the Creative Commons Attribution 4.0 License.
the Creative Commons Attribution 4.0 License.
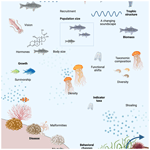
Reviews and syntheses: Biological indicators of low-oxygen stress in marine water-breathing animals
Michael R. Roman
Andrew H. Altieri
Denise Breitburg
Erica M. Ferrer
Natalya D. Gallo
Shin-ichi Ito
Karin Limburg
Kenneth Rose
Moriaki Yasuhara
Anthropogenic warming and nutrient over-enrichment of our oceans have resulted in significant, and often catastrophic, reductions in dissolved oxygen (deoxygenation). Stress on water-breathing animals from this deoxygenation has been shown to occur at all levels of biological organization: cellular, organ, individual, species, population, community, and ecosystem. Most climate forecasts predict increases in ocean deoxygenation; thus, it is essential to develop reliable biological indicators of low-oxygen stress that can be used by regional and global oxygen monitoring efforts to detect and assess the impacts of deoxygenation on ocean life. This review focuses on responses to low-oxygen stress that are manifest at different levels of biological organization and at a variety of spatial and temporal scales. We compare particular attributes of these biological indicators to the dissolved oxygen threshold of response, timescales of response, sensitive life stages and taxa, and the ability to scale the response to oxygen stress across levels of organization. Where there is available evidence, we discuss the interactions of other biological and abiotic stressors on the biological indicators of low-oxygen stress. We address the utility, confounding effects, and implementation of the biological indicators of oxygen stress for research and societal applications. Our hope is that further refinement and dissemination of these oxygen stress indicators will provide more direct support for environmental managers, fisheries and mariculture scientists, conservation professionals, and policymakers to confront the challenges of ocean deoxygenation. An improved understanding of the sensitivity of different ocean species, communities, and ecosystems to low-oxygen stress will empower efforts to design monitoring programs, assess ecosystem health, develop management guidelines, track conditions, and detect low-oxygen events.
- Article
(2198 KB) - Full-text XML
- BibTeX
- EndNote
Oxygen remains fundamental to the success of most marine life. As a result of both a warming planet and coastal eutrophication, oxygen-depleted waters (referred to here as deoxygenated) have increased in spatial and temporal extent in our oceans (Breitburg et al., 2018). Open-ocean oxygen minimum zones have expanded (Stramma et al., 2008, 2010), and coastal areas experiencing hypoxia (low or depleted dissolved oxygen) continue to increase worldwide (Diaz and Rosenberg, 2008; Dai et al., 2023). While some ocean biota evolved to live in permanently low-oxygen environments, normally oxygenated (normoxic) coastal waters are now experiencing periods of hypoxia that range from diel to seasonal in timescale and result in stress for water-breathing animals. In addition, increases in ocean temperatures, both gradual with climate change and episodic through events like marine heat waves, have decreased the solubility of oxygen across various marine ecosystems and increased organisms' metabolic demands and respiration such that deoxygenated waters are becoming less tolerable for marine animals (Woods et al., 2022). New anthropogenic initiatives such as the expansion of ocean aquaculture (e.g., Zhang et al., 2018a) and planned large-scale mitigation measures to enhance marine carbon sequestration (Levin et al., 2023) present new challenges with respect to deoxygenation. These current and future challenges reinforce the critical need to develop biological indicators of oxygen stress that can be used to assess and predict the effects of expanding deoxygenation on ocean biota.
Oxygen has been proposed as an indicator of ocean health and of large-scale restoration progress; for example, we can use oxygen content to monitor reduced nutrient loading (Grégoire et al., 2021). However, in addition to monitoring oxygen, biotic indicators of low-oxygen stress may provide more direct support for environmental managers, fisheries scientists, and policymakers in their efforts to better assess the sensitivity of different ocean species, communities, and ecosystems in response to oxygen content. Indicators enable us to use readily available and measurable data to develop a variable, or set of variables, that reflects the state of some aspect of the system that is important and worth monitoring. Indicators should have specific criteria when being evaluated for monitoring programs (e.g., Yoccoz et al., 2001; Reynolds et al., 2016). These criteria can include being readily quantifiable, responsive, and specific to the stressor of interest; operationally feasible; and able to detect the response to the stressor over space and time. Indicators are often used because they can be easier to measure than the actual aspect of the system they are designed to assess. For example, observations of changes in animal behavior (i.e., avoidance of hypoxic water) can sometimes be used as a quick, reliable, and inexpensive indicator of deoxygenation, whereas in situ sensors used to measure oxygen loss might be difficult to obtain, costly, and time intensive. Indicators can integrate exposure effects over space and time, and they are likely to reflect cause and effect as they tend to have a direct mechanistic link to a stressor of interest. Note, however, that indicators can be misleading if confounding variables are not considered or if the indicator does not have sufficient validation for the level of biological organization considered (e.g., species, population, community, or ecosystem).
Indicators have been widely used by organizations at the international level to assess the state of environmental health and sustainability. However, there remains an open need for research and development of indicators at the international level which focus on the biological effects of deoxygenation. For example, the Framework for Ocean Observing (Lindstrom et al., 2012) has identified numerous essential ocean variables (EOVs) intended to capture the fundamental characteristics of marine ecosystems that can be combined into indicators in order to represent natural complexity, track changes in the environment, reflect management performance, deliver information, and assess progress in achieving long-term goals (Miloslavich et al., 2018). EOVs selected for their societal and scientific responsiveness as well as their implementation readiness include the abiotic variable oxygen as well as biological variables such as biomass of phytoplankton and zooplankton, fish abundance, and coral cover. Garçon et al. (2019) examined the potential application of EOVs to understand biotic responses to oxycline changes within oxygen minimum zones (OMZs) for potential societal benefits such as improved fisheries management. The study of EOVs and similar environmental indicators can have tangible impacts on management and policy, with the potential to shape mitigation efforts and associated biodiversity policies. Thus, here we examine indicators that show biological and ecological responses of organisms to low dissolved oxygen (DO) in an effort to help guide those international efforts, local biological management programs, and field and laboratory research programs.
Low-oxygen stress has been shown to occur at all levels of biological organization (cellular, organ, individual, population, community, and ecosystem), with varying degrees of oxygen sensitivity and timescales of response (Woods et al., 2022; Fig. 1). Measurements are often performed on individuals and extrapolated to higher levels of biological organization, timescales, and spatial extent using various scaling methods that are conceptual, statistical, or simulation-based. Issues specifically related to indicators of low-DO effects include (a) differentiation of lethal versus sublethal responses; (b) the fact that exposure of individuals to low DO is time-dependent because DO varies spatially and temporally and mobile organisms move through dynamic DO fields; (c) low-DO exposure that is almost always part of a suite of abiotic stressors that covary to various degrees, thus making it difficult to isolate the responses to low DO; (d) scaling beyond the measured individual response to low DO that can be challenging because the responses integrate across the population, community, and ecosystem levels, which include a complex suite of biological interactions that are themselves affected by low oxygen.
Deoxygenation rarely acts alone as a stressor. It is frequently recognized to be a result of ocean warming and a product of increased respiration (which can be induced by higher temperatures or excess nutrients). Thus, it is common for hypoxia to co-occur with elevated temperature, lower pH and carbonate saturation state, presence of hydrogen sulfide, and/or increased food supply (Breitburg et al., 2019; Laffoley and Baxter, 2019). This means that some low-oxygen indicators can be confounded with other environmental factors, and attribution specifically to oxygen limitation becomes problematic. In a mixed-model meta-analysis of experimental studies, Sampaio et al. (2021) found that, relative to warming and acidification, hypoxic events tended to induce stronger negative effects on survival, abundance, development, metabolism, growth and reproduction across taxonomic groups (mollusks, crustaceans, and fish), ontogenetic stages, and climate regions studied. However, there were also clear interactions among stressors in their biotic effects (both antagonistic and synergistic; Sampaio et al., 2020). Reddin et al. (2020) compared the interactive effects of warming, hypoxia, and acidification in causing global marine extinction patterns to modern experimental results of the impacts of these stressors on marine organisms. They found that modern clade responses to these climate-related stressors correlated with the clade genus survival rates over the post-Cambrian Phanerozoic, with the interactions of dissolved oxygen and temperature having the strongest negative effects for tropical marine animals.
The focus of this review is on biological indicators of low-oxygen stress in water-breathing marine animals. We identify indicators that have been determined for different levels of biological organization, broadly defined as individuals, species/populations, and communities/ecosystems (Fig. 1). We compare particular attributes of these indicators to the oxygen threshold of response, timescales of responses, sensitive life stages and taxa, and the ability to scale up the response to oxygen stress to higher levels of organization.
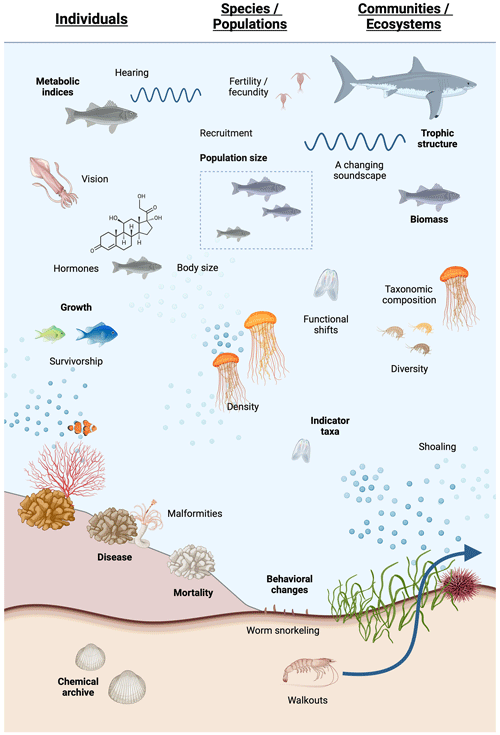
Figure 1Schematic of deoxygenation indicators discussed below for (left) individuals, (middle) populations and species, and (right) communities and ecosystems. Created with https://BioRender.com (last access: 21 August 2024).
