the Creative Commons Attribution 4.0 License.
the Creative Commons Attribution 4.0 License.
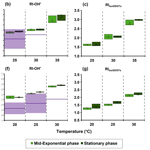
Controls on the composition of hydroxylated isoprenoidal glycerol dialkyl glycerol tetraethers (isoGDGTs) in cultivated ammonia-oxidizing Thaumarchaeota
Laura Villanueva
Nicole J. Bale
Pierre Offre
Gert-Jan Reichart
Stefan Schouten
Membrane lipids of ammonia-oxidizing Thaumarchaeota, in particular isoprenoidal glycerol dialkyl glycerol tetraethers (isoGDGTs) and hydroxylated isoGDGTs (OH-isoGDGTs), have been used as biomarkers and as proxies in various environments. Controlled growth experiments have been used to investigate the factors that influence the composition of these lipids, in particular on how these factors affect the TEX86 temperature proxy, which is based on the degree of cyclization of isoGDGTs. Recently, the ring index of OH-isoGDGTs (RI-OH′), based on cyclization patterns of OH-isoGDGTs, and the abundance of OH-isoGDGTs relative to summed abundances of OH-isoGDGTs and regular isoGDGTs (% OH) have emerged as promising temperature proxies. Here, we examined the impact of growth temperature and growth phase on the distribution of OH-isoGDGTs and their associated proxies using cultures of two thaumarchaeotal strains. Analysis of core lipids and headgroup compositions of isoGDGTs and OH-isoGDGTs showed no consistent differences between the mid-exponential and stationary phases for both strains. Nitrosopumilus adriaticus NF5 shows a substantially higher relative abundance of OH-isoGDGTs (∼ 49 %) compared to Nitrosopumilus piranensis D3C (∼ 5 %) and also relative to observations reported for core lipids in the marine environment (< 17 %), indicating large variations in % OH values even among closely related species. Unlike in the marine environment, the % OH did not decrease with increasing temperatures in either of the strains, possibly reflecting a threshold below 15 °C for this response in the natural environment. The RI-OH′ increases with increasing temperature in cultures of both strains, similar to the ring index of regular isoGDGTs. The relative abundances of the headgroups varied between strains and did not respond to changes in temperature or growth phase. The % OH and RI-OH′ calculated from intact polar lipids with different headgroups revealed large differences between the distinct intact polar lipids, similar to that previously observed for regular isoGDGTs. Together, our findings suggest that growth temperature has a pronounced effect on the degree of cyclization in isoGDGTs and OH-isoGDGTs, in contrast to the relative abundance of OH-isoGDGTs, which mainly exhibits interspecies variability.
- Article
(2278 KB) - Full-text XML
-
Supplement
(1013 KB) - BibTeX
- EndNote
Isoprenoidal GDGTs with zero to four cyclopentane moieties, as well as crenarchaeol and its isomer (cren′), which contain a cyclohexane moiety in addition to four cyclopentane moieties, are the main membrane lipids synthesized by ammonia-oxidizing archaea of Thaumarchaeota (Sinninghe Damsté et al., 2002; Zeng et al., 2019), now referred to as Nitrososphaeria (Genome Taxonomy Database; Rinke et al., 2021). They are widely used as biomarkers and in various environmental proxies (e.g., Blaga et al., 2009; Weijers et al., 2011; Zhang et al., 2011; Sinninghe Damsté et al., 2012; Taylor et al., 2013; Inglis et al., 2015; O'Brien et al., 2017). These include the tetraether index of 86 carbons (TEX86; Schouten et al., 2002) and the ring index of isoGDGTs based on the degree of cyclization of the isoGDGTs (RIisoGDGTs; Pearson et al., 2004; Zhang et al., 2016), which are indicative of temperature conditions at which these organisms grow.
Hydroxylated isoGDGTs (OH-isoGDGTs) are lipids with one or more hydroxy groups in the biphytanyl chains and are also widespread in the marine environment (Liu et al., 2012b; Huguet et al., 2013; Varma et al., 2024a). OH-isoGDGTs with zero to two cyclopentane moieties are typically ubiquitously found in the open ocean, whereas those with three and four cyclopentane moieties, as well as those with a cyclohexane moiety and four cyclopentane moieties (OH-crenarchaeol), are mainly found in cold seep sediments, the Black Sea water column and thaumarchaeotal cultures (Zhang et al., 2023; Elling et al., 2017). OH-isoGDGTs with zero to two cyclopentane moieties are thought to be produced primarily by Thaumarchaeota in the marine environment (Elling et al., 2017; Sinninghe Damsté et al., 2012; Liu et al., 2012b, a) and are utilized in paleothermometers, such as the abundance of OH-isoGDGTs relative to summed abundances of OH-isoGDGTs and regular isoGDGTs (% OH; Huguet et al., 2013), RI-OH′ and RI-OH (Lü et al., 2015), and recently (Varma et al., 2024a). Although OH-isoGDGT-based proxies have demonstrated promise in paleoenvironmental reconstructions (e.g., Davtian et al., 2019; Morcillo-Montalbá et al., 2021; Varma et al., 2024a; Fietz et al., 2020; Davtian and Bard, 2023; Liu et al., 2022; Sinninghe Damsté et al., 2022), the physiological role of OH-isoGDGTs is not well understood, which limits the interpretation of the OH-isoGDGT-based proxies. Biophysical modeling suggests that hydroxylation enhances the fluidity of the membrane and thereby provides a mechanism for archaeal cells to adapt to lower temperatures (Huguet et al., 2017).
The isoGDGT- and OH-isoGDGT-based temperature proxies are mostly established using core-top and suspended particulate matter studies (e.g., Kim et al., 2010; Basse et al., 2014; Xie et al., 2014; Lü et al., 2015, 2019; Hurley et al., 2018; Fietz et al., 2020; Varma et al., 2024a). While mesocosm studies provided insights into the impact of temperature and salinity on isoGDGT lipid distribution and archaeal communities (Schouten et al., 2007; Wuchter et al., 2004), studies of cultivated archaea in laboratory settings allowed studying the impact of specific parameters on lipid compositions of these archaeal cultures. For example, it was shown that the degree of cyclization, as indicated by RIisoGDGTs, increases with higher growth temperatures in cultivated Thaumarchaeota (Qin et al., 2015; Elling et al., 2015). However, the relationship between TEX86 and temperature in these cultures is complex and differs from that observed in the natural environment (Qin et al., 2015; Elling et al., 2015, 2017; Bale et al., 2019). Both RIisoGDGTs and TEX86 exhibit distinct species-specific relationships with growth temperature among different strains of ammonia-oxidizing archaea (Elling et al., 2017, 2015; Bale et al., 2019; Qin et al., 2015; Pitcher et al., 2011; Sinninghe Damsté et al., 2012). Therefore, besides environmental and ecological factors, variations in archaeal community composition across biogeographic regions likely also play a role in shaping the relationship between isoGDGT distribution and temperature (e.g., Trommer et al., 2009; Polik et al., 2018; Besseling et al., 2019; Sollai et al., 2019). The availability of oxygen, rate of ammonia oxidation and culture growth phase are also known to impact the composition of the isoGDGTs in archaeal cells and thereby TEX86 values in culture experiments (Qin et al., 2015; Hurley et al., 2016; Elling et al., 2014, 2015). However, pH and salinity have only a minor effect on TEX86 (Elling et al., 2015; Wuchter et al., 2004). The polar headgroups attached to the core GDGTs vary among different archaeal species, core isoGDGT types, growth phases and temperatures (Bale et al., 2019; Elling et al., 2014, 2015, 2017), suggesting complex archaeal membrane adaptation.
In contrast to regular isoGDGTs, OH-isoGDGTs have been studied less extensively in cultured organisms, but the few existing studies do show major differences between species. Among cultured Thaumarchaeota, OH-isoGDGTs were mainly detected within the order Nitrosopumilales (previously reported as Group I.1a Thaumarchaeota and SAGMCG-1; see Elling et al., 2017) from soil and marine environments, and they were also detected in one culture of Nitrososphaerales (Bale et al., 2019). However, OH-isoGDGTs are not detected in all species of Nitrosopumilales, for instance, in the thermophilic Thaumarchaeota Nitrosotenuis uzonensis (Bale et al., 2019). Similar to regular isoGDGTs, OH-isoGDGTs also exhibit variations in IPL composition across thaumarchaeotal strains (Elling et al., 2017, 2015). While OH-isoGDGT core lipids are utilized as temperature proxies in the marine environment (Huguet et al., 2013), the abundance of OH-isoGDGTs from both core lipids and IPLs in cultures does not seem to be related to the cultivation temperature of these archaea (Elling et al., 2017, 2015). Nevertheless, only a small number of archaea have so far been examined for OH-isoGDGTs, and with the development of new proxies based on OH-isoGDGTs (see Varma et al., 2024a), there is a need for controlled growth studies on the role of OH-isoGDGTs in membranes of archaea.
To further investigate the factors controlling the relative abundance and composition of OH-isoGDGTs, we examined the intact polar lipids of isoGDGTs and OH-isoGDGTs in two strains of Thaumarchaeota grown at different temperatures and harvested at different growth phases. We aim to throw light on the impact of temperature, growth phase and species on the composition of OH-isoGDGTs and the proxies based on them.
