviation from in situ conditions creates a disturbance to which the microbial denitrifying community responds and recovers from (Fig. 5b), indicating that the perturbation response is relative to the background environmental conditions experienced by the denitrifying community (Fig. 2). The press–pulse experiment further indicates that a microbial community can become adjusted to a new physicochemical condition such that a return to the original condition, given enough time (about a month in this case), amounts to additional perturbation (Fig. 3).
The press–pulse experiment (Fig. 3) further indicates that initial perturbation confers subsequent resilience to further perturbation in the denitrifying microbial community (Fig. 5c; Philippot et al., 2008; Griffiths and Philippot, 2013). The physicochemical pulse perturbation response consistently exceeds 60 % N2O production at higher salinities in the freshwater controls (Fig. 3). In contrast, N2O production did not exceed 20 % across all pulsed salinities after 6 months in the press treatments (Fig. 3). Similarly, the pulse perturbation response exceeded 50 % N2O production in tidal freshwater sediments that do not normally experience fluctuations in salinity and remained below 20 % N2O production in sediments from the oligohaline and mesohaline sites that experience daily (tidal) and seasonal fluctuations in salinity (Fig. 2). The observations from the press–pulse experiment (Fig. 3) and measurements along an estuarine salinity gradient (Fig. 2) together suggest that denitrifying microbial communities that experience changing physicochemical conditions may be more resilient to subsequent disturbance than an undisturbed denitrifying community as would be found in more physicochemically stable environments (Fig. 5c). Further research to determine the generality of this finding across ecosystem types and forms of physicochemical perturbation is warranted.
An aspect that requires consideration is the methodological approach we used in the current study. We utilized the acetylene block technique with the addition of substrates to soil or sediment slurries. The use of acetylene with the addition of substrates provides a measure of “potential” denitrification rather than in situ rates of denitrification or N2O production (Groffman et al., 1999, 2006). Acetylene is toxic to microbial nitrifiers (Hynes and Knowles, 1978) and potentially other members of the microbial community, which can inhibit coupled nitrification–denitrification and introduce other discrepancies that can alter nitrogen cycling (Groffman et al., 2006). The use of soil/sediment slurries further alters the biogeochemical zonation found in soils and sediments that is critical to creating the conditions in which redox-sensitive nitrogen cycling processes proceed (Froelich et al., 1979). However, the acetylene block method remains a powerful tool for evaluating controls on denitrification (Groffman et al., 2009), and our approach allowed us to feasibly explore a range of physicochemical variables at various levels and sites (i.e., Figs. 1 and 2) and over time (i.e., Fig. 3) in a controlled setting that would be difficult to undertake with other, less intrusive methods and with intact soils/sediments. Nevertheless, the generalizability of the perturbation response model to various physicochemical variables (Fig. 5) requires further investigation with less intrusive methodologies, such as substrate isotope labeling (Nielsen, 1992), that maintain microbial nitrogen cycling dynamics in relatively intact soils and sediments.
The physicochemical perturbation response we observed includes decreased rates of denitrification (Figs. 1 and 2), indicating inhibition of some portion of the microbial community responsible for the reduction of nitrogen oxides to dinitrogen gas. The changing N2O : DNF ratio, however, clearly indicates that the processes governing the production and consumption of N2O respond differently to the same physicochemical perturbation. Members of the microbial denitrifying community contain a large degree of modularity (Graf et al., 2014; Roco et al., 2017), possessing some or all of the genes that encode the enzymes necessary for catalyzing the nitrogen oxide reduction reactions. Changes in environmental conditions may promote modularity or may drive shifts in these communities, both of which could result in an alteration of the N2O : DNF ratio. For example, some denitrifiers lack the catalytic subunit gene for N2O reductase (nosZ) and produce N2O as the final metabolic product (Hedlund et al., 2011; Philippot et al., 2011). Complex microbial denitrifying communities in sediment/soil environments have been shown to include a variety of regulatory phenotypes that can result in the sequential transcription of genes in the denitrification pipeline, with transcription likely being triggered by the production of intermediates (Liu et al., 2019). Further, an atypical nosZ gene was identified that encodes a functional N2O reductase which, in many cases, is found in otherwise non-denitrifying organisms (Sanford et al., 2012), and the N2O uptake kinetics appear to differ between microbes with the standard and atypical nosZ genes (Yoon et al., 2016). Fungal denitrification, in which N2O is the terminal product, has likewise received increased attention following the finding that some fungi are able to denitrify (Maeda et al., 2015). Rates of denitrification and N2O emission from soils have been linked to the structure of the denitrifying community (Cavigelli and Robertson, 2001; Ruyters et al., 2010; Philippot et al., 2011), and deviations in physicochemical conditions that promote or inhibit modularity, influence transcription, and/or select for certain members of the denitrifying community may alter rates of denitrification and N2O emissions.