2.1 Individuals
2.1.1 Cellular responses
The hypoxia inducible factor (HIF), and HIF-alpha in particular, is a transcription factor common to most metazoans that mediates gene expression in many of the pathways which regulate cellular responses to hypoxia, including metabolic depression, anaerobic metabolism, and mitophagy. Hypoxia and reactive oxygen species (ROS) can inhibit the degradation of HIF-alpha, leading to HIF-alpha accumulation in the cell. The response of the HIF-alpha subunit to hypoxia can be measured through gene expression using RNA sequencing (e.g., Alderdice et al., 2022). The relative amount of HIF-alpha and its location within a cell can be tracked using fluorescent protein tagging (e.g., Kallio et al., 1998). The amount of HIF-alpha is expressed at a higher rate, accumulates in the cell, and is translocated into the nucleus where it accumulates and is then associated with targeted metabolic responses. The response time of HIF expression varies between the species tested (Alderdice et al., 2020). HIFs play a pivotal role in regulating the metabolic response of cells to hypoxia, and a change in HIF is likely to have a number of cascading physiological effects. Application of this indicator would require molecular/cellular techniques to detect changes in HIF (i.e., access to specialized laboratory facilities). Baseline levels and response times vary among different species; thus, multiple time points would be required to know whether an individual is exhibiting an elevated response. HIF expression can also vary diurnally; therefore, treatment controls and temporal factors need to be considered for the use of this indicator.
HIF appears to be widespread across metazoan phyla (aside from sponges) and is therefore highly conserved (Rytkönen et al., 2011). However, there are differences between closely related taxa (congeners) in the timing and magnitude of the HIF response (Alderdice et al., 2020). Broad control of metabolic responses by HIFs is likely to mediate organismal responses to other stressors. More work is needed to determine why species differ in their baseline levels of HIF and the regulation of HIF with prolonged hypoxia exposure since sustained response could have negative/irreversible consequences for organisms.
2.1.2 Sensory systems
Low oxygen can impair the sensory systems of marine water-breathing animals. Changes in animal vision, olfaction, and perception of sound can be sensitive indicators of oxygen stress which impact population and community ecology. While promising, more research is needed to elucidate the primary and secondary effects of sensory impairment by low-oxygen stress on different groups of marine animals to be practical for implementation in monitoring programs.
Vision metrics which show a negative response to low DO include electroretinogram responses (McCormick et al., 2019), behavioral responses (swimming, sinking response to light), and distribution responses that are based on the loss/impairment of vision (McCormick and Levin, 2017). If some species require more light in deoxygenated waters, activities such as prey capture, predator avoidance, and mating may be impaired. Manifestations of visual impairment by hypoxia could include changes in behavior, shoaling distributions, and eye abnormalities (resulting from maternal hypoxia). The physical manifestations of hypoxia, such as abnormalities and growth defects, may be easier to detect than changing visual responses since visual response to low oxygen has been quantified in very few organisms. There is a need to study how deoxygenation might impact the vision of commercially harvested species, particularly since the vision of several larval species is highly sensitive to deoxygenated waters. Impaired vision could influence their survival (McCormick et al., 2022b) and hence be potentially useful for future management in considering susceptibility to catch and possible fisheries restrictions.
Similarly, animal perception of sound may also be impaired by the loss of oxygen. For example, studies on fish show temporary losses of hearing under anoxia; a lowered probability of a “fast startle” response, with an increased risk of falling prey and decreased likelihood of successful foraging; and, possibly, a reduced ability to communicate (Suzue et al., 1987; Sánchez-García et al., 2019). While some reduction in hearing response can occur rapidly with decreased oxygen (<24 h), morphological changes to fish otoliths in deoxygenated waters may occur over years. While animals' perception of sound underwater is changing due to deoxygenation, we can expect to see changes in the underwater soundscape itself that are associated with changes in populations and ecology. Anthropogenic warming is speeding up the rate at which sound travels underwater via changes in density (e.g., Affatati et al., 2022), while changes in oxygen may alter what sounds animals make or the frequency of those sounds.
Changes in the olfactory processes/responses of some marine animals may be associated with changes in oxygen. However, these changes are generally much harder to study and have not been adequately explored across marine fauna to be useful as an indicator at this time (Tigert and Porteus, 2023).
2.1.3 Hormonal changes influencing growth and fertility
Hypoxia exposure causes physiological stress, which increases endocrine cortisol levels relative to normoxic conditions in fish (Léger et al., 2021). Hypoxia can also alter the levels of growth hormones, thereby negatively affecting growth among individuals relative to normoxic conditions (Hou et al., 2020). Oxygen thresholds that give rise to changes in cortisol and growth hormone levels are specific to species and developmental life stage. Changes in cortisol levels can include rapid responses to acute hypoxia exposure or chronic responses to long-term hypoxia exposure. These types of changes in stress hormones can subsequently give rise to reduced immune function and feeding suppression (Gregory and Wood, 1999), potentially leading to reductions in growth or higher natural mortality. Measurements of cortisol in fish are typically conducted using an enzyme-linked immunosorbent assay (ELISA) in blood serum. Since plasma cortisol is the most commonly used indicator of stress in fish, there are ongoing efforts to develop improved protocols for cortisol measurement, including non-invasive methods using fish scales (Sadoul and Geffroy, 2019). Similarly, immunoassays can also be used to measure levels of growth hormone in fish. This indicator is not hypoxia-specific, however, and changes in cortisol or growth hormone levels can reflect responses to other stressors such as handling stress during sampling (which can artificially elevate cortisol). Thus, this indicator would likely work best in experimental or controlled settings such as aquaculture facilities. However, new approaches for measuring cortisol levels in fish scales may further extend the utility of this indicator to field studies/nature.
Hypoxia can disrupt a variety of other hormones in fish and invertebrates beyond stress and growth hormones and include hormones that control gonad development, sperm motility, and reproductive behaviors (Thomas and Rahman, 2009; Wu, 2002). At certain low-oxygen levels, reproduction is entirely inhibited and animals will not attempt to mate for reasons that are not fully understood but likely have to do with hormonal triggers. For example, the egg production rate of copepods has been shown to decrease in deoxygenated water (Ambler, 1985), and egg production overall is reduced in response to chronic oxygen limitation during the copepods' adult life stages. Rising temperatures and low-DO conditions have been linked to changes in copepod antioxidants that would normally protect lipids, proteins, and DNA, all of which are important building blocks for meiosis (von Weissenberg et al., 2022). Laboratory experiments also indicate that exposure to low-oxygen conditions can have transgenerational effects on fish reproduction. For example, Wang et al. (2016) found that hypoxia exposure among male medaka fish led to decreased spermatogenesis and reduced sperm motility in the F2 generation. Female medaka low-oxygen exposure led to greatly reduced hatching success in the F2 generation (Lai et al., 2019). In croaker fish (Micropogonias undulates), exposure to summertime hypoxia resulted in reduced fertility indicator values measured in the fall at the start of the adult spawning season (Thomas et al., 2015). Reductions in fertility were seen at DO levels above those typically associated with croakers' avoidance behavior, such that exposure to fertility-limiting DO levels was high and quite common among individuals found within the hypoxic zone of the Gulf of Mexico (Rose et al., 2017b). Reductions in fertility caused by limiting DO represent the sublethal effects of deoxygenation and can be linked to important changes in population dynamics (e.g., Richmond et al., 2006; Rose et al., 2017a, b).
The development of the fertility indicator illustrates how – through a series of coordinated laboratory experiments, field data collection, and modeling – cause and effect can be established between low-DO exposure and the resulting changes in the endocrine-based indicator. Detailed laboratory experiments enabled the causal links between low-DO exposure and endocrine responses within an individual female adult croaker (Thomas and Rahman, 2009; Rahman and Thomas, 2017). The laboratory data were used to develop a model of the endocrine functioning of vitellogenesis of individual fish (Murphy et al., 2009). This allowed examination of how the indicators measured as blood and organ concentrations would vary over time and under exposures not replicated in the laboratory. These model results were applied to field data from the northern Gulf of Mexico, and the indicators of hypoxia exposure/effects were used to assess hypoxia effects at the population level (Thomas et al., 2015; Rose et al., 2017a).
2.1.4 Growth/body size/condition factor
Growth, size, and condition respond to multiple biotic (i.e., food quality and quantity) and abiotic (oxygen, temperature, pH, salinity) factors. The high ecological relevance of growth, size, and condition is because they integrate physiology over multiple subprocesses (metabolism, feeding) and are influenced by multiple factors and stressors. Thus, while establishing cause-and-effect linkages between low DO and growth, size, and condition can be difficult, there is also extensive information available at the individual level regarding oxygen-induced changes in these factors that are derived from laboratory and field data. This abundance of data reflects the fact that body size and growth are relatively easy to measure and are important determinants of individual fitness because they directly influence other processes that are size- or condition-dependent (e.g., reproduction, mortality). A study indicated that the sensitivity of early life growth of estuarine fish to low DO is higher than that of low-pH conditions (Depasquale et al., 2015). Reduced growth is observed at DO levels above lethal levels and above DO levels that trigger avoidance in zooplankton and fish (Richmond et al., 2006; Stierhoff et al., 2009). In the field, proxies for animal growth rates have included measurements of RNA and DNA; otoliths; and weight-specific egg production (e.g., for copepods), which is usually the same as weight-specific somatic growth (Berggreen et al., 1988). Low DO affects movement, metabolism, feeding behavior, and energy intake, all of which depend non-linearly on both DO and temperature (Woods et al., 2022).