2.1 Cultivation of ammonia-oxidizing archaea
Enrichment cultures of the Nitrosopumilus piranensis D3C strain and the Nitrosopumilus adriaticus NF5 strain were grown in 2 L Schott bottles in Synthetic Crenarchaeota Medium, supplemented with bovine liver catalase (Sigma-Aldrich, Germany) at 5 U mL−1 final concentration (Martens-Habbena et al., 2009; Bayer et al., 2019a, b). The growth phase of the cultures was monitored by measuring nitrite production using Griess reagent (Griess, 1879) prepared with 1 % () sulfanilamide and 0.1 % () N-naphtylethylendiamindihydrochloride in 5 % hydrochloric acid, as well as absorbance spectroscopy (Bayer et al., 2016) using a spectrophotometer (Molecular Devices SpectraMax M2) at absorbance 545 nm. Samples for cell counts (0.5 mL) were fixed with glutaraldehyde (0.5 % ) for 15 min, flash-frozen with liquid nitrogen and subsequently stored at −80 °C until further use. Subsequently, the samples were diluted in TE buffer and stained with SYBR Green I (final concentration 5 × 10−4 of the commercial stock; Life Technologies, the Netherlands) prior to flow cytometry analysis to determine cell abundances by using a BD FACSCelesta flow cytometer.
2.2 Temperature experiment setups
The N. adriaticus NF5 strain was grown at 20, 25 and 30 °C, and the N. piranensis D3C strain was grown at 25, 30 and 35 °C, based on the optimal growth temperatures for each strain at a volume of 1.6 L in 2 L Schott bottles without shaking. Efforts to cultivate the strains at lower temperatures were unsuccessful, potentially due to extremely slow growth rates. The cultures were harvested at the mid-exponential phase and the late exponential/stationary phase as determined by the nitrite concentration of the cultures for each temperature experiment using glass fiber filters of 0.3 µm diameter pore size (GF-75, Advantec, Japan) and stored at −80 °C until further use.
2.3 Lipid extraction and analysis
The GF-75 filters were freeze-dried and extracted using a modified Bligh and Dyer method (Bligh and Dyer, 1959; Sturt et al., 2004; Schouten et al., 2008). The filters were extracted twice ultrasonically in a mixture of methanol (MeOH), dichloromethane (DCM) and phosphate buffer (, ) for 10 min. The supernatant was collected after centrifuging the mixture at 3000 rpm for 2 min. To separate the phases, additional DCM and phosphate buffer were added to the combined supernatants, resulting in a final solvent ratio of (). The organic phase was collected after centrifuging the mixture at 3000 rpm for 2 min, and then the aqueous phase was extracted thrice using DCM. These steps were repeated with the same residue but using a mixture of MeOH, DCM and trichloroacetic acid (, ). The final combined extract was then dried under N2 and stored at −20 °C until analysis. Prior to analysis, an internal standard, deuterated diacylglyceryltrimethylhomoserine (DGTS D-9, Avanti Polar Lipids), was added to the extracts. The extracts with the internal standard were then redissolved in MeOH:DCM (9:1, v:v) and filtered through a 0.45 µm, 4 mm diameter regenerated cellulose syringe filter (BGB Analytik, USA).
IPLs were analyzed according to Wörmer et al. (2013) with modifications as described in Bale et al. (2021). Briefly, samples were analyzed using Agilent 1290 Infinity I UHPLC (ultra-high-performance liquid chromatography) coupled to a Q Exactive Orbitrap HRMS (high-resolution mass spectrometry) with Ion Max source and heated electrospray ionization (HESI) probe. The separation was achieved on Acquity BEH C18 column (Waters, 2.1 mm × 150 mm, 1.7 µm particle). A solvent gradient of MeOH : H2O : formic acid : aqueous ammonia (, v:v) (solvent A) and MeOH : isopropanol : formic acid : aqueous ammonia (, v:v) (solvent B) was used at a constant flow rate of 0.2 mL min−1 starting with 95 % A for 3 min, followed by decreasing A in a linear gradient to 40 % in 12 min and to 0 % in 50 min, and maintaining this until 80 min. Lipids were detected using positive ion monitoring of 350–2000, and the 10 most abundant ions of MS1 scan were used to obtain data-dependent MS2 spectra. In addition, we used dynamic exclusion (6.0 s) and an inclusion list with calculated values for all known isoGDGTs. Fragmentation was achieved using stepped normalized collision energy of 15, 22.5, and 30. isoGDGT and OH-isoGDGTs were quantified by integration of the appropriate peaks of the summed mass chromatograms within 3 ppm of the mass accuracy of relevant molecular ions (, and for isoGDGTs; [M+H−H2O]+, , and for OH-isoGDGTs) of IPLs, which contained them as core lipids. The crenarchaeol isomer (cren′) was tentatively identified by its identical mass spectrum as that of crenarchaeol and its elution order compared with other isoGDGTs in the reverse-phase chromatography.
Our approach is different from core-top studies and most other culture studies, where core lipid compositions rather than intact polar lipid compositions are investigated. However, analysis of core lipids from archaeal biomass generally involves acid hydrolysis of lipid extracts or biomass in order to remove headgroups. Unfortunately, hydroxylated isoGDGTs, the main topic of our study, can potentially undergo partial dehydration, resulting in the formation of, e.g., unsaturated GDGTs (Liu et al., 2012b). This acid hydrolysis would lead to underestimations of OH-isoGDGT abundances and biases when calculating indices of core lipids. Therefore, we restricted ourselves to analyzing the IPL composition of the archaeal biomass and inferred core lipid compositions from the IPLs.
The abundance of OH-isoGDGTs relative to summed abundances of OH-isoGDGTs and regular isoGDGTs isoGDGTs (% OH; Eq. 1; modified after adding OH-isoGDGT-3 and OH-isoGDGT-4 to equation from Huguet et al., 2013), the ring index of OH-isoGDGTs (RI-OH′; Eq. 2; modified after adding OH-isoGDGT with three and four cyclopentane moieties (OH-isoGDGT-3 and OH-isoGDGT-4, respectively) to equation from Lü et al., 2015), TEX86 (Eq. 3; Schouten et al., 2002), (Eq. 4; Varma et al., 2024a) and the ring index of regular isoGDGTs (RIisoGDGTs; Eq. 5; Pearson et al., 2004) were calculated as follows:
where , [OH-n] indicates hydroxylated isoprenoidal GDGTs with n number of cyclopentane rings and [n] indicates non-hydroxylated isoprenoidal GDGTs with n number of cyclopentane rings.
3.1 Intact polar lipid composition of N. piranensis D3C and N. adriaticus NF5 strains
Two strains of ammonia-oxidizing Thaumarchaeota, the N. piranensis D3C strain and the N. adriaticus NF5 strain, grown at different temperatures (25, 30 and 35 °C for the N. piranensis D3C strain; 20, 25 and 30 °C for the N. adriaticus NF5 strain) and harvested at mid-exponential and stationary phases, were analyzed for isoGDGTs and OH-isoGDGTs by performing intact polar lipid analysis. The main isoGDGT core lipids observed in the IPLs were isoGDGTs with zero to four cyclopentane moieties; crenarchaeol; and, tentatively, the crenarchaeol isomer (Fig. S1 in the Supplement and Table S1 in the Supplement). The main OH-isoGDGTs observed were those with zero to four cyclopentane moieties. The headgroups of the IPLs consisted of monohexose (MH), dihexose (DH) and hexose-phosphohexose (HPH).
For the N. piranensis D3C strain, the HPH headgroup was mainly associated with isoGDGT-0 (∼ 18 %–55 %); crenarchaeol (∼ 11 %–70 %); isoGDGT-1 (∼ 5 %–17 %); and minor amounts of isoGDGT-2, isoGDGT-3 and isoGDGT-4 (∼ 0.1 %–7 %) as core lipids (Fig. S1 and Table S1). No HPH-OH-isoGDGTs were detected. Similar to HPH, IPLs with a MH headgroup consisted of isoGDGT-0 (∼ 17 %–71 %), crenarchaeol (∼ 20 %–44 %), isoGDGT-1 (∼ 10 %–22 %), isoGDGT-2 (∼ 3 %–11 %), and minor amounts of isoGDGT-3 and isoGDGT-4 (< 4 %) core lipids. Although the overall abundance of MH-OH-isoGDGTs was low (average ∼ 0.03 %, considering the cultures from different temperatures and growth phases analyzed), the OH-isoGDGT-0, OH-isoGDGT-1 and OH-isoGDGT-2 core lipids were detected for some of the cultures (Table S1). In contrast, IPLs with a DH headgroup had isoGDGT-1 (∼ 1 %–31 %), isoGDGT-2 (∼ 7 %–38 %), isoGDGT-4 (∼ 7 %–36 %) and moderate amounts of isoGDGT-3 (∼ 4 %–11 %) as core lipids, with lower abundances of isoGDGT-0 and crenarchaeol (< 4 %), as well as the crenarchaeol isomer (∼ 1 %–2 %). They also contained relatively high abundance of OH-isoGDGT-1 (∼ 1 %–6 %) and OH-isoGDGT-2 (∼ 2 %–22 %), while OH-isoGDGT-0, OH-isoGDGT-3 and OH-isoGDGT-4 were present in lower abundance of < 2 %. Finally, isoGDGT-0 to isoGDGT-4 and crenarchaeol core lipids lacking any headgroups were also identified, while OH-isoGDGT core lipids without a headgroup were not observed.