Community composition and relative gene abundance (Ruyters et al., 2010; Billings and Tiemann, 2014), transcription of the genes coding enzymes for N2O production and reduction (Magalhaes et al., 2011), and post-transcriptional interference with enzyme assembly and/or function (Liu et al., 2010, 2014) may all play a role in the observed N2O perturbation response. Our press–pulse experiment results indicate that the N2O salinity perturbation response is driven, at least in part, by the inhibition of the expression of the nitrous oxide reductase enzyme, thereby resulting in a higher proportion of N2O as the final product (Fig. 5b). We observed low nosZ expression in all pulse treatments and reduced expression in the press treatment that increased over time through the experiment (Fig. 4). We observed no correlation between nirS expression and either N2O production or N2O : DNF ratio (Fig. 4) across either press or pulse treatments. Further, nosZ expression was significantly correlated with the N2O : DNF ratio in the press treatment (Fig. 4), suggesting that inhibited expression of the nosZ enzyme responsible for the reduction of N2O to N2 was the likely mechanism for increased net N2O production in our press–pulse salinity experiment. In contrast, Liu et al. (2014) found that post-transcriptional interference of nosZ enzyme assembly in low-pH soils was the likely mechanism driving increased N2O production from soils. The mechanisms resulting in the N2O perturbation response may therefore differ between physicochemical variables, with likely combinations of both transcription and post-transcription enzyme inhibition together with more generalized impacts on the microbial community resulting in alternations to nitrogen cycling processes.
The pulse–press salinity experiment hints at the time required for the denitrifying community in estuarine sediments to adapt to salinity perturbation. We observed little nosZ expression in any of the pulse treatments with salinity perturbation whether from the control or press treatments (Fig. 4). In contrast, nosZ expression in the press treatment without additional salinity perturbation recovered somewhat after 1 week at higher salinity (Fig. 4). The expression of nosZ appeared to have fully recovered by 1 month, with no further change observed (Fig. 4). Likewise, N2O : DNF ratios in the press treatment demonstrated little response to pulsed changes in salinity after 1 week – the fraction of N2O produced is elevated (∼ 20 %) relative to the control without any pulse perturbation (∼ 5 %) but considerably lower than the control treatment at higher levels of salinity perturbation (which approach 100 % N2O; Fig. 3). At 1 month post-press, the denitrifying community adapted to the higher salinity and exhibited a pertubation response to reduced salinity, and the response was muted in comparison to the perturbation response of freshwater soils throughout the experiment (Fig. 3). There was little change in the salinity perturbation response in the press treatment after 1 month (Fig. 3). Both the N2O : DNF ratios (Fig. 3) and the nosZ expression results (Fig. 4) indicate that the microbial community recovered from the initial perturbation and adapted to the increased salinity level in the press treatment within 1 month, which suggests a generalizable model of perturbation recovery as gene expression changes with longer-term changes in the microbial community (Fig. 5b). Chen et al. (2018) found that pulsed N2O emissions following thawing of soils extended for 18 d, which suggests a similar time frame for a perturbation response to thawing. In contrast, Steudler et al. (1991) found higher N2O emissions for 7 months following a hurricane in subtropical forest soils. The transient perturbation response of the estuarine denitrifying community to salinity may be similar for some physicochemical variables, but it is unlikely to be the response to all changes in the environment. For instance, a higher N2O production response was observed in soils that had been subjected to low-pH conditions for over 20 years and was linked to post-transcription inhibition of nosZ (Liu et al., 2010, 2014), indicating that the microbial community does not recover from all perturbations and that the timing of any recovery might vary substantially.
Our research indicates that deviations from physicochemical conditions to which the microbial denitrifying community is adapted can induce a perturbation response that promotes increased net N2O production over a broad range of environmental variables (Fig. 1). We suggest a generalized conceptual model of the physicochemical perturbation response characterized by declining denitrification accompanied by increases in the N2O : DNF ratio, with increased net N2O production at moderate levels of disturbance (Fig. 5a). We show that the microbial denitrifying community may adapt to some physicochemical variables over time, such as salinity (Fig. 3), with moderation of the pulse perturbation response under press disturbance conditions (Fig. 5b). The pulse salinity perturbation response is characterized by an initial inhibition of nosZ enzyme expression (Fig. 4) that gives way to more effective N2O reduction that is likely driven by the recovery of gene expression and/or change in the denitrifier community composition (Fig. 5c). These findings indicate that an experimental press perturbation (Fig. 3) and in situ exposure to changes in physicochemical conditions such as salinity changes in oligohaline and mesohaline sediment (Fig. 2) confer resilience to subsequent perturbation (Fig. 5b; Philippot et al., 2008; Li et al., 2014). We therefore hypothesize that the perturbation response will be stronger in denitrifying communities from physicochemically stable ecosystems (i.e., ocean sediments and deep tropical soils) than from ecosystems that experience more physicochemical variability (i.e., temperate soils and tidal marshes). It is likely that this generalized perturbation response model (Fig. 5) does not describe the response of the denitrifying community to all changes in the physicochemical environment (such as low soil pH; Liu et al., 2014). However, this conceptual model may provide a useful framework for understanding (and potentially mitigating) N2O emissions from sediments and soils. Changing environmental conditions that perturb the denitrifying community likely promote hotspots and hot moments (Groffman et al., 2009) of N2O emissions and account for some of the variability in observed N2O emissions from soils and sediments.
The data that support the findings of this paper are available on the Environmental Data Initiative repository at https://doi.org/10.6073/pasta/49650b321b5c977b5e8baa92d991254b (Weston, 2024).