Both laboratory and field studies have shown that fish and invertebrate species are often smaller in oxygen-limited waters (Richmond et al., 2006; Casini et al., 2016; Limburg and Casini, 2018). Physiology suggests that growth rate is more sensitive to oxygen than development rate; thus, for crustaceans, animals grow less between molts and are smaller. The environmental oxygen level below which an organism can no longer obtain sufficient oxygen to support normal respiration is often termed the organism's critical oxygen partial pressure, Pcrit (e.g., Fry and Hart, 1948). Respiration rate will be independent of environmental oxygen above Pcrit and will be limited by and proportional to environmental oxygen below Pcrit. The environmental oxygen level below which an organism can no longer obtain sufficient oxygen to support a minimum survivable respiration rate can be thought of as the organism's lethal oxygen partial pressure, Pleth. Below Pleth there will be an increased probability of mortality due to the scarcity of oxygen in the environment. When oxygen levels are <Pcrit for any particular species, a reduction in growth and size is likely to occur. When oxygen levels are <Pleth for that same hypothetical species, it might be replaced by a smaller species with a lower Pleth. Thus, dominance of smaller-sized organisms can occur as the result of oxygen limiting growth among particular cohorts of individuals or through the replacement of large-bodied individuals/species in an area by smaller species over a prolonged period of hypoxia. Warmer temperature can also result in smaller body sizes among fish and invertebrates (e.g., Atkinson, 1994). Thus, lower oxygen frequently interacts with temperature to reduce organism size and can also cause a shift towards smaller-bodied species (Chapelle and Peck, 1999; Gillooly et al., 2001; Rubalcaba et al., 2020; Verberk et al., 2021). For example, smaller copepods have a higher surface-to-volume ratio compared to larger copepods, which favors their oxygen uptake (which occurs through their body surface) over larger copepods in hypoxic waters. In laboratory experiments, Stalder and Marcus (1997) showed that the smaller copepod Acartia tonsa survived low-oxygen conditions better than the larger Labidocera aestiva and Centropages hamatus. In similar types of laboratory experiments, Roman et al. (1993) found that the smaller copepod Oithona colcarva survived low-oxygen conditions better than the larger Acartia tonsa. Small-bodied and sessile benthic taxa are often more hypoxia-tolerant than large-bodied taxa. This can lead to faunal size zonation across oxygen gradients among benthic meio-, macro-, and megafauna, as observed in oxygen minimum zones of the Indian Ocean (Gooday et al., 2009a) and Pacific Ocean (Levin et al., 1991).
The condition factor is calculated from organism length and weight or by direct methods related to lipid content (e.g., Herbinger et al., 1991). The condition factor is a morphometric measurement taken on animals collected inside/outside of hypoxic areas, while estimates for growth require that multiple samples be taken over time. The condition factor of Baltic cod has been related to hypoxia exposure with worsening hypoxia in the last 2 decades, leading to poor condition that along with other trending factors (decreasing food availability) has contributed to a long-term population decline (Casini et al., 2016; Limburg and Casini, 2019). Both the individual growth and the condition factor can be scaled up to the population with sufficient sampling. For example, Eby et al. (2005) assessed low-DO effects on Atlantic croaker in the Neuse River, US, estuary using growth rates estimated from cage experiments in the field and benthic cores used to quantify food availability. They compared summers across 3 years that had different hypoxia conditions and conducted field surveys (feeding, condition, growth) to assess the effects of low DO on juvenile fish growth rate. They used a stage-within-age matrix model to estimate the population-level effects of low DO and found that reduced juvenile growth due to hypoxia also reduced population growth rates. As coastal hypoxia expands, more studies will be needed to understand the effects of low DO on animal growth rates.
2.1.5 Malformation
Low-DO conditions can result in abnormal development of marine organisms. The most sensitive life stages are larvae with malformation by hypoxia confirmed for larval stages of polychaetes, oysters, and fishes in laboratory experiments. For example, larval development of the tubeworm Hydroides elegans was delayed, and more malformed larvae were found in low-DO conditions (Shin et al., 2013, 2014; Leung and McAfee, 2020). High mortality and detrimental effects on development and growth were found in the oyster Crassostrea virginica under hypoxia (Baker and Mann, 1992). Exposure to moderate hypoxia for larval stages of the European sea bass (Dicentrarchus labrax) induced opercular malformation (Cadiz et al., 2018). A subset of market squid (Doryteuthis opalescence) embryos exposed to low DO and low pH exhibited malformations including eye dimorphism and deformities in the mantle and body (Navarro et al., 2016). Malformation caused during early life stages might induce lower survival of larvae through reduced ability to capture food and escape from predators. More research is needed to determine the carryover effects of malformation during larval stages to the later developmental stages. Oxygen demand and food availability are both related to malformation; thus, warmer temperatures and less food availability are important co-stressors.
2.1.6 Mortality
Mortality is the most conspicuous and common metric for hypoxia impact on aquatic organisms and is used in the development of water quality criteria in various coastal systems. Mortality at a particular oxygen level indicates that the organism's metabolic processes cannot be maintained by the ambient oxygen. While animals may tolerate short-term reductions in oxygen, mortality occurs once they deplete their anaerobic coping mechanisms. Immediate mortality may occur during extreme hypoxic events or under anoxic conditions that are accompanied by the release of hydrogen sulfide. Most low-oxygen tolerance measurements for mortality are made under laboratory conditions by manipulating oxygen partial pressure (Vaquer-Sunyer and Duarte, 2008). The lethal oxygen concentration as defined in laboratory experiments is measured over a set time period, usually 24 h. Lethal hypoxia has also been estimated from field measurements with organism presence/absence as a function of oxygen concentration (or partial pressure). Field-based estimates of mortality are less certain because of the temporal and spatial variations in oxygen as well as changes in the vertical/horizontal distribution of the organism due to avoidance of the deoxygenated water. Temperature will affect the assessment of lethal oxygen level because of its influence on oxygen solubility as well as the animals' overall metabolic demands. Thus, if an oxygen concentration is used for estimates of lethal oxygen level, the temperature and salinity conditions should also be reported to allow for the calculation of oxygen partial pressure (Hofmann et al., 2011).
The accurate use of mortality as an indicator of deoxygenation is subject to the characteristics of an organism's life history and habitat. Larval stages with limited oxygen uptake features may have higher lethal oxygen thresholds than juvenile and adult stages. Spawning fish with salinity challenges and feeding cessation may also be more sensitive to low oxygen. Benthic species which cannot swim out of hypoxic zones may have more physiological mechanisms to survive at low oxygen concentrations. Low DO that results in individual mortality can have a range of critical levels that may depend on the age/size of the organism. These variable impacts could be used in assessments of the impact of low oxygen on the mortality rate of populations (Rose et al., 2017b). The lethal limit of oxygen for a particular species can be used for the analysis of available animal habitat (e.g., Brandt et al., 2023) and as a water quality criterion for maintaining the species in particular water bodies (Ekau et al., 2010).
2.1.7 Chemical archives of hypoxia exposure
Dissolved manganese (Mn2+ and Mn3+) becomes more abundant under low-oxygen conditions in marine waters (Trouwborst et al., 2006). Fish otoliths take up the trace element manganese (Mn), and the Mn : Ca ratio in the aragonitic otoliths can reflect the fish's presence in deoxygenated waters (Limburg et al., 2015). The use of Mn : Ca in otoliths as a hypoxia indicator requires knowledge about regional differences in seawater Mn concentrations which can otherwise confound or complicate interpretations of otolith data in fish from different areas. Further complicating the use of this metric of hypoxia exposure is the observation that manganese uptake is also affected by growth rate (Limburg et al., 2015). Another otolith chemical proxy for hypoxia is the ratio of Mn to the trace element magnesium (Mg), which is also taken up in otoliths but is regulated by growth processes (Limburg et al., 2018). These chemical ratios in otoliths were used to infer not only exposure to hypoxia of cod to low-oxygen waters in the Baltic but also physiological stress as indicated by reduced metabolic activity as suggested by lower Mg : Ca (Limburg and Casini, 2019), but see Valenza et al. (2023) for an opposite response in the Gulf of Mexico. Recent analysis of six fish species from three open-ocean OMZs (Namibia, Southern California, and Baja California) revealed a common elemental fingerprint attributed to hypoxia exposure, based on Sr : Ca, Mn : Ca, Ba : Ca, Cu : Ca, and Mg : Ca and distinct from giant sea bass collected in well-oxygenated shallow waters (Cavole et al., 2023). Few tests of invertebrate structures exist; however, Navarro et al. (2016) documented elevated U : Ca in squid statoliths experimentally subjected to low oxygen alone and low oxygen/low pH compared to normoxic conditions.
2.1.8 Metabolic indices
The tolerance to hypoxia decreases with increasing temperature as a result of reduced oxygen solubility and increased animal respiration (Pörtner and Knust, 2007). The relationship of oxygen supply to oxygen demand, called the metabolic index (MI), describes the potential of the environment to support aerobic metabolism relative to basal metabolism (Deutsch et al., 2015). The MI accounts for the non-linear interactions of temperature and oxygen stress in particular organisms. Deutsch et al. (2020) recently updated this metabolic index to account for the effect of species-specific oxygen supply capacity. This modification improves estimates for highly mobile or hypoxia-tolerant species with high oxygen supply capacities. The application of both metabolic indices (Penn et al., 2018; Deutsch et al., 2020) requires information on experimentally derived temperature-dependent low-oxygen thresholds that are not available for most marine species. Yet, these indices can still be applied when experimental data are lacking, using the approach developed by Howard et al. (2020), which is based on the development of different ecophysiotypes. The MI is not an index of biological stress due to low-oxygen waters but rather a predictor of the environment that satisfies the oxygen requirement of a particular species or ecophysiotypes. The MI approach has been used to project species distributions in future warmer oceans (Deutsch et al., 2015); past and future species extinction (Penn et al., 2018); the distribution and size of species in future oceans (Deutsch et al., 2022); and the “climate velocity” of the MI, which predicts how fast and in which direction an organism will need to move in order to survive and maintain its metabolic niche in a future ocean (Parouffe et al., 2023). Clarke et al. (2021) developed a comparable index, called the aerobic growth index (AGI), which integrates growth theory, metabolic theory, and biogeography (Cheung et al., 2013) to create a theoretical oxygen-supply-to-demand ratio. AGI uses oxygen demand at the maintenance metabolic rate, while the metabolic indices (Deutsch et al., 2020; Penn et al., 2018) use oxygen demand at the resting metabolic rate. Note that these forecasts and hindcasts using MI include livable habitat space estimated from temperature and oxygen and not the required food resources or predation pressure. In addition, the forecasts of animal distributions based on MI or AGI currently do not allow for variation in tolerances within species, adaptive responses that take days or weeks to occur, nor do they allow for adaptation to lower oxygen through evolution. Most of the information we have on oxygen tolerance (which forms the basis of MI) is derived from studies that focused on the adult stages of larger organisms. Few if any of these MI forecasts include validation with measurements of animal abundance. One validation used the in situ temperature and oxygen of Chesapeake Bay to predict the bay volume where oxygen supply would exceed oxygen demand for the copepod Acartia tonsa (Roman et al., 2019). Field measurements of copepod distributions verified that A. tonsa abundance was higher in areas of the water column with a positive predicted MI index (Roman et al., 2019).