For the N. adriaticus NF5 strain, the IPLs with an HPH headgroup consisted mostly of isoGDGT-0 (∼ 12 %–83 %); crenarchaeol (∼ 5 %–31 %); isoGDGT-1 (∼ 3 %–15 %); and < 4 % of isoGDGT-2, isoGDGT-3 and isoGDGT-4 as core lipids (Fig. S2 in the Supplement and Table S2 in the Supplement). Unlike in N. piranensis D3C, HPH-OH-isoGDGT-0 to HPH-OH-isoGDGT-2 were detected in the N. adriaticus NF5 strain, although at relatively low abundances of < 2 %. The MH-isoGDGTs comprised ∼ 10 %–33 % isoGDGT-0; ∼ 12 %–25 % crenarchaeol; ∼ 4 %–8 % isoGDGT-1; and < 3 % of isoGDGT-2, isoGDGT-3 and isoGDGT-4 core lipids. MH-OH-isoGDGTs were more abundant in the N. adriaticus NF5 strain than in the N. piranensis D3C strain and consisted of ∼ 18 %–57 % of OH-isoGDGT-0, ∼ 6 %–12 % of OH-isoGDGT-1 and ∼ 0.6 %–4 % of OH-isoGDGT-2. The DH headgroup was primarily associated with isoGDGT-1 (∼ 2 %–20 %), isoGDGT-2 (∼ 5 %–22 %), isoGDGT-3 (∼ 3 %–7 %) and isoGDGT-4 (∼ 2 %–9 %) core lipids among the regular isoGDGTs, with smaller proportions of < 1 % of isoGDGT-0, crenarchaeol and crenarchaeol isomer. OH-isoGDGT-0 to OH-isoGDGT-4 were observed as core lipids for DH-OH-isoGDGTs with predominantly OH-isoGDGT-2 (∼ 19 %–52 %) and OH-isoGDGT-1 (∼ 6 %–47 %). Core lipids without any headgroup consisted of isoGDGT-0 to isoGDGT-4 and crenarchaeol, while no OH-isoGDGT core lipids were observed, similar to the N. piranensis D3C strain.
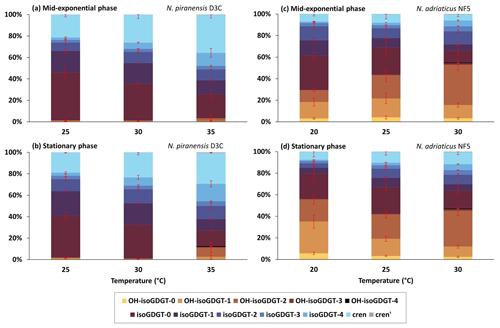
Figure 1Relative abundance of core lipids (inferred from IPL analysis) of isoGDGTs and OH-isoGDGTs at different temperatures for (a) the N. piranensis D3C strain harvested at the mid-exponential phase, (b) the N. piranensis D3C strain harvested at the stationary phase, (c) the N. adriaticus NF5 strain harvested at the mid-exponential phase and (d) the N. adriaticus NF5 strain harvested at the stationary phase. Error bars denote the standard deviation of the triplicates. Note that since we do not correct for differences in ionization efficiencies for the different headgroups, the actual relative abundance of each headgroup may be different.
In order to facilitate comparisons of our IPL results with those of IPLs and core lipid results from other cultures and natural environments, we calculated the summed amount of core lipids across all headgroups and plotted the overall relative abundance of each core lipid (Fig. 1). This assumes that all the IPLs have similar ionization efficiencies, an assumption which is likely incorrect. However, it does facilitate comparisons of trends in core lipid compositions – the main aim of our study. Furthermore, we note that there are also likely biases in reported core lipid compositions due to OH-isoGDGTs having varying ionization efficiencies compared to isoGDGTs for different laboratories (see Varma et al., 2024a) and because OH-isoGDGTs may be underrepresented due to losses incurred during acid hydrolysis. Due to differences in, e.g., ionization efficiencies between IPL analysis, using electron spray ionization, and core lipid analysis, using atmospheric chemical ionization, quantitative differences are likely when comparing our results with those of the core lipid composition of other cultures and environments. However, trends, e.g., changing relative abundances with temperature, will remain comparable.
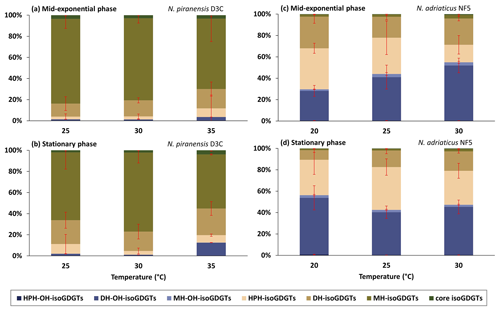
Figure 2Relative abundance based on total ion intensity of headgroups of isoGDGTs and OH-isoGDGTs at different temperatures from (a) the N. piranensis D3C strain harvested at the mid-exponential phase, (b) the N. piranensis D3C strain harvested at the stationary phase, (c) the N. adriaticus NF5 strain harvested at the mid-exponential phase and (d) the N. adriaticus D3C strain harvested at the stationary phase. HPH, DH and MH denote hexose-phosphohexose, dihexose and monohexose, respectively. Error bars denote the standard deviation of the triplicates Note that since we do not correct for differences in ionization efficiencies for the different headgroups, the actual relative abundance of each headgroup may be different.
The core lipid composition and the headgroup composition of the N. piranensis D3C strain and the N. adriaticus NF5 strain show substantial differences between the two strains (Figs. 1 and 2), especially regarding the relative abundance of OH-isoGDGTs. This suggests that large variations in the lipid composition exist among enrichment cultures of different strains, despite the fact that they are derived from same environment (i.e., Adriatic surface waters) and have a relatively high genetic relatedness (Bayer et al., 2016). Below we discuss the impact of growth phases and growth temperatures on the lipid composition of the cultures as well as species differences.
3.2 Impact of growth phase on isoGDGT and OH-isoGDGT distribution
The nitrite concentration of the cultures was used as a proxy to assess their growth phases, and the cells were harvested when the nitrite concentration was ∼ 450 µM at the mid-exponential phase and ∼ 900 µM at the late exponential/stationary phase (Fig. S3 in the Supplement). A cell abundance of 107 and 108 cells mL−1 was observed at the mid-exponential phase and the stationary phase, respectively. The observed variations in isoGDGT and OH-isoGDGT distribution between the mid-exponential and stationary phases were generally small and temperature dependent (Fig. 1). For instance, for the N. piranensis D3C strain, a slightly higher relative abundance of OH-isoGDGTs during the stationary phase (average of triplicate experiments, 12.6 ± 7 %) compared to the mid-exponential phase (average of triplicate experiments, 3.5 ± 3.7 %) is observed at 35 °C (t test; p < 0.05) but not at 25 and 30 °C (Fig. 1a and b, Table S3 in the Supplement). From the mid-exponential phase to the stationary phase, isoGDGT-0 and crenarchaeol decrease (from 22.2 ± 3.9 % to 14.3 ± 0.3 % and from 35.4 ± 2.8 % to 28.7 ± 0.2 % for isoGDGT-0 and crenarchaeol, respectively), while OH-isoGDGT-0 slightly increases (from 0.1 ± 0.1 % to 0.3 ± 0.1 %), but only at 35 °C, while a decrease in isoGDGT-0 (from 34.3 ± 1 % to 31.3 ± 1.4 %) and an increase in isoGDGT-2 (from 10.4 ± 1 % to 13.2 ± 1.3 %) are only observed at 30 °C (t test; all at a significance of p < 0.05). The composition of headgroups does not change from the mid-exponential phase to the stationary phase in the N. piranensis D3C strain with all three growth temperatures (Fig. 2, Table S4 in the Supplement).
The N. adriaticus NF5 strain exhibits a higher proportion of OH-isoGDGTs during the stationary phase (average of triplicates 56.3 ± 12.1 %) compared to the mid-exponential phase (average of triplicates 29.6 ± 5.4 %) at 20 °C but not at 25 and 30 °C (Fig. 1, Table S3). At 20 °C, isoGDGT-1 and isoGDGT-2 decreases (from 14.2 ± 2.1 to 5.5 ± 1.3 % for isoGDGT-1 and from 13.2 ± 3.6 to 4 ± 0.8 % for isoGDGT-2) from the mid-exponential phase to the stationary phase (Fig. 1, Table S3). At 30 °C, the relative abundance of isoGDGT-2 decreases (from 12.2 ± 1.2 % to 8.8 ± 1.1 %) and crenarchaeol slightly increases (from 5.5 ± 2 % to 11.2 ± 2.3 %) from the mid-exponential phase to the stationary phase (t test; all at a significance of p < 0.05). However, no trend is observed at 25 °C. In terms of the headgroups, between the mid-exponential phase and the stationary phase across the tested growth temperatures, the fractional abundance of HPH-isoGDGTs does not show any consistent variations, while DH-isoGDGTs generally show a decrease at 20 and 30 °C (from 29.6 ± 6.2 % to 9 ± 2.1 % and from 24.6 ± 2.2 % to 18.2 ± 1.6 %, respectively) and MH-isoGDGTs show a decrease (from 3.4 ± 1 % to 2.1 ± 0.5 %) at 30 °C (t test; all at a significance of p < 0.05). For OH-isoGDGTs, the OH-isoGDGTs with HPH and DH headgroups show an increase from the mid-exponential phase to the stationary phase (from 0.3 ± 0.1 % to 0.8 ± 0.2 % and from 27.9 ± 5.1 % to 53 ± 11.3 %, respectively), and, correspondingly, MH-OH-isoGDGTs show a decrease (from 1.5 ± 0.2 to 2.5 ± 0.6 %), but only at 20 °C (Fig. 2, Table S4).