NBW, JLB, and SBJ: conceptualization. NBW, CT, and PJK: formal analysis. NBW, CT, PJK, WP, CH, CM, and PVC: investigation. NBW, JLB, and SBJ: methodology. NBW and SBJ: project administration. NBW and CT: visualization. NBW, CT, PJK, JLB, WP, and SBJ: writing (original draft). All authors: writing (review and editing). NBW, JLB, PVC, and SBJ: funding acquisition.
The contact author has declared that none of the authors has any competing interests.
Publisher’s note: Copernicus Publications remains neutral with regard to jurisdictional claims made in the text, published maps, institutional affiliations, or any other geographical representation in this paper. While Copernicus Publications makes every effort to include appropriate place names, the final responsibility lies with the authors.
We thank Margaret Garcia, Thomas Hoffman, Hillary Koch, Anniet Laverman, Hal Leaman, Justin Meschter, Jack Middelburg, Gerard Ondrey, Melanie Vile, and Justin Walsh for assisting with field collection and/or laboratory analyses.
This research has been supported by the Department of Geography and the Environment at Villanova University; the National Oceanic and Atmospheric Administration (grant no. NA960PO113); the National Science Foundation (grant nos. 9982133, 0620959, and 1350491); and the Georgia Sea Grant, University of Georgia (grant nos. NA06RG0029-R/WQ11 and NA06RG0029-R/WQ12A).
This paper was edited by Edouard Metzger and reviewed by Aubin Thibault de Chanvalon and one anonymous referee.
Baggs, E. M., Smales, C. L., and Bateman, E. J.: Changing pH shifts the microbial sourceas well as the magnitude of N2O emission from soil, Biol. Fertil. Soil., 46, 793–805, https://doi.org/10.1007/s00374-010-0484-6, 2010.
Bahram, M., Espenberg, M., Pärn, J., Lehtovirta-Morley, L., Anslan, S., Kasak, K., Koljalg, U., Liira, J., Maddison, M., Moora, M., Niinemets, Ü., Öpik, M., Pärtel, M., Soosaar, K., Zobel, M., Hildebrand, F., Tedersoo, L., and Mander, Ü.: Structure and function of the soil microbiome underlying N2O emissions from global wetlands, Nat. Commun., 13, 1430, https://doi.org/10.1038/s41467-022-29161-3, 2022.
Balderston, W. L., Sherr, B., and Payne, W. J.: Blockage by acetylene of nitrous-oxide reduction in Pseudomonas perfectomarinus, Appl. Environ. Microbiol., 31, 504–508, https://doi.org/10.1128/aem.31.4.504-508.1976, 1976.
Bao, Q. L., Ju, X. T., Gao, B., Qu, Z., Christie, P., and Lu, Y. H.: Response of nitrous oxide and corresponding bacteria to managements in an agricultural soil, Soil Sci. Soc. Am. J., 76, 130–141, https://doi.org/10.2136/sssaj2011.0152, 2012.
Barnard, R., Leadley, P. W., and Hungate, B. A.: Global change, nitrification, and denitrification: A review, Global Biogeochem. Cy., 19, GB1007, https://doi.org/10.1029/2004gb002282, 2005.
Beaulieu, J. J., Tank, J. L., Hamilton, S. K., Wollheim, W. M., Hall, R. O., Mulholland, P. J., Peterson, B. J., Ashkenas, L. R., Cooper, L. W., Dahm, C. N., Dodds, W. K., Grimm, N. B., Johnson, S. L., McDowell, W. H., Poole, G. C., Valett, H. M., Arango, C. P., Bernot, M. J., Burgin, A. J., Crenshaw, C. L., Helton, A. M., Johnson, L. T., O'Brien, J. M., Potter, J. D., Sheibley, R. W., Sobota, D. J., and Thomas, S. M.: Nitrous oxide emission from denitrification in stream and river networks, P. Natl. Acad. Sci. USA, 108, 214–219, https://doi.org/10.1073/pnas.1011464108, 2011.
Bender, E. A., Case, T. J., and Gilpin, M. E.: Perturbation experiments in community ecology – theory and practice, Ecology, 65, 1–13, https://doi.org/10.2307/1939452, 1984.
Betlach, M. R. and Tiedje, J. M.: Kinetic explanation for accumulation of nitrite, nitric-oxide, and nitrous-oxide during bacterial denitrification, Appl. Environ. Microbiol., 42, 1074–1084, 1981.
Billings, S. A. and Tiemann, L. K.: Warming-induced enhancement of soil N2O efflux linked to distinct response times of genes driving N2O production and consumption, Biogeochemistry, 119, 371–386, https://doi.org/10.1007/s10533-014-9973-2, 2014.
Bowen, J. L., Ward, B. B., Morrison, H. G., Hobbie, J. E., Valiela, I., Deegan, L. A., and Sogin, M. L.: Microbial community composition in sediments resists perturbation by nutrient enrichment, Isme J., 5, 1540–1548, https://doi.org/10.1038/ismej.2011.22, 2011.
Braker, G., Fesefeldt, A., and Witzel, K. P.: Development of PCR primer systems for amplification of nitrite reductase genes (nirK and nirS) to detect denitrifying bacteria in environmental samples, Appl. Environ. Microbiol., 64, 3769–3775, 1998.