Field metabolic rates (FMRs) have been estimated for teleost fish by analyzing the δ13C of their otoliths (Chung et al., 2019). The stable isotope composition of C in the aragonite of fish otoliths varies with the isotopic composition of fish blood, which is determined by the dissolved inorganic carbon (DIC) in ambient water and the metabolized carbon released by respiration. Chung et al. (2019) determined that the δ13C of the otoliths of Atlantic cod (Gadus morhua) was related to oxygen consumption in the laboratory. The relationships established were applied to wild cod and other deep-water fish species to infer in situ FMRs (Chung et al., 2019). Jones et al. (2023) applied the FMR approach to assess warming and deoxygenation of the North Sea on both juveniles and adult European plaice (Pleuronectes platessa) in time series in the North Sea between the 1980s and 2000s to show the effect of increasing temperatures on the FMR of the fish. Like other otolith proxies, the FMR was limited to timescales no shorter than approximately 1 month (e.g., Jones et al., 2023). However, recent developments in otolith microchemistry increased the timescale of response to 10 d (Sakamoto et al., 2022), and it possibly extends from weekly to daily for faster growing otolith species like jack mackerel (Muto et al., 2022; Enomoto et al., 2022). Otolith δ13C and δ18O can be measured simultaneously and thus can be used to assess FMRs (carbon ratios) and temperature (oxygen ratios) under model assumptions and known salinity conditions since δ18O of water has a linear relationship with salinity. Additional laboratory work to calibrate the δ13C of otoliths with respiration needs to be conducted for additional fish species including both juveniles and adults over a range of temperatures. Both the MI and the FMR have the potential to be widely used for direct measurements of metabolic rate in fishes, serve as valuable input data to models, and are important tools to assess fish in a future warmer ocean with less oxygen.
2.2 Species/populations
2.2.1 Indicator species
Particular species can be bioindicators of a set of environmental conditions including past and present oxygen state (Schwacke et al., 2012). They may reflect high sensitivity to hypoxia or they may be hypoxia-tolerant species that dominate a system under severe oxygen loss as other species are eliminated. We can also identify indicator taxa (higher-level groupings such as genera or families) whose presence or absence may reflect hypoxia. Ligooxyphiles are low-oxygen specialists, meaning that they are species that select for and thrive in environments with extreme hypoxic conditions (Gallo et al., 2020); their presence and abundance can be used as an indicator of hypoxic conditions. Examples of macroscopic ligooxyphiles include certain types of soft-bodied fishes (e.g., Cherublemma emmelas and Cephalurus cephalus), medusa, and ctenophores.
An indicator species can respond to extremes, duration, or temporal variability in oxygen availability. Loss or increase of sentinel hypoxia indicator species or taxa is likely detected via community inventories as part of time series. Community-based sampling through imagery taken by remote operated vehicles (ROVs) or sampling by trawls, multicores, or grabs can detect these sentinel species. The presence of biogenic materials from sentinel/indicator species (e.g., shells, scales, otoliths, environmental DNA (eDNA), bones) in sediment cores could also be used to examine species response to oxygenation through time (considering changing sedimentation and erosion) and to detect hypoxia indicator taxa (e.g., Moffitt et al., 2015).
Hypoxia tolerance is often associated with organic enrichment as is the case for annelids in the genus Capitella (Rosenberg, 1972), although Capitella cannot tolerate anoxia (Ogino and Toyohara, 2019). Taxa indicative of low-oxygen conditions that are found commonly in suboxic basins (DO <0.1 mL L−1) often include the gastropod Astyris (Allia) permodesta and the oligochaete Olavius crassitunicatus (Levin et al., 2003). In the Gulf of California, the catshark Cephalurus cephalus and the ophidiid Cherublemma emmelas select for areas with suboxic conditions (DO <5 µmol kg−1; Gallo et al., 2018). The mollusks Lucinoma heroica and Dacrydium pacificum and the codlet Bregmaceros bathymaster are also species indicative of the presence of extreme hypoxic conditions in the Gulf of California (Zamorano et al., 2006). Certain species of benthic foraminifera have been used as indicators of low-oxygen conditions in paleo-oceanographic studies (Sen Gupta and Machain-Castillo, 1993). Uvigerina peregrina, for example, is associated with oxygen minimum zones in the Pacific and Arabian Sea (e.g., Moffitt et al., 2014). Members of the genera Globobulimina and Chilostomella can withstand euxinic conditions and can store and respire nitrate (Glud et al., 2009; Piña-Ochoa et al., 2010). The benthic foraminifera, Globobulimina pseudospinescens, Stainforthia fusiformis, and Nonionella turgida, can indicate the presence of anoxic or severely hypoxic conditions in Scandinavian fjords, and these species can survive these conditions by storing and respiring intracellular nitrate (Risgaard-Petersen et al., 2006). For hypoxia-tolerant species that take over a system via successful reproduction, this may be related to life-cycle duration and could take months to years. Long-term seasonal presence of bottom water hypoxia may favor pelagic copepod species, which brood their eggs, as compared to broadcast spawners, whose eggs would sink into anoxic/hypoxic bottom waters. For example, increased eutrophication and low-oxygen bottom waters have resulted in an increase in the abundance of the small, egg-carrying copepod Oithona davisae in Tokyo Bay and a decline in the occurrence of Acartia omorii and Paracalanus sp., copepods that release their eggs into the water column (Uye, 1994).
Indicator species presence may be a straightforward way to detect oxygen changes and is easy to interpret. Hypoxia thresholds have been demonstrated for many taxa (Vaquer-Sunyer and Duarte, 2008), and highly sensitive species have been identified. However, indicator species or taxa may vary regionally as species evolve different oxygen tolerances in different settings or geographic regions that vary in the intensity and temporal variability of hypoxia (Chu and Gale, 2016). Note that a single indicator taxon niche is rarely unidimensional such that multiple indictors can provide a more robust effect of deoxygenation. Mobile species tend to function as hypoxia-sensitive sentinels, whereas sessile taxa may be better tolerant sentinels. A species utility as a sentinel may be determined by accessibility, interest, and response time. If the sentinel species is dominant and lost under increasing hypoxia or if the sentinel species is rare and increasing under hypoxia, it can alter the structure and diversity of communities (or catch). Hypoxia-tolerant and hypoxia-sensitive species may also have different trophic strategies giving rise to food web shifts that accompany the loss or gain of certain indicator taxa.
2.2.2 Disease/parasites
Under low-oxygen conditions, some parasitic and microbial infections can become more common or severe, affecting both individuals and populations. Certain types of pathogenic bacteria start to grow under low-oxygen conditions, increasing organisms' likelihood of exposure (Guo et al., 2022). In addition, low-oxygen effects on host immune responses are common. For instance, low oxygen disrupts endocrine function and can alter organisms' abilities to buffer against parasitic, bacterial, and viral infections at the hormone level (Overstreet, 2021). Reduced hemocyte function and reactive oxygen species production have been found in fish, mollusks, and crustaceans exposed to hypoxia (Mydlarz et al., 2006; Breitburg et al., 2015; Burnett and Burnett, 2022). The exposure to hypoxia can alter individuals' immune responses on timescales of minutes to hours as well as through longer-duration chronic exposure. At the population level, low oxygen can increase the prevalence, mean intensity, and spatial distribution of infections. Understanding disease as an indicator of low-oxygen conditions may be especially important in an aquaculture context as steps can be taken to improve oxygen conditions for the organisms or to move the organisms to well-oxygenated waters. The concentration or partial pressure of oxygen that induces disease is a potentially significant non-lethal oxygen threshold that may be useful in setting water quality goals. However, elevated infection prevalence and intensity can be influenced by a wide variety of factors, including co-occurring stressors such as elevated pCO2. Disease metrics may therefore better serve as indicators of past hypoxia (and the biological changes caused by hypoxia) than as indicators of currently occurring hypoxia.
2.2.3 Behavioral responses
Avoidance is a near-universal response of mobile species to encountering low-oxygen conditions. Tolerances vary among species and life stages, and because species vary in how they respond to near-lethal levels of hypoxia, avoidance behaviors have broad implications for functional habitat availability and can alter spatial and temporal overlap between predators and prey, potential competitors, and conspecifics. Measurable indicators of avoidance of low-oxygen waters can be presence/absence, shallower depths of centers of abundance maxima, and reductions of vertical habitat space.
Zooplankton can change their vertical position in the water column to avoid low-DO bottom waters. In general, depth-stratified zooplankton sampling has shown that copepod abundances are higher in the surface mixed layer and within the pycnocline compared to hypoxic bottom water in coastal environments (Roman et al., 1993, 2012; Keister et al., 2000; Pierson et al., 2009; Keister and Tuttle, 2013). However, the vertical compression of their distribution to the upper water column can increase their vulnerability to predation by visually feeding fish and thus alter food-web processes (e.g., Pothoven et al., 2012; Roman et al., 2012).
Fish are among the most hypoxia-sensitive and most mobile aquatic species that shoal towards the surface, into shallow areas, or away towards open/oxygenated water as oxygen concentrations decline (Eby and Crowder, 2002; Wu, 2002). For example, avoidance of low oxygen has been demonstrated by billfish (Stramma et al., 2011), tuna (Ingham et al., 1977), and sharks (Vedor et al., 2021). Skipjack tuna in particular exhibit an alarm threshold of 3.5 mL L−1 DO, which helps them avoid conditions representing their median tolerance of 2.4–2.8 mL L−1 DO (Ingham et al., 1977). These various behavioral responses to evade hypoxia can lead to habitat compression in which a portion of an organism's range becomes unusable (Kim et al., 2023) and the absence of an organism from where it is normally found. In extreme situations, such an escape response may fail when fish are trapped by land, the entire water column goes hypoxic, and/or fish are encircled by hypoxic water, resulting in fish kills which are among the most conspicuous signs of hypoxia. Few studies have examined the response time of avoidance behaviors of mobile taxa, which appear to vary for sensitive versus tolerant species from minutes to days.