Our results are in contrast to those observed earlier for Nitrosopumilus maritimus SCM1, which showed an increase in MH-isoGDGTs and MH-OH-isoGDGTs and a substantial decrease in HPH-isoGDGTs from the early growth phase to the late stationary phase (Elling et al., 2014). Elling et al. (2014) suggested based on these results that the prevalence of HPH-isoGDGTs indicates growing metabolically active cells. Our results suggest that care has to be taken with this interpretation, as headgroup composition is species-dependent and growth phase differences are not reflected in the abundance of HPH-isoGDGTs relative to MH- and DH-isoGDGTs in our cultures. With respect to core lipids, N. maritimus showed a decrease in isoGDGT-0 and crenarchaeol and an increase in isoGDGT-2 from the exponential phase to the stationary phase (Elling et al., 2014). This is similar to some trends observed in the N. piranensis D3C strain, while no such trends are observed for the N. adriaticus NF5 strain in our study. This is interesting since N. maritimus SCM1 and N. piranensis D3C are more closely related to each other phylogenetically than either of them are to N. adriaticus NF5 (Zheng et al., 2024). This suggests that growth phase modulation of IPL and core lipid composition is not consistent among different species of Thaumarchaeota. Importantly, our results also suggest that growth phase does not impact OH-isoGDGT abundances and composition in a consistent manner between the mid-exponential phase and the stationary phase within the range of incubation temperature tested and across different species of the same genus.
3.3 Impact of growth temperature on isoGDGT and OH-isoGDGT distribution
The two strains were cultivated at different temperatures: at 25, 30 and 35 °C for the N. piranensis D3C strain, which has an optimal growth temperature at ∼ 32 °C, and at 20, 25 and 30 °C for the N. adriaticus NF5 strain, which has an optimal growth temperature at ∼ 30 °C (Bayer et al., 2016). We were unable to grow both strains at temperatures above 35 and below 20 °C, likely due to their slow growth rate at those temperatures (Bayer et al., 2016).
With respect to isoGDGT core lipids, we observed that for the N. piranensis D3C strain, isoGDGT-0 and isoGDGT-1 consistently decrease with increasing temperature, while isoGDGT-4 and crenarchaeol slightly increase (Fig. 1, Table S3). For the N. adriaticus NF5 strain, increasing growth temperature is generally associated with increases in relative abundance of isoGDGT-3, isoGDGT-4, and crenarchaeol isomer and a decrease in isoGDGT-0 and isoGDGT-1 (Fig. 1, Table S3). In contrast, the relative abundance of crenarchaeol remained low (< 12 %) and did not change with growth temperature. Our results show some similarities but also differences with other culture studies. The N. maritimus, NAOA2 and NAOA6 strains showed an increase in the relative abundance of crenarchaeol and a decrease in isoGDGT-0 and isoGDGT-1 with increasing growth temperature (Elling et al., 2015; Qin et al., 2015), while a decrease in isoGDGT-0 and an increase in crenarchaeol with increasing growth temperature was also observed for Nitrosopumilus oxyclinae HCE1 and Nitrosopumilus cobalaminigenes HCA1 (Qin et al., 2015), similar to our results for the N. piranensis D3C strain. However, the thermophilic Nitrosotenuis uzonensis exhibited a decrease in isoGDGT-0 to isoGDGT-4 and an increase in crenarchaeol and crenarchaeol isomer from 37 to 46 °C but showed no change from 46 to 50 °C (Bale et al., 2019). Looking at the lipid profiles of natural archaeal communities in marine environments, a decrease in isoGDGT-0 and an increase in isoGDGT-1 to isoGDGT-3, crenarchaeol and its isomer are observed with increasing water temperatures (e.g., Kim et al., 2010). Thus, in general, isoGDGT-0 tends to decrease with increasing temperature, while crenarchaeol increases (with the exception of the N. adriaticus NF5 strain, which has a low abundance of crenarchaeol), while isoGDGT-1 to isoGDGT-4 show variable patterns.
For the relative abundances of OH-isoGDGT of the N. piranensis D3C strain, no consistent trends with temperature are observed (Fig. 1, Table S3). Some differences are observed for the N. adriaticus NF5 strain; e.g., OH-isoGDGT-0 and OH-isoGDGT-1 decrease with increasing growth temperature in the stationary phase, and OH-isoGDGT-2 and OH-isoGDGT-3 increase with growth temperature in the mid-exponential phase and between 25 and 30 °C in the stationary phase. Altogether, however, we do not see substantial and consistent changes in the overall abundances of OH-isoGDGTs. If we normalize the OH-isoGDGTs on the total sum of OH-isoGDGTs, we observe that for both N. piranensis D3C and N. adriaticus NF5 strains, the relative abundance of OH-isoGDGT-0 and OH-isoGDGT-1 decreases (from 10 to 2 % and from 52 to 19 %, respectively, for OH-isoGDGT-0 and OH-isoGDGT-1), and OH-isoGDGT-2 increases (from 37 to 70 %) with temperature (Fig. S4 in the Supplement). This is similar to the trend observed for OH-isoGDGT core lipids in the marine environment (Lü et al., 2015; Varma et al., 2024a).
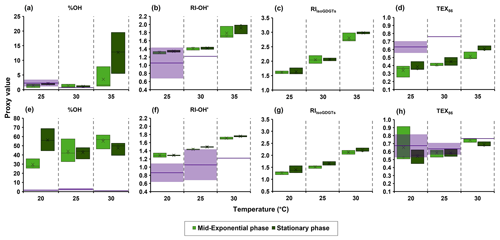
Figure 3The isoGDGT- and OH-isoGDGT-based proxies with temperature from IPLs of (a–d) the Nitrosopumilus piranensis D3C strain and (e–h) the Nitrosopumilus adriaticus NF5 strain, harvested at mid-exponential and stationary phases. Purple lines and shaded areas denote the observed means and 1 standard deviation, respectively, for each proxy from global marine surface sediments for sea surface temperature (± 1 °C) according to compiled datasets based on core lipids from Varma et al. (2024a) and Rattanasriampaipong et al. (2022).
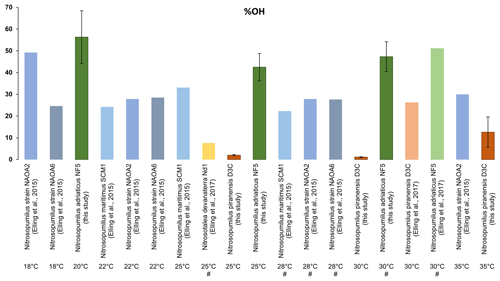
Figure 4Relative abundance of OH-isoGDGTs relative to all isoGDGTs plus OH-isoGDGTs inferred from the IPLs, observed in different cultures of Thaumarchaeota grown at different temperatures and harvested at the stationary phase. Values from previous studies (Elling et al., 2017, 2015) were calculated according to IPL data reported. The optimal temperature of each strain is denoted by “#”. Error bars denote standard deviation for the triplicates used in this study.
We calculated the OH-isoGDGT-based proxies, % OH and RI-OH′, and regular isoGDGT-based proxies, TEX86 and RIisoGDGTs, from the core lipid composition of the IPLs for the two strains we investigated (Fig. 3). We observe similar % OH values of 1.5 % at 25 and 30 °C for the N. piranensis D3C strain, as well as a somewhat higher percentage at 35 °C (4 % and 13 % in mid-exponential and stationary phases, respectively; Fig. 3). An increase in % OH values with growth temperature is observed in the N. adriaticus NF5 strain at the mid-exponential phase (from 30 to 55 %), while no apparent trend is observed for stationary phase cultures of N. adriaticus NF5. Thus, the % OH does not strongly respond to temperature, in contrast to the natural marine environment, where the relative abundance of OH-isoGDGTs increases (to maximum value of 17 %) with decreasing temperature (e.g., Huguet et al., 2013; Varma et al., 2024a). However, it should be noted that in the marine environment, the relative abundance of OH-isoGDGTs is quite low at temperatures > 20 °C (< 2 %) and only substantially increases below ∼ 15 °C (Huguet et al., 2013; Varma et al., 2024a). These temperatures are much lower than the growth temperatures used in this study (20–35 °C), and thus it may be that the relative abundance of OH-isoGDGTs substantially increases only below a certain temperature threshold. Unfortunately, we were unable to grow sufficient amount of biomass for both of the thaumarchaeotal species at temperatures lower than 20 °C because of their extremely low growth rates in those conditions. We also observe that the N. adriaticus NF5 strain has an apparent higher relative abundance of OH-isoGDGTs compared to that found in global surface sediments from environments with similar water temperatures (Fig. 3), although, as noted above, quantitative comparisons are difficult due to the fact that we cannot correct for differences in ionization efficiencies of IPLs. More importantly, there are substantial differences in the relative abundances between the two strains; i.e., N. piranensis D3C has much lower fractional abundance of OH-isoGDGTs (1 %–11 %) than N. adriaticus NF5 (29 %–56 %) (Fig. 1). Calculation of the relative abundances of OH-isoGDGTs in other cultures of Thaumarchaeota (Fig. 4; IPL data from Elling et al., 2015, 2017) also show large variations. Furthermore, for each strain, the OH-isoGDGT abundance at optimal temperatures does not seem to be substantially different from sub-optimal growth temperatures (Fig. S5 in the Supplement). Other than Ca. Nitrosotalea devanaterra Nd1 having a very low % OH value, closely related strains of Nitrosopumilus also do not show any particular trend (Fig. S5). This suggests that % OH values may be impacted by species composition in the natural environment.