Brown, J. R., Blankinship, J. C., Niboyet, A., van Groenigen, K. J., Dijkstra, P., Le Roux, X., Leadley, P. W., and Hungate, B. A.: Effects of multiple global change treatments on soil N2O fluxes, Biogeochemistry, 109, 85–100, https://doi.org/10.1007/s10533-011-9655-2, 2012.
Burgin, A. J. and Groffman, P. M.: Soil O2 controls denitrification rates and N2O yield in a riparian wetland, J. Geophys. Res.-Biogeo., 117, G01010, https://doi.org/10.1029/2011jg001799, 2012.
Butterbach-Bahl, K., Baggs, E. M., Dannenmann, M., Kiese, R., and Zechmeister-Boltenstern, S.: Nitrous oxide emissions from soils: how well do we understand the processes and their controls?, Philos. T. R. Soc. B, 368, 20130122, https://doi.org/10.1098/rstb.2013.0122, 2013.
Cavigelli, M. A. and Robertson, G. P.: Role of denitrifier diversity in rates of nitrous oxide consumption in a terrestrial ecosystem, Soil Biol. Biochem., 33, 297–310, https://doi.org/10.1016/s0038-0717(00)00141-3, 2001.
Chen, Z., Yang, S. Q., Zhang, A. P., Jing, X., Song, W. M., Mi, Z. R., Zhang, Q. W., Wang, W. Y., and Yang, Z. L.: Nitrous oxide emissions following seasonal freeze-thaw events from arable soils in Northeast China, J. Integr. Agr., 17, 231–246, https://doi.org/10.1016/s2095-3119(17)61738-6, 2018.
Conrad, R.: Soil microorganisms as controllers of atmospheric trace gases (H2, CO, CH4, OCS, N2O, and NO), Microbiol. Rev., 60, 609–640, https://doi.org/10.1128/mmbr.60.4.609-640.1996, 1996.
Davidson, E. A., Swank, W. T., and Perry, T. O.: Distinguishing between nitrification and denitrification as sources of gaseous nitrogen production in soil, Appl. Environ. Microbiol., 52, 1280–1286, https://doi.org/10.1128/aem.52.6.1280-1286.1986, 1986.
Denman, K. L., Brasseur, G., Chidthaisong, A., Ciais, P., Cox, P. M., Dickinson, R. E., Hauglustaine, D., Heinze, C., Holland, E., Jacob, D., Lohmann, U., Ramachandran, S., da Silva Dias, P. L., Wofsy, S. C., and Zhang, X.: Couplings between changes in the climate system and biogeochemistry, in: Climate Change 2007: The Physical Science Basis, Contribution of Working Group I to the Fourth Assessment Report of the Intergovernmental Panel on Climate Change, edited by: Solomon, S., Qin, D., Manning, M., Chen, Z., Marquis, M., Averyt, K. B., Tignor, M., and Miller, H. L., Cambridge University Press, Cambridge, United Kingdom and New York, NY, USA, 499–507, 2007.
Elder, J. W. and Lal, R.: Tillage effects on gaseous emissions from an intensively farmed organic soil in North Central Ohio, Soil Till. Res., 98, 45–55, https://doi.org/10.1016/j.still.2007.10.003, 2008.
Firestone, M. K., Firestone, R. B., and Tiedje, J. M.: Nitrous oxide from soil denitrification: Factors controlling its biological production, Science, 208, 749–751, https://doi.org/10.1126/science.208.4445.749, 1980.
Forster, P., Ramaswamy, V., Artaxo, P., Berntsen, T., Betts, R., Fahey, D. W., Haywood, J., Lean, J., Lowe, D. C., and Myhre, G.: Changes in atmospheric constituents and in radiative forcing, Climate Change 2007: The Physical Science Basis, Contribution of Working Group I to the 4th Assessment Report of the Intergovernmental Panel on Climate Change, edited by: Solomon, S., Qin, D., Manning, M., Chen, Z., Marquis, M., Averyt, K. B., Tignor, M., and Miller, H. L., Cambridge University Press, Cambridge, United Kingdom and New York, NY, USA, 2007.
Froelich, P. N., Klinkhammer, G. P., Bender, M. L., Luedtke, N. A., Heath, G. R., Cullen, D., Dauphin, P., Hammond, D., Hartman, B., and Maynard, V.: Early oxidation of organic-matter in pelagic sediments of the eastern Equatorial Atlantic – suboxic diagenesis, Geochim. Cosmochim. Ac., 43, 1075–1090, https://doi.org/10.1016/0016-7037(79)90095-4, 1979.
Galloway, J. N., Dentener, F. J., Capone, D. G., Boyer, E. W., Howarth, R. W., Seitzinger, S. P., Asner, G. P., Cleveland, C. C., Green, P. A., Holland, E. A., Karl, D. M., Michaels, A. F., Porter, J. H., Townsend, A. R., and Vorosmarty, C. J.: Nitrogen cycles: Past, present, and future, Biogeochemistry, 70, 153–226, https://doi.org/10.1007/s10533-004-0370-0, 2004.