Hypoxia may force organisms into a subpar habitat with fitness consequences, such as striped bass (Morone saxatilis), which are pushed from deeper hypoxic waters into shallows, where they are confronted with thermal stress (Kraus et al., 2015; Itakura et al., 2021). Many shallow-water fish species also utilize aquatic surface respiration, ventilating more highly oxygenated water at the air–water interface. While this avoidance behavior of last resort may enhance survival, there are associated risks of increased vulnerability to aerial and surface predators (Dominici et al., 2012). This phenomenon is apparent in the “jubilees” in Mobile Bay, Alabama, USA (May, 1973), and the “lobster walkouts” in St. Helena Bay, South Africa (Cockcroft, 2001), which are among the most widely recognized of such events and have achieved culturally iconic status. In these cases, commercially important species, including crustaceans and fish, flee hypoxic bottom waters and move into shallows or even onto shore, searching for more-oxygenated water where they are vulnerable to harvest in their lethargic and moribund state. Often mobile organisms emerging from their burrow or crevice habitats become more vulnerable to predation, and so an ancillary indicator of hypoxia may be predators gorging on dead and moribund organisms where those predators are adept at tracking the edge of fluctuating hypoxia areas and/or at making brief forays into hypoxic areas (Seitz et al., 2009). However, these top predators with high mobility are facing a tradeoff between low-oxygen and increased prey availability. In other cases, predators that track more-oxygenated water masses may be able to exploit prey that have done the same, such as tuna species in the mid-latitudes that aggregate in the warm core eddies with high oxygen concentration in the subsurface, which allows them to feed on mesopelagic species for a longer time (Xing et al., 2023).
Soft-bottom infaunal species also exhibit escape responses emerging from burrows and buried positions to the sediment surface to seek higher oxygen (and possibly evading hydrogen sulfide). This includes Nephrops lobsters normally tucked away in burrows that suddenly appear in bottom trawls during hypoxic events, infaunal worms atypically exposed on the sediment surface, and amphipods that extend their tubes above the sediment surface to reach higher into the water columns (Diaz and Rosenberg, 1995).
2.2.4 Population size
A reduction in population size in response to deoxygenation can be the result of reduced reproduction and recruitment, increased mortality as a direct response to oxygen stress or indirect response through less food availability, or increased predation. Depending on the generation time of the species, both short-term episodic deoxygenation and longer-term chronic deoxygenation can reduce population size (Adamack et al., 2017; Roman et al., 2019; Pierson et al., 2022, 2023; Duskey et al., 2023). The limiting and lethal oxygen partial pressure for impacts on the various developmental stages of the species would allow the assessment of the impact of in situ oxygen partial pressures on the population. Limits of this approach include the need to know the Pcrit and Pleth oxygen levels of the various life stages, unknown genetic adaptations to low oxygen, and other abiotic/biotic factors that complicate the interpretations. It usually is not possible to have the oxygen tolerance information for all species of interest, so comparisons/modeling will be necessary to broaden applications to guilds, functional groups, and body size scaling. Impacts of low oxygen are taken into consideration for population models for commercial fisheries and predictions of essential habitat for restoration and protection.
2.2.5 Population growth rate
Population growth rate integrates growth, survival, and reproduction of individuals and expresses the net effect of these vital rates at the population level. Population growth rate therefore reflects multiple pathways of low-DO effects. Population-level growth rate is also the basis of management of harvested species and regulatory actions. Like mortality, measuring population growth rate directly in the field is challenging, but there is a long history of using statistics and modeling to scale population growth from the available data on growth, mortality, and reproduction (Doak et al., 2021).
Population growth rates integrate across effects and life stages and are used for fisheries management and species conservation. Logistic population models have a long history in ecology and directly use population growth rate (r) and carrying capacity (K). Maximum sustainable yield (MSY) is traditionally estimated as one-fourth of K times r. Fisheries stock assessments and population modeling for conservation often use more-complicated matrix projection models with the population divided into classes (age, stage, or size) that use survival, growth, and reproduction rates specific to classes to generate r and K, in addition to other population-level metrics (Doak et al., 2021). The link to hypoxia indicators is how exposure to low DO affects the survival, growth, and reproduction rates, either of the total population or by age class (Rose et al., 2001). Smith and Crowder (2011) used a logistic growth model for blue crabs (Callinectes sapidus) and included hypoxia effects via changes in predation mortality, which affects r and K. Eby et al. (2005) demonstrated how a traditional stage-based matrix model can be used to combine the reduced juvenile stage growth rate due to hypoxia with the finite population growth rate λ, which is equal to er. There are many examples of hypoxia causing reduced habitat availability (e.g., Zhang et al., 2010; Gallo and Levin, 2016; Franco et al., 2022) that can limit the production of a particular life stage within the life history, translating into reduced local productivity (related to r) and reduced carrying capacity. Long et al. (2014) used an age-structured matrix model for the clam Macoma balthica in two regions with varying DO (permanently normoxic and occasionally hypoxic) and found that hypoxia affected mortality via altered predation pressure, fecundity, and maturity. They reported the response of λ as a function of the proportion of the area extent of the hypoxic zone and the duration of the hypoxia.
Low DO has direct and indirect effects that affect survival and reproduction (maturity, fecundity), all of which determine population growth rate, r. There are few examples of direct calculation of the growth rate of the population from field data, but it is more common to use a model to scale these parameters to population growth rates. An example is Eby et al. (2005), who used a stage-within-age matrix projection model and converted low-DO effects on the growth rate of individuals into extended stage duration for juvenile croaker.
2.2.6 Recruitment rate
We use the term recruitment here in the fisheries sense of the number of individuals that survive to the stage or age after which the natural mortality rate is relatively constant. Note that recruitment is also used (often with benthos and some invertebrates) as the number of larvae that settle and enter their sessile stage. Fisheries recruitment as an indicator of low DO is of direct ecological and management relevance as it is a driver of population dynamics and forms the basis of most fisheries management plans. However, examples of empirically based DO effects on fisheries recruitment are rare because recruitment is highly variable, logistically difficult to study, and influenced by many factors and stressors (Houde, 1997), making isolation of the effects of low DO challenging.
Ariyama and Secor (2010) analyzed dredge catch data and showed that the recruitment of gazami crab (Portunus trituberculatus) is related to DO levels. Jung and Houde (2004) examined bay anchovy (Anchoa mitchilli) in Chesapeake Bay and found that recruitment of young of the year (YOY) in October was related to DO concentrations and standing stock biomass in the previous summer. They used anchovy length rather than DO directly as a proxy for low-DO effects on growth in a Ricker spawner-recruitment model. Similarly based on analysis of survey data, Boyer et al. (2001) also implicate hypoxia in the reduction of the northern Benguela sardine (Sardinops sagax) recruitment. Population recruitment is thus a valuable index of deoxygenation that has a direct application to fisheries management.
2.3 Communities/ecosystems
2.3.1 Diversity
Diversity metrics reflect the number of species present and how individuals are distributed among the species. This information represents an aggregated outcome of biotic responses manifesting at the individual and population level that are discussed earlier in this paper. Diversity metrics may include components of species richness, evenness, dominance, rarity, and occasionally trophic traits or a combination of these metrics. Common indices include species richness (S), Shannon–Wiener (H′), Simpson's D, Pielou's J, rarefaction (ESx), Hill numbers (qD), and rank 1 dominance (R1D). Diversity indicator metrics can be applied to counts of individuals categorized by species, family, or even phyla but can also be applied to operational taxonomic units (OTUs) or amplicon sequence variants (ASVs), even when species associated with genetic sequences are not known. Diversity, evenness, and dominance are calculated from count data based on field samples or imagery that are often generated by extensive processing or analysis in the laboratory. Diversity of eukaryotes typically declines with decreasing DO concentration below a threshold that varies with guild and assemblage body size (e.g., mega, macro, meiofauna; Breitburg, 2002; Levin, 2003; Gooday et al., 2010). Examples of species-richness declines under low oxygen exist for many different systems, including bivalves in temperate estuaries (Ducrotoy et al., 2019), corals in tropical reefs (Altieri et al., 2017), benthos and plankton on seamounts (Wishner et al., 1995), demersal fish in oxygen minimum zone regions (Gallo and Levin, 2016), and fauna of continental slopes (Gooday et al., 2010; Hunter et al., 2012). Dramatic diversity declines are often accompanied by declines in evenness and increased dominance by one or a few species (Levin, 2003; Jeffreys et al., 2012; Yasuhara et al., 2012). Dominance by species may reflect high physiological tolerance to low oxygen, better competitive abilities under low oxygen (relative to other species), high food supply, or a combination of these factors.
Diversity thresholds are influenced by the duration of exposure and temporal variability of low-oxygen stress such that diversity responses differ in coastal versus bathyal OMZ settings in different ocean basins and for mobile versus sessile fauna (Levin et al., 2009a; Chu and Gale, 2016; Chu et al., 2019). The diversity (and evenness and dominance) response to oxygen declines or increases has been documented over seasonal cycles, interannually (e.g., ENSO), and over historical and geological timescales (Arntz et al., 2006; Rabalais and Baustian, 2020; Alvarez Zarikian et al., 2022). In east Pacific OMZs where oxygen stress is persistent, diversity thresholds for benthic macroinvertebrates and demersal fish occur around 7 µmol kg−1 DO (Sperling et al., 2016; Gallo et al., 2020). In coastal waters with seasonal hypoxia, diversity thresholds (assumed to be reflected in species thresholds) may average around 63 µmol kg−1 DO, but for crustaceans they can be 25–42 µmol kg−1 DO in the east Pacific and 43–77 µmol kg−1 DO in the Atlantic Ocean (Chu and Gale, 2016).