With respect to RI-OH′, we observe a consistent increase with temperature for both N. piranensis D3C and N. adriaticus NF5 strains, with values slightly higher than RI-OH′ values observed for core lipids in marine surface sediments from a similar sea surface temperature regime (Fig. 3). Interestingly, since the sedimentary OH-isoGDGT signal, similar to regular isoGDGTs, is more likely to be coming from subsurface waters with lower temperatures (see Varma et al., 2024a), this would align the environmental RI-OH′ values with the observed RI-OH′ values in the cultures. For the regular isoGDGTs, the RIisoGDGTs increases with temperature for both strains, while for TEX86, the N. piranensis D3C strain shows an increase with increasing temperature, but the N. adriaticus NF5 strain shows only an increase in TEX86 from 25 to 30 °C (Fig. 3). This agrees with previous observations (Bale et al., 2019; Elling et al., 2015; Qin et al., 2015) where RIisoGDGTs shows a better response to temperature than TEX86 for different cultures of Thaumarchaeota. The also shows a trend with temperature that is similar to TEX86 for the N. piranensis D3C strain due to the low abundance of OH-isoGDGTs in this strain (Fig. S6 in the Supplement). However, in the N. adriaticus NF5 strain, the shows an increasing trend with temperature in the stationary phase unlike TEX86, although this is not observed for the mid-exponential phase. Thus, the ring indices of both isoGDGTs and OH-isoGDGTs consistently increase with growth temperature, while the relative abundance of OH-isoGDGTs seems independent of growth temperature, at least for the temperature range and species used in this study.
3.4 Comparison of IPLs with different headgroups
The headgroup compositions do not exhibit a consistent change with temperature for both N. piranensis D3C and N. adriaticus NF5 strains (Figs. 2, S1, and S2, Table S4). This is similar to what was reported for N. maritimus, but NAOA6 showed a considerable decrease in MH-isoGDGTs, while NAOA2 showed increase in HPH-isoGDGTs with temperature (Elling et al., 2015). Bale et al. (2019) observed no substantial change in headgroup composition in N. uzonensis between 37 and 46 °C, while an increase in MH and DH headgroups of isoGDGTs and a decrease in HPH-isoGDGTs were observed between 46 and 50 °C. This suggests that headgroup composition may not be influenced by changes in temperature in a consistent manner but mainly differs between different strains.
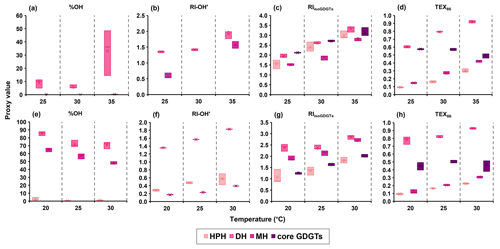
Figure 5Correlations of isoGDGT- and OH-isoGDGT-based proxies with temperature for different headgroups of IPLs from (a–d) the Nitrosopumilus piranensis D3C strain and (e–h) the Nitrosopumilus adriaticus NF5 strain, harvested at the stationary phase.
Interestingly, we do observe large differences in distribution of core lipids between the different headgroups (Figs. S1 and S2). Indeed, different proxy values and trends are observed when they are calculated for each headgroup (Figs. 5 and S7 in the Supplement). For example, % OH, RI-OH′, and TEX86 for the DH headgroup have substantially higher values compared to those of other headgroups. This observation is not unusual; for example, the ring index of isoGDGTs and TEX86 values have been shown to vary strongly between IPLs with different headgroups in culture studies as well as in water column and sediments (Elling et al., 2014; Lengger et al., 2012; Schouten et al., 2012; Basse et al., 2014). Comparison of N. piranensis D3C and N. adriaticus NF5 strains shows that OH-isoGDGTs mainly occur with DH headgroup and only to a minor extent with MH and HPH (Fig. 2). This agrees with the results of the same strains from Elling et al. (2017), who also observed a predominance of OH-isoGDGTs in IPLs with a DH headgroup.
With respect to , TEX86 and RIisoGDGTs, all of them increase with increasing temperature for all three headgroups for N. piranensis D3C and N. adriaticus NF5 strains, and only TEX86 and based on core lipids show no increase (Figs. 5 and S7). In contrast, RIisoGDGTs for N. maritimus, NAOA6 and NAOA2 show variable trends in the response of different IPLs to increasing growth temperature (Elling et al., 2015). For OH-isoGDGT-based proxies, some trends in % OH and RI-OH′ are more prominent for specific IPLs. For example, a substantially higher % OH is observed for IPLs with a DH headgroup in both strains, and for the N. adriaticus NF5 strain, IPLs with MH and DH headgroups show a slight decrease in % OH with increasing growth temperature (Fig. 5). For RI-OH′, a slight increase with temperature is observed for IPLs with DH and MH groups in the N. piranensis D3C strain, and for the N. adriaticus NF5 strain this trend is apparent for all IPL headgroups (Figs. 3 and 5).
The fact that OH-isoGDGT-derived proxies show such strong differences between IPLs with different headgroups may become relevant when IPLs are transformed to core lipids upon cell death and settling to the sediment floor in the natural environment. IPLs are known to have substantially different degradation rates, with HPH IPLs thought to degrade much faster than IPLs with MH or DH headgroups (Schouten et al., 2012; Xie et al., 2014). If so, then this could substantially alter the amount and possibly even distribution of OH-isoGDGTs upon degradation, with OH-isoGDGTs initially being present at lower abundances as fossil lipids compared to how they occurred as IPLs in the living cell as they are mainly tied to the DH headgroup, which degrades much slower than IPLs with the HPH headgroup, containing hardly any OH-isoGDGTs (Figs. 2 and 5a, e).
3.5 Implications for OH-isoGDGT-based proxies
The results of this culture study, combined with previous research, reveal that the abundance of OH-isoGDGTs (% OH) does not exhibit a consistent relationship with temperature, while large variations in % OH values are observed between strains (Elling et al., 2015, 2017; Bale et al., 2019; this study). This suggests that the species composition may be an important factor controlling OH-isoGDGT abundances in the marine environment. If so, the observed trends in % OH with temperature could be attributed to changes in species composition, with polar species potentially exhibiting the highest OH-isoGDGT abundances. However, the lack of data for cultures grown at temperatures < 15 °C renders this hypothesis still uncertain. In contrast, our culture study showed a general increase in RI-OH′ with an increase in temperature for Thaumarchaeota, in agreement with trends observed in natural environment (Lü et al., 2015). This suggests that temperature is the main factor controlling the degree of cyclization of OH-isoGDGTs, and, thus, RI-OH′ may be a suitable temperature proxy. Indeed, RI-OH′ has been successfully applied (e.g., Morcillo-Montalbá et al., 2021; Sinninghe Damsté et al., 2022; Davtian and Bard, 2023), although the absolute values of the reconstructed temperatures sometimes differed from those obtained using other proxies, such as and/or TEX86 (Davtian et al., 2021; Morcillo-Montalbá et al., 2021; Varma et al., 2023). This could potentially be resolved by conducting further culture studies where a true temperature calibration of RI-OH′ can be achieved.
Regarding , similar findings to those of TEX86 are observed; i.e., it does not consistently correlate with temperature across all cultivated Thaumarchaeota. This limitation may be partly due to the growth temperatures being always ≥ 18 °C in these studies. Species-specific variations in and TEX86 proxy values and their trends with temperature are also observed for these proxies. Consequently, TEX86 (e.g., Elling et al., 2017; Bale et al., 2019; Elling et al., 2015; Qin et al., 2015) and might also be controlled by species composition. Nevertheless, despite these limitations, TEX86 has proven to be a suitable tool for reconstructing temperatures in records covering the Cenozoic (see Schouten et al., 2013; Tierney, 2013), and the inclusion of OH-isoGDGT-0 in the improves the potential of the proxy considerably for polar regions (Varma et al., 2024a).
Finally, OH-isoGDGT core lipids are primarily found to be associated with the dihexose headgroup, which is thought to be relatively more resistant to degradation compared to phospho-headgroups (Schouten et al., 2010). This indicates that OH-isoGDGT core lipids may only be released gradually during diagenesis, potentially leading to biases in OH-isoGDGT-based proxies, including % OH, RI-OH′ and . Future studies examining the (oxic) degradation of OH-isoGDGTs, similar to previous studies conducted on regular isoGDGTs (Schouten et al., 2004; Kim et al., 2009; Lengger et al., 2014), may be beneficial in investigating this bias.