Gerringa, L. J. A., Hummel, H., and Moerdijk-Poortvliet, T. C. W.: Relations between free copper and salinity, dissolved and particulate organic carbon in the Oosterschelde and Westerschelde, Netherlands, J. Sea Res., 40, 193–203, https://doi.org/10.1016/S1385-1101(98)00021-5, 1998.
Giblin, A. E., Weston, N. B., Banta, G. T., Tucker, J., and Hopkinson, C. S.: The effects of salinity on nitrogen losses from an oligohaline estuarine sediment, Estuar. Coast., 33, 1054–1068, https://doi.org/10.1007/s12237-010-9280-7, 2010.
Goodroad, L. L. and Keeney, D. R.: Nitrous oxide emissions from soils during thawing, Can. J. Soil Sci., 64, 187–194, https://doi.org/10.4141/cjss84-020, 1984.
Grabb, K. C., Buchwald, C., Hansel, C. M., and Wankel, S. D.: A dual nitrite isotopic investigation of chemodenitrification by mineral-associated Fe(II) and its production of nitrous oxide, Geochim. Cosmochim. Ac., 196, 388–402, https://doi.org/10.1016/j.gca.2016.10.026, 2017.
Graf, D. R. H., Jones, C. M., and Hallin, S.: Intergenomic comparisons highlight modularity of the denitrification pathway and underpin the importance of community structure for N2O emissions, Plos One, 9, e114118, https://doi.org/10.1371/journal.pone.0114118, 2014.
Griffiths, B. S. and Philippot, L.: Insights into the resistance and resilience of the soil microbial community, Fems Microbiol. Rev., 37, 112–129, https://doi.org/10.1111/j.1574-6976.2012.00343.x, 2013.
Groffman, P. M., Holland, E., Myrold, D. D., Robertson, G. P., and Zou, X.: Denitrification, in: Standard Soil Methods for Long Term Ecological Research, edited by: Robertson, G. P., Bledsoe, C. S., Coleman, D. C., and Sollins, P., Oxford University Press, New York, NY, 272–288, 1999.
Groffman, P. M., Altabet, M. A., Bohlke, J. K., Butterbach-Bahl, K., David, M. B., Firestone, M. K., Giblin, A. E., Kana, T. M., Nielsen, L. P., and Voytek, M. A.: Methods for measuring denitrification: Diverse approaches to a difficult problem, Ecol. Appl., 16, 2091–2122, https://doi.org/10.1890/1051-0761(2006)016[2091:mfmdda]2.0.co;2, 2006.
Groffman, P. M., Butterbach-Bahl, K., Fulweiler, R. W., Gold, A. J., Morse, J. L., Stander, E. K., Tague, C., Tonitto, C., and Vidon, P.: Challenges to incorporating spatially and temporally explicit phenomena (hotspots and hot moments) in denitrification models, Biogeochemistry, 93, 49–77, https://doi.org/10.1007/s10533-008-9277-5, 2009.
Harrison-Kirk, T., Beare, M. H., Meenken, E. D., and Condron, L. M.: Soil organic matter and texture affect responses to dry/wet cycles: Effects on carbon dioxide and nitrous oxide emissions, Soil Biol. Biochem., 57, 43–55, https://doi.org/10.1016/j.soilbio.2012.10.008, 2013.
Hedlund, B. P., McDonald, A. I., Lam, J., Dodsworth, J. A., Brown, J. R., and Hungate, B. A.: Potential role of Thermus thermophilus and T. oshimai in high rates of nitrous oxide (N2O) production in ∼ 80 °C hot springs in the US Great Basin, Geobiology, 9, 471–480, https://doi.org/10.1111/j.1472-4669.2011.00295.x, 2011.
Henault, C., Grossel, A., Mary, B., Roussel, M., and Leonard, J.: Nitrous oxide emission by agricultural soils: A review of spatial and temporal variability for mitigation, Pedosphere, 22, 426–433, 2012.
Henry, S., Bru, D., Stres, B., Hallet, S., and Philippot, L.: Quantitative detection of the nosZ gene, encoding nitrous oxide reductase, and comparison of the abundances of 16S rRNA, narG, nirK, and nosZ genes in soils, Appl. Environ. Microbiol., 72, 5181–5189, https://doi.org/10.1128/aem.00231-06, 2006.
Hou, H. J., Peng, S. Z., Xu, J. Z., Yang, S. H., and Mao, Z.: Seasonal variations of CH4 and N2O emissions in response to water management of paddy fields located in Southeast China, Chemosphere, 89, 884–892, https://doi.org/10.1016/j.chemosphere.2012.04.066, 2012.
Hu, H. W., Chen, D., and He, J. Z.: Microbial regulation of terrestrial nitrous oxide formation: Understanding the biological pathways for prediction of emission rates, Fems Microbiol. Rev., 39, 729–749, https://doi.org/10.1093/femsre/fuv021, 2015.
Huang, X. D., Grace, P., Mengersen, K., and Weier, K.: Spatio-temporal variation in soil derived nitrous oxide emissions under sugarcane, Sci. Total Environ., 409, 4572–4578, https://doi.org/10.1016/j.scitotenv.2011.07.044, 2011.
Hynes, R. K. and Knowles, R.: Inhibition by acetylene of ammonia oxidation in Nitrosomonas-europaea, Fems Microbiol. Lett., 4, 319–321, https://doi.org/10.1111/j.1574-6968.1978.tb02889.x, 1978.