Advantages of diversity (and evenness and dominance) as an indicator of low-oxygen stress include integration of the response across species with a clear linkage to ecosystem function and health. Changes in dominance are easy to detect via monitoring programs. Thus, when the community shifts to a dominance by a hypoxia-tolerant species, it can be a good indicator of oxygen stress at the ecosystem level. Diversity assessment often requires painstaking inventory of species and counts of individuals, requiring both time and resources. Quantitative multiplex PCR (e.g., Wong et al., 2022) can measure eDNA for several target species, small species, and even cryptic species but is limited for quantitative assessment (e.g., Shelton et al., 2023). Diversity, evenness, and dominance can also be influenced by other factors such as salinity, food availability, or contamination, independently or synergistically with deoxygenation (Rozenzweig and Abramsky, 1993; Levin and Gage, 1998; Piló et al., 2015).
Local (alpha) diversity responses to low-oxygen stress are well documented for benthic metazoan invertebrates (Gooday et al., 2010), benthic foraminifera (Tsujimoto et al., 2006), and demersal fish (Gallo et al., 2020), with the paleo-literature replete with examples for fossil-forming biota (e.g., Tsujimoto et al., 2008; Aberhan and Baumiller, 2003; Yasuhara et al., 2012; Moffit et al., 2014, 2015; Singh et al., 2015). Alpha diversity can be scaled up to gamma diversity across gradients or larger geographic scales. Annelid, nematode, and calcareous foraminifera species show high dominance among benthic sediment fauna subject to severe hypoxia. In extreme OMZs, a single species may comprise 40 %–100 % of the macrofauna (Levin, 2003; Jeffries et al., 2012) and foraminifera (Gooday et al., 2000). Metazoan examples include Linopherus sp. on the Pakistan margin at 800 m (100 % of macrofaunal individuals), Olavius crassitunicatus on the Peru margin (86 %), and Diaphorosoma sp. on the Chile margin (73 %). Protozoan examples include the foraminifera Bolivina seminuda on the Oman margin (43 %) (Gooday et al., 2000). In coastal waters of Chesapeake Bay, paleo-dominance (60 %–90 %) by Ammonia parkinsoniana is associated with hypoxia (Karlsen et al., 2000).
Because hypoxia can favor some species, including invasives, hypoxia may lead to higher regional diversity in an ecosystem, even while suppressing alpha diversity at a given impacted site. Given that recovery of diversity following a hypoxic event may take far longer than the initial decline, the fingerprint of diversity as a hypoxia indicator may be apparent for years or decades, allowing for detection of a hypoxic event long after oxygenated conditions have returned.
A more-mechanistic community-level indicator of the intensity of effects caused by eutrophication-induced hypoxia, focused on species loss, is the effect factor (EF) (Cosme and Hauschild, 2016). EF is designed to evaluate impacts of anthropogenic nitrogen and organic inputs on demersal communities by assessing the fraction of species that will be affected by hypoxia based on their individual thresholds. It requires knowledge of species in the community, their hypoxia sensitivities, their geographic distributions, and environmental conditions. A species sensitivity distribution methodology is used to combine species distribution and lowest-observed-effect concentrations for species to estimate the DO concentration at which half of the community's species are affected. This metric, which extends the concept of diversity to include species sensitivity to hypoxia, can function as a hypoxia stress or ecosystem health index; it has been applied at large spatial scales for five climate zones (Cosme and Hauschild, 2016) and to 66 large marine ecosystems (Cosme et al., 2017). Modifications involving species density distributions have generated additional indices including a potentially affected fraction and potentially disappeared fraction (Cosme et al., 2017).
2.3.2 Taxonomic shifts and ratios
Changing species abundance can lead to taxonomic shifts in community composition, and resulting ratios of specific taxa are sometimes considered hypoxia indicators. Because mobile species can respond quickly to oxygen decline, the resultant shifts in taxonomic composition may offer an early warning of hypoxia. These typically reflect differential tolerance of taxa at the species or higher level. As with other indicators, the intensity, persistence, duration, and temporal sequence of hypoxia will influence taxonomic responses. Taxonomic responses are detected by sampling and counting the entire community or by sampling and counting targeted taxa. Thresholds for taxonomic response differ between seasonally hypoxic/coastal systems and permanently hypoxic systems and with species ontogenetic stage, mobility, and body size.
It is generally thought that larger-bodied animals that can swim will be most sensitive to low-oxygen conditions and will avoid areas of hypoxia when possible. For example, in a meta-analysis of Atlantic species, fish and crustaceans exhibited less hypoxia tolerance (i.e., higher sublethal and lethal thresholds) than priapulids and mollusks (Vaquer-Sunyer and Duarte, 2008), presumably because of their high metabolic demands and their high mobility. In pelagic systems, specific copepod and krill genera specialize in low-oxygen conditions (Wishner et al., 2013; Tremblay et al., 2020). In coastal waters the dominance of gelatinous zooplankton (ctenophores, jellyfish, siphonophores, salps) over crustaceans in hypoxic waters reflects their tolerance to hypoxia (Breitburg et al., 1997; Ekau et al., 2010; Miller and Graham, 2012; Purcell, 2012). Note, however, that cusk eels and cat sharks (Gallo et al., 2018) and tuna crabs (Pineda et al., 2016) at bathyal depths in the eastern Pacific can be extraordinarily abundant at DO concentrations <2 µmol kg−1.
Within OMZs and other hypoxic areas, echinoderms often avoid the lowest oxygen concentrations but form dense bands at OMZ edges (discussed earlier). Sponges with high hypoxia tolerance often replace stony corals (Chu et al., 2019), and annelids and nematodes often dominate over other major taxa in coastal and deep sediments subject to hypoxia (Levin, 2003; Levin et al., 2009a; Rabalais and Basutian, 2020). However, there are locations such as the Namibian shelf where mollusks or crustaceans dominate the infauna at very low oxygen concentrations and the longer-lived, hard-shelled gastropod and bivalve taxa have been proposed as indicators of oxygen change (Zettler et al., 2009, 2013). Among foraminifera, the rotaliids and buliminids with small, thin-walled calcareous tests dominate in severe hypoxia over forams with agglutinated tests (Gooday et al., 2009a). On the Louisiana shelf Pseudononion atlanticum, Epistominella vitrea, and Buliminella morgani have been used as indicators of historically low oxygen in sediment core records (Osterman, 2003). Similarly, some ostracod species (e.g., Bicornucythere bisanensis in Japan; Yasuhara et al., 2007; Loxoconcha sp. in the eastern coast of the USA; Alvarez Zarikian et al., 2022; Cronin and Vann, 2003) have been used as low-oxygen indicators (Yasuhara et al., 2012).
Assessing taxonomic shifts and ratios is an important objective of most long-term ecological time series; however, it can be difficult to assess these indicators across ecosystems due to methodological artifacts and limitations. For instance, the temporal and spatial scales of response by the zooplankton community to low oxygen can be very small and sometimes go undetected due to the coarsely integrated sampling approach of most extended net tows (Wishner et al., 2018). For metazoan meiofaunal communities, the nematode : copepod ratio is often cited as an indicator of contaminant stress (Warwick, 1981) but is also seen to change along oxygen gradients in space and time (Levin et al., 2009a). Nematode counts increase relative to copepod counts as oxygen declines, reflecting the strong tolerance of nematodes to severe hypoxia. The ratio emerges easily from quantitative surveys of meiofaunal taxa but can be time-consuming and difficult to compute when done manually. Changes in nematode : copepod ratios have been observed along OMZ gradients in the eastern Pacific on a seamount off Mexico (Levin et al., 1991) and on the Costa Rica (Neira et al., 2001), Chile (Neira et al., 2001), and Peru (Levin et al., 2002) margins. Interannual changes in nematode dominance are associated with ENSO cycles off Peru and Chile (Gutierrez et al., 2008; Levin et al., 2009a). The ratio affects the next trophic level – potentially selecting for consumers with different food preferences – and can indicate functional change. Among macrofauna, the polychaete to amphipod ratio can reflect changing eutrophication (Dauvin, 2018) and water quality (Maximov and Berezina, 2023) but does not seem to be a good oxygen indicator. For protozoa, the ratio of Ammonia to Elphidium (both benthic foraminifera genera) is a common oxygen/eutrophication proxy, with Ammonia species much more tolerant to hypoxia (Sen Gupta and Machain-Costillo, 1993).
Recovery following hypoxia may follow a predictable pattern of species accumulation and replacements (Lim et al., 2006; Steckbauer et al., 2011), and thus taxonomic characterization of communities in a well-studied system may indicate the timing of a prior hypoxic event. As a consequence of variation among species in their tolerance to hypoxia and their ability to recolonize habitats following a low-oxygen event, community composition will be a product of not only the severity of hypoxia, but also the interval between such events (i.e., persistent, seasonal, episodic, or periodic). In areas where hypoxia is persistent, is frequent, or has recently occurred, we might expect to see the simplest types of communities made up of a limited number of hypoxia-tolerant and/or opportunistic species. While hypoxia is typically thought of as an agent of species elimination, it can have positive effects at the local scale on the presence or abundance of some animals, by freeing up resources through elimination of competitively dominant species or by excluding less-tolerant predators and creating a predation refuge for more-hypoxia-tolerant prey. Moreover, these potential benefits can extend to invasive species, and as a consequence, hypoxia may lead to higher beta or gamma diversity in an ecosystem, even while suppressing alpha diversity at a given impacted site.
2.3.3 Abundance and biomass
Changes to community-level measures of abundance and biomass can occur in response to hypoxia. Animal abundance and biomass collections are typically made using community-sampling methods, including trawls, sediment cores, net tows or pumps, and visual surveys, or are quantified from video and still imaging by ROVs, submersibles, photo sleds, or autonomous landers. Changes in these indicators can be seen when sampling across oxygen gradients in space or in a time series as abundance and biomass respond to oxygen changes seasonally, as well as over interannual, historical, and geologic timescales (Seitz et al., 2009; Moffitt et al., 2014, 2015). Species abundance and biomass reflect important biological processes such as recruitment, growth, avoidance, mortality, and local extinctions. These indicators broadly integrate responses across species and changes related to the productivity of a system, which is important from a fisheries and ecosystem management perspective (Breitburg, 2002). However, because abundance and biomass are closely linked to productivity and food availability, they are often confounded with nutrient input and eutrophication, which gives rise to opposing responses (i.e., abundance will increase with eutrophication but decrease with deoxygenation; Breitburg et al., 2009).