This study shows how the lipid composition, specifically OH-isoGDGTs and the proxies derived from them, are affected by growth temperature and growth phase in two strains of Thaumarchaeota. No systematic trends are observed in the core lipid and headgroup composition between the mid-exponential and stationary phases for both N. piranensis D3C and N. adriaticus NF5 strains. Growth temperatures do cause some changes in lipid composition, mainly in the degree of cyclization of isoGDGTs and OH-isoGDGTs. Indeed, RI-OH′ increases with increasing temperature in both cultures, similar to the marine environment, in contrast to % OH, which remains relatively constant. The N. adriaticus NF5 strain shows a substantially higher abundance of OH-isoGDGTs compared to N. piranensis D3C, suggesting species composition impacts % OH. OH-isoGDGT-based proxies calculated from intact polar lipids with different headgroups also show considerable differences between the headgroups and variations in their response to temperature in both strains, similar to regular isoGDGT-based proxies. This suggests that species composition and variations in IPLs and their degradation rates may have an impact on % OH in the natural environment. Further studies investigating the OH-isoGDGTs in archaeal cultures grown at temperatures < 15 °C, e.g., from species isolated from cold/ polar regions, are needed to provide insights into the role of OH-isoGDGTs in archaeal membrane adaptation.
All data related to this article are available online through the DOI https://dataportal.nioz.nl/doi/10.25850/nioz/7b.b.kh (Varma et al., 2024b).
The supplement related to this article is available online at: https://doi.org/10.5194/bg-21-4875-2024-supplement.
SS, LV and DV designed the experiments. DV conducted lab experiments, which included culturing work, lipid extraction and analysis. NJB assisted with lipid data analysis from UHPLC. DV performed the data analysis and wrote the manuscript together with all co-authors. SS, LV, PO and GJR supervised the project, provided critical feedback, and contributed to shaping the research and manuscript.
The contact author has declared that none of the authors has any competing interests.
Publisher's note: Copernicus Publications remains neutral with regard to jurisdictional claims made in the text, published maps, institutional affiliations, or any other geographical representation in this paper. While Copernicus Publications makes every effort to include appropriate place names, the final responsibility lies with the authors.
We thank Barabara Bayer for providing the cultures used in this study and Monique Verweij and Denise Dorhout for analytical support. This work was carried out under the program of the Netherlands Earth System Science Centre (NESSC).
This research has been supported by the Netherlands Earth System Science Centre (grant no. 024.002.001) and the European Union's Horizon 2020 (grant no. 847504).
This paper was edited by Sebastian Naeher and reviewed by two anonymous referees.
Bale, N. J., Palatinszky, M., Rijpstra, W. I. C., Herbold, C. W., Wagner, M., and Sinninghe Damsté, J. S.: Membrane lipid composition of the moderately thermophilic ammonia-oxidizing archaeon “Candidatus Nitrosotenuis uzonensis” at different growth temperatures, Appl. Environ. Microb., 85, e01332-19, https://doi.org/10.1128/AEM.01332-19, 2019.
Bale, N. J., Ding, S., Hopmans, E. C., Arts, M. G. I., Villanueva, L., Boschman, C., Haas, A. F., Schouten, S., and Sinninghe Damsté, J. S.: Lipidomics of environmental microbial communities. I: Visualization of component distributions using untargeted analysis of high-resolution mass spectrometry data, Front. Microbiol., 12, 659302, https://doi.org/10.3389/FMICB.2021.659302, 2021.
Basse, A., Zhu, C., Versteegh, G. J. M., Fischer, G., Hinrichs, K. U., and Mollenhauer, G.: Distribution of intact and core tetraether lipids in water column profiles of suspended particulate matter off Cape Blanc, NW Africa, Org. Geochem., 72, 1–13, https://doi.org/10.1016/J.ORGGEOCHEM.2014.04.007, 2014.
Bayer, B., Vojvoda, J., Offre, P., Alves, R. J. E., Elisabeth, N. H., Garcia, J. A. L., Volland, J. M., Srivastava, A., Schleper, C., and Herndl, G. J.: Physiological and genomic characterization of two novel marine thaumarchaeal strains indicates niche differentiation, ISME J., 10, 1051–1063, https://doi.org/10.1038/ismej.2015.200, 2016.
Bayer, B., Vojvoda, J., Reinthaler, T., Reyes, C., Pinto, M., and Herndl, G. J.: Nitrosopumilus adriaticus sp. nov. and Nitrosopumilus piranensis sp. nov., two ammonia-oxidizing archaea from the adriatic sea and members of the class nitrososphaeria, Int. J. Syst. Evol. Micr., 69, 1892–1902, https://doi.org/10.1099/ijsem.0.003360, 2019a.
Bayer, B., Pelikan, C., Bittner, M. J., Reinthaler, T., Könneke, M., Herndl, G. J., and Offre, P.: Proteomic response of three marine ammonia-oxidizing archaea to hydrogen peroxide and their metabolic interactions with a heterotrophic alphaproteobacterium, mSystems, 4, 15 pp., https://doi.org/10.1128/msystems.00181-19, 2019b.
Besseling, M. A., Hopmans, E. C., Koenen, M., van der Meer, M. T. J., Vreugdenhil, S., Schouten, S., Sinninghe Damsté, J. S., and Villanueva, L.: Depth-related differences in archaeal populations impact the isoprenoid tetraether lipid composition of the Mediterranean Sea water column, Org. Geochem., 135, 16–31, https://doi.org/10.1016/j.orggeochem.2019.06.008, 2019.
Blaga, C. I., Reichart, G. J., Heiri, O., and Sinninghe Damsté, J. S.: Tetraether membrane lipid distributions in water-column particulate matter and sediments: A study of 47 European lakes along a north-south transect, J. Paleolimnol., 41, 523–540, https://doi.org/10.1007/s10933-008-9242-2, 2009.
Bligh, E. G. and Dyer, W. J.: A rapid method of total lipid extraction and purification, Can. J. Biochem. Phys., 37, 911–917, https://doi.org/10.1139/O59-099, 1959.
Davtian, N. and Bard, E.: A new view on abrupt climate changes and the bipolar seesaw based on paleotemperatures from Iberian Margin sediments, P. Natl. Acad. Sci. USA, 120, e2209558120, https://doi.org/10.1073/PNAS.2209558120, 2023.
Davtian, N., Ménot, G., Fagault, Y., and Bard, E.: Western Mediterranean Sea paleothermometry over the Last Glacial Cycle based on the novel RI-OH index, Paleoceanogr. Paleoclimatology, 34, 616–634, https://doi.org/10.1029/2018PA003452, 2019.
Davtian, N., Bard, E., Darfeuil, S., Ménot, G., and Rostek, F.: The novel hydroxylated tetraether index RI-OH' as a sea surface temperature proxy for the 160–45 ka BP period off the Iberian margin, Paleoceanogr. Paleoclimatology, 36, 1–34, https://doi.org/10.1029/2020PA004077, 2021.
Elling, F. J., Könneke, M., Lipp, J. S., Becker, K. W., Gagen, E. J., and Hinrichs, K. U.: Effects of growth phase on the membrane lipid composition of the thaumarchaeon Nitrosopumilus maritimus and their implications for archaeal lipid distributions in the marine environment, Geochim. Cosmochim. Ac., 141, 579–597, https://doi.org/10.1016/j.gca.2014.07.005, 2014.
Elling, F. J., Könneke, M., Mußmann, M., Greve, A., and Hinrichs, K. U.: Influence of temperature, pH, and salinity on membrane lipid composition and TEX86 of marine planktonic thaumarchaeal isolates, Geochim. Cosmochim. Ac., 171, 238–255, https://doi.org/10.1016/j.gca.2015.09.004, 2015.
Elling, F. J., Könneke, M., Nicol, G. W., Stieglmeier, M., Bayer, B., Spieck, E., de la Torre, J. R., Becker, K. W., Thomm, M., Prosser, J. I., Herndl, G. J., Schleper, C., and Hinrichs, K. U.: Chemotaxonomic characterisation of the thaumarchaeal lipidome, Environ. Microbiol., 19, 2681–2700, https://doi.org/10.1111/1462-2920.13759, 2017.
Fietz, S., Ho, S. L., and Huguet, C.: Archaeal membrane lipid-based paleothermometry for applications in polar oceans, Oceanography, 33, 105–114, https://doi.org/10.5670/oceanog.2020.207, 2020.
Griess, P.: Bemerkungen zu der Abhandlung der HH. Weselsky und Benedikt “Ueber einige Azoverbindungen”?, Ber. Dtsch. Chem. Ges., 12, 426–428, https://doi.org/10.1002/CBER.187901201117, 1879.
Huguet, C., Fietz, S., and Rosell-Melé, A.: Global distribution patterns of hydroxy glycerol dialkyl glycerol tetraethers, Org. Geochem., 57, 107–118, https://doi.org/10.1016/j.orggeochem.2013.01.010, 2013.
Huguet, C., Fietz, S., Rosell-Melé, A., Daura, X., and Costenaro, L.: Molecular dynamics simulation study of the effect of glycerol dialkyl glycerol tetraether hydroxylation on membrane thermostability, BBA-Biomembranes, 1859, 966–974, https://doi.org/10.1016/j.bbamem.2017.02.009, 2017.