Kearns, P. J., Angell, J. H., Feinman, S. G., and Bowen, J. L.: Long-term nutrient addition differentially alters community composition and diversity of genes that control nitrous oxide flux from salt marsh sediments, Estuar. Coast. Shelf Sci., 154, 39–47, https://doi.org/10.1016/j.ecss.2014.12.014, 2015.
Kearns, P. J., Angell, J. H., Howard, E. M., Deegan, L. A., Stanley, R. H. R., and Bowen, J. L.: Nutrient enrichment induces dormancy and decreases diversity of active bacteria in salt marsh sediments, Nat. Commun., 7, 12881, https://doi.org/10.1038/ncomms12881, 2016.
Knowles, R.: Denitrification, Microbiol. Rev., 46, 43–70, 1982.
Larsen, K. S., Andresen, L. C., Beier, C., Jonasson, S., Albert, K. R., Ambus, P., Arndal, M. F., Carter, M. S., Christensen, S., Holmstrup, M., Ibrom, A., Kongstad, J., van der Linden, L., Maraldo, K., Michelsen, A., Mikkelsen, T. N., Pilegaard, K., Prieme, A., Ro-Poulsen, H., Schmidt, I. K., Selsted, M. B., and Stevnbak, K.: Reduced N cycling in response to elevated CO2, warming, and drought in a Danish heathland: Synthesizing results of the CLIMAITE project after two years of treatments, Glob. Change Biol., 17, 1884–1899, https://doi.org/10.1111/j.1365-2486.2010.02351.x, 2011.
Li, Y., Fu, X. Q., Liu, X. L., Shen, J. L., Luo, Q., Xiao, R. L., Li, Y. Y., Tong, C. L., and Wu, J. S.: Spatial variability and distribution of N2O emissions from a tea field during the dry season in subtropical central China, Geoderma, 193, 1–12, https://doi.org/10.1016/j.geoderma.2012.10.008, 2013.
Liu, B. B., Morkved, P. T., Frostegard, A., and Bakken, L. R.: Denitrification gene pools, transcription and kinetics of NO, N2O and N2 production as affected by soil pH, Fems Microbiol. Ecol., 72, 407–417, https://doi.org/10.1111/j.1574-6941.2010.00856.x, 2010.
Liu, B. B., Frostegård, Å., and Bakken, L. R.: Impaired reduction of N2O to N2 in acid soils is due to a posttranscriptional interference with the expression of nosZ, Mbio, 5, 3, https://doi.org/10.1128/mBio.01383-14, 2014.
Liu, B. B., Zhang, X., Bakken, L. R., Snipen, L., and Frostegård, Å.: Rapid succession of actively transcribing denitrifier populations in agricultural soil during an anoxic spell, Front. Microbiol., 9, 3208, https://doi.org/10.3389/fmicb.2018.03208, 2019.
Maeda, K., Spor, A., Edel-Hermann, V., Heraud, C., Breuil, M. C., Bizouard, F., Toyoda, S., Yoshida, N., Steinberg, C., and Philippot, L.: N2O production, a widespread trait in fungi, Sci. Rep., 5, 9697, https://doi.org/10.1038/srep09697, 2015.
Magalhaes, C., Costa, J., Teixeira, C., and Bordalo, A. A.: Impact of trace metals on denitrification in estuarine sediments of the Douro River estuary, Portugal, Mar. Chem., 107, 332–341, https://doi.org/10.1016/j.marchem.2007.02.005, 2007.
Magalhaes, C. M., Machado, A., Matos, P., and Bordalo, A. A.: Impact of copper on the diversity, abundance and transcription of nitrite and nitrous oxide reductase genes in an urban European estuary, Fems Microbiol. Ecol., 77, 274–284, https://doi.org/10.1111/j.1574-6941.2011.01107.x, 2011.
Mathieu, O., Hénault, C., Lévêque, J., Baujard, E., Milloux, M. J., and Andreux, F.: Quantifying the contribution of nitrification and denitrification to the nitrous oxide flux using 15N tracers, Environ. Pollut., 144, 933–940, https://doi.org/10.1016/j.envpol.2006.02.005, 2006.
McClain, M. E., Boyer, E. W., Dent, C. L., Gergel, S. E., Grimm, N. B., Groffman, P. M., Hart, S. C., Harvey, J. W., Johnston, C. A., Mayorga, E., McDowell, W. H., and Pinay, G.: Biogeochemical hot spots and hot moments at the interface of terrestrial and aquatic ecosystems, Ecosystems, 6, 301–312, https://doi.org/10.1007/s10021-003-0161-9, 2003.
Mettel, C., Kim, Y., Shrestha, P. M., and Liesack, W.: Extraction of mRNA from Soil, Appl. Environ. Microbiol., 76, 5995–6000, https://doi.org/10.1128/aem.03047-09, 2010.
Nielsen, L. P.: Denitrification in sediment determined from nitrogen isotope pairing, FEMS Microbiol. Ecol., 86, 357–362, https://doi.org/10.1111/j.1574-6968.1992.tb04828.x 1992.
Nixon, S. W.: Eutrophication and the macroscope, Hydrobiologia, 629, 5–19, https://doi.org/10.1007/s10750-009-9759-z, 2009.