The abundance of vulnerable taxa or size groups will typically decrease once oxygen levels fall below a certain oxygen threshold, and they may first rapidly increase to higher-than-baseline levels prior to or at this threshold due to edge effects (Wishner et al., 1995, 2013; Levin, 2003; Yasuhara et al., 2007, 2012; Gooday et al., 2010). Edge effects (a) can result from different taxa aggregating in a smaller area due to avoidance of a hypoxic zone as described for certain fisheries species in the Gulf of Mexico (Craig, 2012); (b) can result from plentiful food (e.g., phytodetritus and meiofauna) combined with the absence of predators in a specific oxygen zone as described for OMZs (Gallo and Levin, 2016); or (c) can be related to dominant taxa that thrive at high abundances at a specific low-oxygen threshold, for example, ophiuroids bands that form on seamounts and continental margins (Levin, 2003), cusk eels in Narragansett Bay (Hale et al., 2016), and the cusk eel Cherublemma emmelas in the Gulf of California (Gallo et al., 2018, 2020).
The degree of abundance and biomass limitation due to low DO is dependent on the severity, extent, and duration of hypoxia. Oxygen thresholds are taxon-specific, body-size-specific, and region-specific. For example, on the US Pacific Coast (Keller et al., 2015) and off Peru (Rosenberg et al., 1983), the catch per unit effort (CPUE) of demersal fish decreases below a specific oxygen threshold; however, the thresholds differ between the two systems, with the oxygen threshold being lower off the coast of Peru. DO has also been shown to be an important covariate in explaining demersal fish CPUE in Chesapeake Bay (Bucheister et al., 2013). However, in general, fish landings can be poor indicators of hypoxia due to the effects of shoaling and aggregation (Rose et al., 2019; Chesney et al., 2000).
At the community level, changes in animal density can occur at a higher oxygen threshold than that required to see changes in species richness or diversity. For demersal fish in the Gulf of California, for instance, DO 3±1 µmol kg−1 was identified as the threshold below which fish density decreased (Gallo et al., 2020), compared to a DO threshold of 7 µmol kg−1 for diversity (H′). Reductions in abundance of sensitive, less-mobile fish species may occur due to fish kills (Thronson and Quigg, 2008). Effects may be direct via increased mortality, through prolonged exposure to low DO (Breitburg et al., 1999; Turner, 2001; Diaz and Breitburg, 2009), or indirect, via reduction of habitat availability in the benthos (Turner, 2001) and water column (Breitburg et al., 1999; Chesney et al., 2000; Turner, 2001) or through alterations to food web structure (Miller and Graham, 2012). The general observation of reduced coastal copepod abundances in water columns with hypoxic bottom waters (Roman et al., 1993; Keister et al., 2000; Kimmel et al., 2012) suggests they have lower population growth and greater mortality, predation, and/or emigration.
2.3.4 Functional shifts
In areas subject to episodic or seasonal hypoxia, infaunal animals may exhibit changes in dwelling habit and depth within sediments. Behavioral responses to hypoxia include tube lengthening or body extension into the water column by polychaetes and amphipods, shallower burial, emergence from sediment, or aggregate formation to raise animals up into the water column (reviewed in Diaz and Rosenberg, 1995; Levin et al., 2009a). Although these are rarely monitored as indicators, they generally reflect oxygen declines. These changes along with replacement of large, deep dwellers and suspension feeders by taxa that are smaller, near-surface dwelling, and surface-deposit-feeding lead to declines in bioturbation and bioirrigation under hypoxia (Diaz and Rosenberg, 1995; Middelburg and Levin, 2009). Metrics that reflect these changes include sediment mixed layer depth, burrow size and diversity, and bioturbation rate (Db), although the latter metric is not always positively correlated with oxygen concentration (Smith et al., 2000). Under persistent, stable hypoxia some tolerant species deepen their vertical distributions as long as some oxygen is present (Levin et al., 2009a). In low sedimentation areas, hypoxia-induced changes in sediment mixing can lead to reduced organic matter decomposition and enhanced carbon preservation (Canfield, 1994).
Thresholds for the changes outlined above often occur around 2 mL L−1 DO for shallow-water taxa and at 0.4 mL L−1 DO (or less) for OMZ species. Sediments on the Pakistan margin shift from laminated (no bioturbation) to fully bioturbated across gradients from DO 0.1 to 0.2 mL L−1 (Levin et al., 2009b). Because nutrient and organic matter additions often drive oxygen depletion, the hypoxia indicators described above also reflect organic matter enrichment (Pearson, 1978). Other functional changes can include altered rates of colonization (DO between 0.05 and 0.5 mL L−1; Levin et al., 2013) and altered carbon cycling pathways (Woulds et al., 2007), with protozoans dominating carbon uptake over metazoans under severe hypoxia. These functional responses tend to occur on seasonal or longer timescales and may therefore prove most useful as an indicator of long-term oxygen loss.
2.3.5 Food web structure
Deoxygenation can result in changes in the presence, abundance, and behavior of interacting species in marine food webs. The severity and distribution of oxygen concentrations (or partial pressures) can affect relevant behaviors and alter encounter rates. As discussed previously, the presence, distribution, and behaviors of individual species can change in response to both oxygen distribution and its effects on organisms at low levels. Under low-oxygen conditions, tolerant species can become more dominant throughout the food web because their predatory strategies are less affected and/or escape behavior is less impaired relative to other species with which they interact (Breitburg et al., 1997, 1999). Certain feeding modes such as carnivory can become less common or even absent under severely hypoxic conditions (Sperling et al., 2013). Food chain length can also be impacted by hypoxia, becoming longer and supporting fewer top predators, with more energy flow dominated by microbial pathways (such as a shift from carbon fixation to chemosynthesis). This change in food web structure results in less trophic transfer upward and the presence of conspicuous microbial mats that are themselves an indicator of hypoxic conditions (Levin, 2003; Woulds et al., 2007; Levin et al., 2009a). This relationship between food chain length and low oxygen was apparent during the early Cenozoic warm period (50 Mya), when the warmer, less-oxygenated ocean supported longer food chains and a lower abundance of top predators (Norris et al., 2013).
Shifts in the distribution of species from an area subject to oxygen loss to areas nearby (where oxygen levels may vary) could alter surrounding food webs. Certain ecological guilds that were once underrepresented may become more abundant in an ecosystem as they escape deoxygenation elsewhere or track prey that have migrated to find better-oxygenated waters.
As with other metrics, thresholds for trophic changes will depend on differences in tolerance of the various interacting species. Tolerance thresholds will vary among species (e.g., finfish versus gelatinous zooplankton), habitats (e.g., estuaries vs. OMZs), geography, and temperatures. In estuaries, gelatinous zooplankton (scyphozoans and ctenophores) tend to be more tolerant of low-oxygen exposure than their copepod and larval fish prey and more tolerant than juvenile or adult fishes competing for the same prey (Breitburg et al., 1997). Conversely, sessile or relatively sedentary organisms are often more hypoxia-tolerant than their predators and may gain a refuge. This is the case for estuarine hard clams that reach their highest abundance in low-DO areas where their predators are excluded (Altieri, 2008) and in kelp forests where hypoxia reduces grazing pressure, thereby increasing kelp survival (Ng and Micheli, 2020).
Shifts in consumer interactions associated with hypoxia result in altered food web structure and trophic function. Lower proportions of carnivory in the polychaete community have been found under low-oxygen conditions (Sperling et al., 2013). Under hypoxic conditions demersal fish on continental slopes shift from feeding in the water column (on vertically migrating zooplankton and fish) to consuming largely benthic prey, yielding longer, less-efficient food chains (Gallo, 2018). Hypoxia-induced changes in food webs may result from changes in the abundances of some species and/or the distributional overlap of predators and prey (Breitburg et al., 1997; Ekau et al., 2010). Diets of fishes can differ in hypoxic water as shown for Atlantic bumper (Chloroscombrus chrysurus) in the Gulf of Mexico (Glaspie et al., 2018). Some fishes may even benefit from hypoxia if their prey are forced into more-vulnerable predatory habitats as suggested for Chesapeake Bay, where striped bass may benefit from the concentration of bay anchovy prey in the well-oxygenated mixed layer (Costantini et al., 2008). Whether the effects of hypoxia on fish populations are positive or negative is likely to be species-specific and ecosystem-dependent (Breitburg et al., 1997; Breitburg, 2002; Costantini et al., 2008) and also dependent on the severity of low oxygen coupled to the prevailing temperatures and the relative tolerances of predators and prey.
Shifts in trophic structure are detected with field measures of encounter rates, gut content analysis, and stable isotopes that detect changes in the base of the food chain (δ13C) or trophic level (δ15N). Models such as ECOPATH/ECOSIM (Christensen and Walters, 2004) are useful to combine field and experimental data to achieve a more-comprehensive understanding of changes to food webs (e.g., de Mutsert et al., 2017). Similarly, biomass, abundance, or catch trophic spectra can offer a high-level indicator of changes to food webs (e.g., Gascuel et al., 2005).
There are some indices that incorporate elements of diversity and trophic function such as the infaunal trophic index (ITI) (Word, 1980), AZTI's marine biotic index (AMBI) (Caswell et al., 2019), and the Trophic State Index for Benthic Invertebrates (TSI-BI) (Chalar et al., 2011). These have been applied over a range of sediment ecosystems and over extended periods of time to examine change, including in response to oxygen loss.
Trophic indicators offer a holistic measure that synthesizes a variety of responses of individual species. As with many indicators discussed here, time and resources are required for fieldwork and experiments. Changes in food web structure can be driven by other co-occurring environmental stressors in addition to hypoxia, such as changes in nutrient input, introduction of an invasive species, and species distribution changes due to climate change.