Hurley, S. J., Elling, F. J., Könneke, M., Buchwald, C., Wankel, S. D., Santoro, A. E., Lipp, J. S., Hinrichs, K. U., and Pearson, A.: Influence of ammonia oxidation rate on thaumarchaeal lipid composition and the TEX86 temperature proxy, P. Natl. Acad. Sci. USA, 113, 7762–7767, https://doi.org/10.1073/pnas.1518534113, 2016.
Hurley, S. J., Lipp, J. S., Close, H. G., Hinrichs, K. U., and Pearson, A.: Distribution and export of isoprenoid tetraether lipids in suspended particulate matter from the water column of the Western Atlantic Ocean, Org. Geochem., 116, 90–102, https://doi.org/10.1016/j.orggeochem.2017.11.010, 2018.
Inglis, G. N., Farnsworth, A., Lunt, D., Foster, G. L., Hollis, C. J., Pagani, M., Jardine, P. E., Pearson, P. N., Markwick, P., Galsworthy, A. M. J., Raynham, L., Taylor, K. W. R., and Pancost, R. D.: Descent toward the Icehouse: Eocene sea surface cooling inferred from GDGT distributions, Paleoceanography, 30, 1000–1020, https://doi.org/10.1002/2014PA002723, 2015.
Kim, J. H., Huguet, C., Zonneveld, K. A. F., Versteegh, G. J. M., Roeder, W., Sinninghe Damsté, J. S., and Schouten, S.: An experimental field study to test the stability of lipids used for the TEX86 and palaeothermometers, Geochim. Cosmochim. Ac., 73, 2888–2898, https://doi.org/10.1016/J.GCA.2009.02.030, 2009.
Kim, J. H., van der Meer, J., Schouten, S., Helmke, P., Willmott, V., Sangiorgi, F., Koç, N., Hopmans, E. C., and Sinninghe Damsté, J. S.: New indices and calibrations derived from the distribution of crenarchaeal isoprenoid tetraether lipids: Implications for past sea surface temperature reconstructions, Geochim. Cosmochim. Ac., 74, 4639–4654, https://doi.org/10.1016/j.gca.2010.05.027, 2010.
Lengger, S. K., Hopmans, E. C., Reichart, G. J., Nierop, K. G. J., Sinninghe Damsté, J. S., and Schouten, S.: Intact polar and core glycerol dibiphytanyl glycerol tetraether lipids in the Arabian Sea oxygen minimum zone. Part II: Selective preservation and degradation in sediments and consequences for the TEX86, Geochim. Cosmochim. Ac., 98, 244–258, https://doi.org/10.1016/J.GCA.2012.05.003, 2012.
Lengger, S. K., Hopmans, E. C., Sinninghe Damsté, J. S., and Schouten, S.: Impact of sedimentary degradation and deep water column production on GDGT abundance and distribution in surface sediments in the Arabian Sea: Implications for the TEX86 paleothermometer, Geochim. Cosmochim. Ac., 142, 386–399, https://doi.org/10.1016/j.gca.2014.07.013, 2014.
Liu, L., Guan, H., Xu, L., Sun, Z., and Wu, N.: Paleoclimate Records of the Middle Okinawa Trough Since the Middle Holocene: Modulation of the Low-Latitude Climate, Front. Earth Sci., 10, 1–13, https://doi.org/10.3389/feart.2022.799280, 2022.
Liu, X.-L., Summons, R. E., and Hinrichs, K. U.: Extending the known range of glycerol ether lipids in the environment: Structural assignments based on tandem mass spectral fragmentation patterns, Rapid Commun. Mass Spe., 26, 2295–2302, https://doi.org/10.1002/rcm.6355, 2012a.
Liu, X.-L., Lipp, J. S., Simpson, J. H., Lin, Y. S., Summons, R. E., and Hinrichs, K. U.: Mono- and dihydroxyl glycerol dibiphytanyl glycerol tetraethers in marine sediments: Identification of both core and intact polar lipid forms, Geochim. Cosmochim. Ac., 89, 102–115, https://doi.org/10.1016/j.gca.2012.04.053, 2012b.
Lü, X., Liu, X. L., Elling, F. J., Yang, H., Xie, S., Song, J., Li, X., Yuan, H., Li, N., and Hinrichs, K. U.: Hydroxylated isoprenoid GDGTs in Chinese coastal seas and their potential as a paleotemperature proxy for mid-to-low latitude marginal seas, Org. Geochem., 89–90, 31–43, https://doi.org/10.1016/j.orggeochem.2015.10.004, 2015.
Lü, X., Chen, J., Han, T., Yang, H., Wu, W., Ding, W., and Hinrichs, K. U.: Origin of hydroxyl GDGTs and regular isoprenoid GDGTs in suspended particulate matter of Yangtze River Estuary, Org. Geochem., 128, 78–85, https://doi.org/10.1016/j.orggeochem.2018.12.010, 2019.
Martens-Habbena, W., Berube, P. M., Urakawa, H., De La Torre, J. R., and Stahl, D. A.: Ammonia oxidation kinetics determine niche separation of nitrifying Archaea and Bacteria, Nature, 461, 976–979, https://doi.org/10.1038/nature08465, 2009.
Morcillo-Montalbá, L., Rodrigo-Gámiz, M., Martínez-Ruiz, F., Ortega-Huertas, M., Schouten, S., and Sinninghe Damsté, J. S.: Rapid climate changes in the westernmost Mediterranean (Alboran Sea) over the last 35 kyr: New insights from four lipid paleothermometers (, , RI-OH′, and LDI), Paleoceanogr. Paleocl., 36, e2020PA004171, https://doi.org/10.1029/2020PA004171, 2021.
O'Brien, C. L., Robinson, S. A., Pancost, R. D., Sinninghe Damsté, J. S., Schouten, S., Lunt, D. J., Alsenz, H., Bornemann, A., Bottini, C., Brassell, S. C., Farnsworth, A., Forster, A., Huber, B. T., Inglis, G. N., Jenkyns, H. C., Linnert, C., Littler, K., Markwick, P., McAnena, A., Mutterlose, J., Naafs, B. D. A., Püttmann, W., Sluijs, A., van Helmond, N. A. G. M., Vellekoop, J., Wagner, T., and Wrobel, N. E.: Cretaceous sea-surface temperature evolution: Constraints from TEX86 and planktonic foraminiferal oxygen isotopes, Earth-Sci. Rev., 172, 224–247, https://doi.org/10.1016/J.EARSCIREV.2017.07.012, 2017.
Pearson, A., Huang, Z., Ingalls, A. E., Romanek, C. S., Wiegel, J., Freeman, K. H., Smittenberg, R. H., and Zhang, C. L.: Nonmarine crenarchaeol in Nevada hot springs, Appl. Environ. Microb., 70, 5229–5237, https://doi.org/10.1128/AEM.70.9.5229-5237.2004, 2004.
Pitcher, A., Hopmans, E. C., Mosier, A. C., Park, S. J., Rhee, S. K., Francis, C. A., Schouten, S., and Sinninghe Damsté, J. S.: Core and intact polar glycerol dibiphytanyl glycerol tetraether lipids of ammonia-oxidizing Archaea enriched from marine and estuarine sediments, Appl. Environ. Microb., 77, 3468–3477, https://doi.org/10.1128/AEM.02758-10, 2011.
Polik, C. A., Elling, F. J., and Pearson, A.: Impacts of paleoecology on the TEX86 sea surface temperature proxy in the Pliocene-Pleistocene Mediterranean Sea, Paleoceanogr. Paleoclimatology, 33, 1472–1489, https://doi.org/10.1029/2018PA003494, 2018.
Qin, W., Carlson, L. T., Armbrust, E. V., Devol, A. H., Moffett, J. W., Stahl, D. A., and Ingalls, A. E.: Confounding effects of oxygen and temperature on the TEX86 signature of marine Thaumarchaeota, P. Natl. Acad. Sci. USA, 112, 10979–10984, https://doi.org/10.1073/pnas.1501568112, 2015.
Rattanasriampaipong, R., Zhang, Y. G., Pearson, A., Hedlund, B. P., and Zhang, S.: Archaeal lipids trace ecology and evolution of marine ammonia-oxidizing archaea, P. Natl. Acad. Sci. USA, 119, 1–10, https://doi.org/10.1073/pnas.2123193119, 2022.
Rinke, C., Chuvochina, M., Mussig, A. J., Chaumeil, P. A., Davín, A. A., Waite, D. W., Whitman, W. B., Parks, D. H., and Hugenholtz, P.: A standardized archaeal taxonomy for the Genome Taxonomy Database, Nat. Microbiol., 6, 946–959, https://doi.org/10.1038/s41564-021-00918-8, 2021.
Schouten, S., Hopmans, E. C., Schefuß, E., and Sinninghe Damsté, J. S.: Distributional variations in marine crenarchaeotal membrane lipids: A new tool for reconstructing ancient sea water temperatures?, Earth Planet. Sc. Lett., 204, 265–274, https://doi.org/10.1016/S0012-821X(02)00979-2, 2002.