Payne, W. J.: Reduction of nitrogenous oxides by microorganisms, Bacteriol. Rev., 37, 409–452, https://doi.org/10.1128/mmbr.37.4.409-452.1973, 1973.
Philippot, L., Andert, J., Jones, C. M., Bru, D., and Hallin, S.: Importance of denitrifiers lacking the genes encoding the nitrous oxide reductase for N2O emissions from soil, Glob. Change Biol., 17, 1497–1504, https://doi.org/10.1111/j.1365-2486.2010.02334.x, 2011.
Philippot, L., Cregut, M., Chèneby, D., Bressan, M., Dequiet, S., Martin-Laurent, F., Ranjard, L., and Lemanceau, P.: Effect of primary mild stresses on resilience and resistance of the nitrate reducer community to a subsequent severe stress, Fems Microbiol. Lett., 285, 51–57, https://doi.org/10.1111/j.1574-6968.2008.01210.x, 2008.
Porubsky, W. P., Weston, N. B., and Joye, S. B.: Benthic metabolism and the fate of dissolved inorganic nitrogen in intertidal sediments, Estuar. Coast. Shelf Sci., 83, 392–402, https://doi.org/10.1016/j.ecss.2009.04.012, 2009.
Raut, N., Dorsch, P., Sitaula, B. K., and Bakken, L. R.: Soil acidification by intensified crop production in South Asia results in higher N2O (N2+N2O) product ratios of denitrification, Soil Biol. Biochem., 55, 104–112, https://doi.org/10.1016/j.soilbio.2012.06.011, 2012.
Ravishankara, A. R., Daniel, J. S., and Portmann, R. W.: Nitrous oxide (N2O): The dominant ozone-depleting substance emitted in the 21st Century, Science, 326, 123–125, https://doi.org/10.1126/science.1176985, 2009.
Robinson, T. C., Latta, D. E., Notini, L., Schilling, K. E., and Scherer, M. M.: Abiotic reduction of nitrite by Fe(II): a comparison of rates and N2O production, Environ. Sci., 23, 1531–1541, 2021.
Roco, C. A., Bergaust, L. L., Bakken, L. R., Yavitt, J. B., and Shapleigh, J. P.: Modularity of nitrogen-oxide reducing soil bacteria: Linking phenotype to genotype, Environ. Microbiol., 19, 2507–2519, https://doi.org/10.1111/1462-2920.13250, 2017.
Rosamond, M. S., Thuss, S. J., and Schiff, S. L.: Dependence of riverine nitrous oxide emissions on dissolved oxygen levels, Nat. Geosci., 5, 715–718, https://doi.org/10.1038/ngeo1556, 2012.
Ruyters, S., Mertens, J., T'Seyen, I., Springael, D., and Smolders, E.: Dynamics of the nitrous oxide reducing community during adaptation to Zn stress in soil, Soil Biol. Biochem., 42, 1581–1587, https://doi.org/10.1016/j.soilbio.2010.05.036, 2010.
Sanford, R. A., Wagner, D. D., Wu, Q. Z., Chee-Sanford, J. C., Thomas, S. H., Cruz-Garcia, C., Rodriguez, G., Massol-Deya, A., Krishnani, K. K., Ritalahti, K. M., Nissen, S., Konstantinidis, K. T., and Loffler, F. E.: Unexpected nondenitrifier nitrous oxide reductase gene diversity and abundance in soils, P. Natl. Acad. Sci. USA, 109, 19709–19714, https://doi.org/10.1073/pnas.1211238109, 2012.
Seitzinger, S. P.: Denitrification in fresh-water and coastal marine ecosystems – ecological and geochemical significance, Limnol. Oceanogr., 33, 702–724, 1988.
Steudler, P. A., Melillo, J. M., Bowden, R. D., Castro, M. S., and Lugo, A. E.: The effects of natural and human disturbances on soil nitrogen dynamics and trace gas fluxes in a Puerto Rican wet forest, Biotropica, 23, 356–363, https://doi.org/10.2307/2388252, 1991.
Teh, Y. A., Silver, W. L., Sonnentag, O., Detto, M., Kelly, M., and Baldocchi, D. D.: Large greenhouse gas emissions from a temperate peatland pasture, Ecosystems, 14, 311–325, https://doi.org/10.1007/s10021-011-9411-4, 2011.
Teixeira, C., Magalhaes, C., Joye, S. B., and Bordalo, A. A.: The role of salinity in shaping dissolved inorganic nitrogen and N2O dynamics in estuarine sediment-water interface, Mar. Pollut. Bull., 66, 225–229, https://doi.org/10.1016/j.marpolbul.2012.11.004, 2013.