Schouten, S., Hopmans, E. C., and Sinninghe Damsté, J. S.: The effect of maturity and depositional redox conditions on archaeal tetraether lipid palaeothermometry, Org. Geochem., 35, 567–571, https://doi.org/10.1016/J.ORGGEOCHEM.2004.01.012, 2004.
Schouten, S., Forster, A., Panoto, F. E., and Sinninghe Damsté, J. S.: Towards calibration of the TEX86 palaeothermometer for tropical sea surface temperatures in ancient greenhouse worlds, Org. Geochem., 38, 1537–1546, https://doi.org/10.1016/J.ORGGEOCHEM.2007.05.014, 2007.
Schouten, S., Hopmans, E. C., Baas, M., Boumann, H., Standfest, S., Könneke, M., Stahl, D. A., and Sinninghe Damsté, J. S.: Intact membrane lipids of “Candidatus Nitrosopumilus maritimus,” a cultivated representative of the cosmopolitan mesophilic group I crenarchaeota, Appl. Environ. Microb., 74, 2433–2440, https://doi.org/10.1128/aem.01709-07, 2008.
Schouten, S., Middelburg, J. J., Hopmans, E. C., and Sinninghe Damsté, J. S.: Fossilization and degradation of intact polar lipids in deep subsurface sediments: A theoretical approach, Geochim. Cosmochim. Ac., 74, 3806–3814, https://doi.org/10.1016/J.GCA.2010.03.029, 2010.
Schouten, S., Pitcher, A., Hopmans, E. C., Villanueva, L., van Bleijswijk, J., and Sinninghe Damsté, J. S.: Intact polar and core glycerol dibiphytanyl glycerol tetraether lipids in the Arabian Sea oxygen minimum zone: I. Selective preservation and degradation in the water column and consequences for the TEX86, Geochim. Cosmochim. Ac., 98, 228–243, https://doi.org/10.1016/j.gca.2012.05.002, 2012.
Schouten, S., Hopmans, E. C., and Sinninghe Damsté, J. S.: The organic geochemistry of glycerol dialkyl glycerol tetraether lipids: A review, Org. Geochem., 54, 19–61, https://doi.org/10.1016/j.orggeochem.2012.09.006, 2013.
Sinninghe Damsté, J. S., Schouten, S., Hopmans, E. C., Van Duin, A. C. T., and Geenevasen, J. A. J.: Crenarchaeol: The characteristic core glycerol dibiphytanyl glycerol tetraether membrane lipid of cosmopolitan pelagic crenarchaeota, J. Lipid Res., 43, 1641–1651, https://doi.org/10.1194/jlr.M200148-JLR200, 2002.
Sinninghe Damsté, J. S., Rijpstra, W. I. C., Hopmans, E. C., Jung, M. Y., Kim, J. G., Rhee, S. K., Stieglmeier, M., and Schleper, C.: Intact polar and core glycerol dibiphytanyl glycerol tetraether lipids of group I.1a and I.1b Thaumarchaeota in soil, Appl. Environ. Microb., 78, 6866–6874, https://doi.org/10.1128/AEM.01681-12, 2012.
Sinninghe Damsté, J. S., Warden, L. A., Berg, C., Jürgens, K., and Moros, M.: Evaluation of the distributions of hydroxylated glycerol dibiphytanyl glycerol tetraethers (GDGTs) in Holocene Baltic Sea sediments for reconstruction of sea surface temperature: the effect of changing salinity, Clim. Past, 18, 2271–2288, https://doi.org/10.5194/cp-18-2271-2022, 2022.
Sollai, M., Villanueva, L., Hopmans, E. C., Keil, R. G., and Sinninghe Damsté, J. S.: Archaeal sources of intact membrane lipid biomarkers in the oxygen deficient zone of the eastern tropical south Pacific, Front. Microbiol., 10, 765, https://doi.org/10.3389/FMICB.2019.00765, 2019.
Sturt, H. F., Summons, R. E., Smith, K., Elvert, M., and Hinrichs, K. U.: Intact polar membrane lipids in prokaryotes and sediments deciphered by high-performance liquid chromatography/electrospray ionization multistage mass spectrometry–new biomarkers for biogeochemistry and microbial ecology, Rapid Commun. Mass Spe., 18, 617–628, https://doi.org/10.1002/RCM.1378, 2004.
Taylor, K. W. R., Huber, M., Hollis, C. J., Hernandez-Sanchez, M. T., and Pancost, R. D.: Re-evaluating modern and Palaeogene GDGT distributions: Implications for SST reconstructions, Global Planet. Change, 108, 158–174, https://doi.org/10.1016/j.gloplacha.2013.06.011, 2013.
Tierney, J. E.: Biomarker-based inferences of past climate: the TEX86 paleotemperature Proxy, 2nd ed., Elsevier Ltd., 379–393, https://doi.org/10.1016/B978-0-08-095975-7.01032-9, 2013.
Trommer, G., Siccha, M., van der Meer, M. T. J., Schouten, S., Sinninghe Damsté, J. S., Schulz, H., Hemleben, C., and Kucera, M.: Distribution of Crenarchaeota tetraether membrane lipids in surface sediments from the Red Sea, Org. Geochem., 40, 724–731, https://doi.org/10.1016/j.orggeochem.2009.03.001, 2009.
Varma, D., Hättig, K., Meer, M. T. J. Van Der, and Reichart, G.: Constraining water depth influence on organic paleotemperature proxies using sedimentary archives, Paleoceanogr. Paleocl., 38, e2022PA004533, https://doi.org/10.1029/2022PA004533, 2023.
Varma, D., Hopmans, E. C., van Kemenade, Z. R., Kusch, S., Berg, S., Bale, N. J., Sangiorgi, F., Reichart, G. J., Sinninghe Damsté, J. S., and Schouten, S.: Evaluating isoprenoidal hydroxylated GDGT-based temperature proxies in surface sediments from the global ocean, Geochim. Cosmochim. Ac., 370, 113–127, https://doi.org/10.1016/J.GCA.2023.12.019, 2024a.
Varma, D., Villanueva, L., Bale, N., Offre, P., Reichart, G.-J., and Schouten, S.: Thaumarchaeotal culture data_NF5_D3C, NIOZ [data set], https://doi.org/10.25850/nioz/7b.b.kh, 2024b.
Weijers, J. W. H., Lim, K. L. H., Aquilina, A., Sinninghe Damsté, J. S., and Pancost, R. D.: Biogeochemical controls on glycerol dialkyl glycerol tetraether lipid distributions in sediments characterized by diffusive methane flux, Geochem. Geophy.. Geosy., 12, 1–15, https://doi.org/10.1029/2011GC003724, 2011.
Wörmer, L., Lipp, J. S., Schröder, J. M., and Hinrichs, K. U.: Application of two new LC–ESI–MS methods for improved detection of intact polar lipids (IPLs) in environmental samples, Org. Geochem., 59, 10–21, https://doi.org/10.1016/J.ORGGEOCHEM.2013.03.004, 2013.
Wuchter, C., Schouten, S., Coolen, M. J. L., and Sinninghe Damsté, J. S.: Temperature-dependent variation in the distribution of tetraether membrane lipids of marine Crenarchaeota: Implications for TEX86 paleothermometry, Paleoceanography, 19, 1–10, https://doi.org/10.1029/2004PA001041, 2004.
Xie, S., Liu, X. L., Schubotz, F., Wakeham, S. G., and Hinrichs, K. U.: Distribution of glycerol ether lipids in the oxygen minimum zone of the Eastern Tropical North Pacific Ocean, Org. Geochem., 71, 60–71, https://doi.org/10.1016/j.orggeochem.2014.04.006, 2014.
Zeng, Z., Liu, X.-L., Farley, K. R., Wei, J. H., Metcalf, W. W., Summons, R. E., and Welander, P. V: GDGT cyclization proteins identify the dominant archaeal sources of tetraether lipids in the ocean., P. Natl. Acad. Sci. USA, 116, 22505–22511, https://doi.org/10.1073/pnas.1909306116, 2019.
Zhang, T., He, W., Liang, Q., Zheng, F., Xiao, X., Zeng, Z., Zhou, J., Yao, W., Chen, H., Zhu, Y., Zhao, J., Zheng, Y., and Zhang, C.: Lipidomic diversity and proxy implications of archaea from cold seep sediments of the South China Sea, Front. Microbiol., 14, 1241958, https://doi.org/10.3389/FMICB.2023.1241958, 2023.
Zhang, Y. G., Zhang, C. L., Liu, X. L., Li, L., Hinrichs, K. U., and Noakes, J. E.: Methane Index: A tetraether archaeal lipid biomarker indicator for detecting the instability of marine gas hydrates, Earth Planet. Sc. Lett., 307, 525–534, https://doi.org/10.1016/j.epsl.2011.05.031, 2011.
Zhang, Y. G., Pagani, M., and Wang, Z.: Ring Index: A new strategy to evaluate the integrity of TEX86 paleothermometry, Paleoceanography, 31, 220–232, https://doi.org/10.1002/2015PA002848, 2016.
Zheng, Y., Wang, B., Gao, P., Yang, Y., Xu, B., Su, X., Ning, D., Tao, Q., Li, Q., and Zhao, F.: Novel order-level lineage of ammonia-oxidizing archaea, ISME J., 18, wrad002, https://doi.org/10.1093/ismejo/wrad002, 2024.