Tian, H. Q., Xu, R. T., Canadell, J. G., Thompson, R. L., Winiwarter, W., Suntharalingam, P., Davidson, E. A., Ciais, P., Jackson, R. B., Janssens-Maenhout, G., Prather, M. J., Regnier, P., Pan, N. Q., Pan, S. F., Peters, G. P., Shi, H., Tubiello, F. N., Zaehle, S., Zhou, F., Arneth, A., Battaglia, G., Berthet, S., Bopp, L., Bouwman, A. F., Buitenhuis, E. T., Chang, J. F., Chipperfield, M. P., Dangal, S. R. S., Dlugokencky, E., Elkins, J. W., Eyre, B. D., Fu, B. J., Hall, B., Ito, A., Joos, F., Krummel, P. B., Landolfi, A., Laruelle, G. G., Lauerwald, R., Li, W., Lienert, S., Maavara, T., MacLeod, M., Millet, D. B., Olin, S., Patra, P. K., Prinn, R. G., Raymond, P. A., Ruiz, D. J., van der Werf, G. R., Vuichard, N., Wang, J. J., Weiss, R. F., Wells, K. C., Wilson, C., Yang, J., and Yao, Y. Z.: A comprehensive quantification of global nitrous oxide sources and sinks, Nature, 586, 248–256, https://doi.org/10.1038/s41586-020-2780-0, 2020.
van Damme, S., Struyf, E., Maris, T., Ysebaert, T., Dehairs, F., Tackx, M., Heip, C., and Meire, P.: Spatial and temporal patterns of water quality along the estuarine salinity gradient of the Scheldt estuary (Belgium and The Netherlands): Results of an integrated monitoring approach, Hydrobiologia, 540, 29–45, https://doi.org/10.1007/s10750-004-7102-2, 2005.
van den Heuvel, R. N., Bakker, S. E., Jetten, M. S. M., and Hefting, M. M.: Decreased N2O reduction by low soil pH causes high N2O emissions in a riparian ecosystem, Geobiology, 9, 294–300, https://doi.org/10.1111/j.1472-4669.2011.00276.x, 2011.
Vilain, G., Garnier, J., Decuq, C., and Lugnot, M.: Nitrous oxide production from soil experiments: denitrification prevails over nitrification, Nutr. Cycl. Agroecosyst., 98, 169–186, https://doi.org/10.1007/s10705-014-9604-2, 2014.
Vitousek, P. M. and Howarth, R. W.: Nitrogen limitation on land and in the sea – how can it occur, Biogeochemistry, 13, 87–115, 1991.
Wang, H., Yan, Z. F., Ju, X. T., Song, X. T., Zhang, J. B., Li, S. L., and Xia, Z. B.: Quantifying nitrous oxide production rates from nitrification and denitrification under various moisture conditions in agricultural soils: Laboratory study and literature synthesis, Front. Microbiol., 13, 1110151, https://doi.org/10.3389/fmicb.2022.1110151, 2023.
Wang, L. F., Cai, Z. C., Yang, L. F., and Meng, L.: Effects of disturbance and glucose addition on nitrous oxide and carbon dioxide emissions from a paddy soil, Soil Till. Res., 82, 185–194, https://doi.org/10.1016/j.still.2004.06.001, 2005.
Weitzman, J. N., Groffman, P. M., Adler, P. R., Dell, C. J., Johnson, F. E., Lerch, R. N., and Strickland, T. C.: Drivers of hot spots and hot moments of denitrification in agricultural systems, J. Geophys. Res.-Biogeo., 126, e2020JG006234, https://doi.org/10.1029/2020jg006234, 2021.
Weslien, P., Klemedtsson, A. K., Borjesson, G., and Klemedtsson, L.: Strong pH influence on N2O and CH4 fluxes from forested organic soils, Europ. J. Soil Sci., 60, 311–320, https://doi.org/10.1111/j.1365-2389.2009.01123.x, 2009.
Weston, N.: Laboratory measurements of nitrous oxide production rates in agricultural soils from Lancaster, PA, estuarine sediments from the Scheldt Estuary Belgium/Netherlands, and estuarine soils from the Delaware River NJ under gradients of physicochemical perturbation ver 1, Environmental Data Initiative [data set], https://doi.org/10.6073/pasta/49650b321b5c977b5e8baa92d991254b, 2024.
Wuebbles, D. J.: Nitrous oxide: No laughing matter, Science, 326, 56–57, https://doi.org/10.1126/science.1179571, 2009.
Xu, J. Z., Wei, Q., Yang, S. H., Gao, X. L., and Lv, Y. P.: Influence of watering methods on the short-term pulse emissions of nitrous oxide from disturbed horticultural soil, Commun. Soil Sci. Plant Anal., 46, 2688–2706, https://doi.org/10.1080/00103624.2015.1089269, 2015.
Yang, Z., Deng, Y., Zhong, L., Xiao, R., and Su, X. X.: Responses of soil bacterial and fungal denitrification and associated N2O emissions to organochloride pesticide, Sci. Total Environ., 905, 167321, https://doi.org/10.1016/j.scitotenv.2023.167321, 2023.
Yoon, S., Nissen, S., Park, D., Sanford, R. A., and Löffler, F. E.: Nitrous oxide reduction kinetics distinguish bacteria harboring Clade I Nosz from those harboring Clade II NosZ, Appl. Environ. Microbiol., 82, 3793–3800, https://doi.org/10.1128/aem.00409-16, 2016.
Yoshinari, T. and Knowles, R.: Acetylene inhibition of nitrous oxide reduction by denitrifying bacteria, Biochem. Biophys. Res. Commun., 69, 705–710, https://doi.org/10.1016/0006-291x(76)90932-3, 1976.