3.1 Scaling of indicators
Scaling of indicators is often necessary to enable the observed values of the indicator to be interpreted as representing the state of the system and for results to be expressed on spatial and temporal scales that are ecologically or societally meaningful. Consideration of what types of scaling and to what extent scaling is needed is important when selecting an indicator, designing a sampling plan, and interpreting and communicating the results of an indicator. Scaling often determines what species and life stages to measure, the specific indicator(s) needed, and how to allocate effort to sampling locations and frequency of sampling.
Scaling can employ graphical or statistical analyses to extrapolate the measured conditions of individuals to broader areas than those locations sampled (e.g., subregions, basin-wide) or to more-generalized timescales than those captured by the data (e.g., month, season, years). This scaling employs the statistical concept of looking for patterns in the data collected at different locations and/or over time and subsequently making key assumptions about how these data reflect broader conditions to infer the population of indicator values.
Another type of scaling analysis is used with indicators to derive a mechanistic understanding of how the indicator logically and causally relates to higher levels of biological organization (population, community, or ecosystem). For example, low-DO impaired vision affects detecting prey, which determines feeding and growth, which affects vulnerability to predator (mortality) and fecundity, which affects population abundance. This integration and scaling across levels of biological organization from the organismal to ecosystem level can be represented in a conceptual diagram (Altieri and Witman, 2006), where low-oxygen stress reduced survivorship and growth of individual mussels and impacted the density and spatial extent of mussel populations. Individuals of a single species could be used to infer the state of the population, while observations on multiple species can be leveraged to community (e.g., diversity) and food web levels (e.g., energy pathways). The condition of individuals as indicated by lipid content (e.g., Herbinger et al., 1991) suggests sufficient exposure to low DO can elicit a response of the bioenergetics and physiology of the individual. Reduced animal condition can be related to the oxygen state of the system and can lead to higher mortality, lowered fecundity, and other responses that can be directly related to population, community, or food web levels. While values of indicators on subsets of individuals can stand alone to show exposure and responses of individuals, scaling translates indicator observations into potentially more relevant levels of biological organization and scales of time and space. This mechanistic scaling approach was used by Rose et al. (2017a, b) to examine how reduced growth, increased mortality, and reduced fecundity due to low-DO exposure affected croaker (Micropogonias undulatus) population dynamics in the Gulf of Mexico. By using an agent-based model with a 2-D grid that included dynamic DO field, the time-dependent exposures of individuals were simulated and avoidance behavior was projected.
Scaling of indicators can include numerical models which provide a quantitative translation of the indicator into variables that are more relevant to management and society. Common situations requiring such modeling are when multiple stressors covary and DO effects need to be isolated or when expressing indicators in units explicitly chosen to inform policy (e.g., economic impacts of reduced biodiversity) and management decisions (e.g., fisheries yield). For instance, Franco et al. (2022) scaled low-DO effects to habitat changes of Pacific halibut (Hippoglossus stenolepis) in the northeastern Pacific. They used fisheries-independent data and model predictions of oxygen, and a metabolic index was used to map suitable aerobic habitat.
3.2 Application of indicators
The suite of indicators discussed differ in the temporal and spatial scales of oxygen influence, which are reflected in the types of settings where it is most applicable, in possible confounding factors, and in the expertise and resources required for application. These differences are summarized in Table 1. All of these aspects together influence the potential applications of the different indicators of low-oxygen stress.
Table 1Summary considerations for application of hypoxia indicators including settings, confounding factors, expertise, resource requirements, and utility.
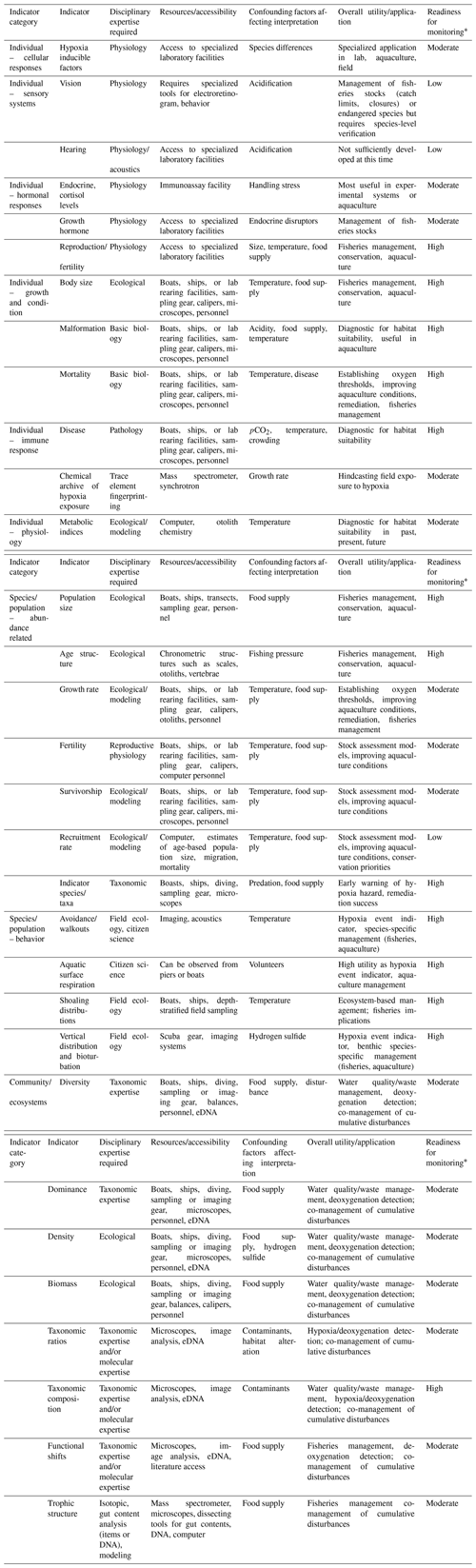
* Readiness based on combined criteria: conceptual foundation, feasibility of implementation, response variability, interpretation, and utility (Jackson et al., 2000; EPA 620-R-99/005).
Fisheries and aquaculture. Coastal areas where natural and anthropogenic nutrient inputs can result in deoxygenation of bottom waters include regions of enhanced fisheries and aquaculture (Nixon and Buckley, 2002; Breitburg et al., 2018; Zhan et al., 2023). While deoxygenation impacts on fisheries catch can be difficult to ascertain because of fish movement, adaptations, and changes in fishing effort and techniques, laboratory experiments have demonstrated deleterious impacts on species that are commercially harvested (Roman et al., 2019; Rose et al., 2017b; Laffoley and Baxter, 2019; Zhan et al., 2023). Deoxygenation impacts on fisheries may be most impactful on artisanal fisheries and aquaculture facilities, which often have little capacity to relocate as hypoxia grows in space and time. Aquaculture can contribute to deoxygenation through the organic input to bottom waters (Rice, 2015), and animals restrained in nets and cages are unable to escape harmful oxygen conditions. Research is needed to develop aquaculture species with strong hypoxia tolerance and economic potential (see Zhan et al., 2023).
Water quality management. Environmental management agencies in many countries have established goals to protect and expand essential habitats for aquatic organisms using oxygen concentration as an indicator to estimate in situ physiological stress. In Chesapeake Bay, USA, this approach has been taken to develop estimated habitat space for different animal groups based on oxygen concentrations and low-oxygen tolerance (e.g., Batiuk et al., 2009; Zhang et al., 2018b). In the Gulf of Mexico, nutrient management targets aim to reduce the area of the hypoxic zone to achieve similar habitat improvements (Scavia and Donnely, 2007). The Baltic Marine Environment Protection Commission (HELCOM) considers “oxygen debt” as a metric for ecosystem health (e.g., Stoicescu et al., 2019). This is consistent with the EU Marine Strategy Framework Directive aimed at improving marine waters, which considers the concentration of oxygen in near-bottom waters as an indicator (Friedland et al., 2021). In all of these cases, low-oxygen conditions are prioritized because they are considered to have negative consequences for a broad range of harvestable marine organisms as well as their pelagic and benthic prey. Generally, these minimum oxygen concentrations are based on mortality estimates and sometimes sublethal effects. The partial pressure of oxygen rather than oxygen concentration may be the more-relevant measure of DO availability (Hofmann et al., 2011) because it integrates the effects of temperature and salinity on oxygen availability.
Climate and the carbon cycle. Biotic changes associated with deoxygenation can alter processes of carbon and nutrient mixing, remineralization, nitrification and denitrification, carbon transport, accumulation and sequestration, and climate feedbacks from nitrous oxide or methane release (Breitburg et al., 2018). The use of biotic indicators can identify times, places, or conditions where these climate-relevant changes to the carbon or nutrient cycles or feedbacks may occur. The Intergovernmental Panel on Climate Change (IPCC) reports and World Ocean Assessments mention oxygen in the context of climate change far more than the Intergovernmental Science-Policy Platform on Biodiversity and Ecosystem Services (IPBES), but even these suffer from low confidence of oxygen observations or models and limited to no attention in summaries for policymakers (Levin et al., 2023). Ideally, oxygen sensitivity and indicators discussed in this review could become a central part of climate change and biodiversity policy, for example, in the United Nations Framework Convention on Climate Change (UNFCCC) global stock take, Ocean Dialogue, nationally determined contributions and national adaptation plans, and the research and systematic observation discussions in UNFCCC Subsidiary Body for Scientific and Technological Advice (SBSTA).
Biodiversity and conservation. Deoxygenation is recognized as a threat to biodiversity, particularly for larger taxa or those adapted to highly oxygenated waters. However, the extent to which deoxygenation is recognized and addressed in global assessments of marine threats and in policies that conserve biodiversity varies greatly. The Convention on Biological Diversity Framework 2030 does not mention oxygen but is developing diversity indicators that could readily incorporate oxygen-sensitive taxa or guilds (Hughes and Grumbine, 2023). The new Biodiversity Beyond National Jurisdiction (BBNJ) Agreement to protect biodiversity and enable its sustainable use in international waters mentions deoxygenation only in its preamble and in the context of types of capacity development and technology transfer, but climate and specifically oxygen vulnerability is not included in a list of criteria for marine protected areas or environmental impact assessment. Projected changes in oxygen availability and habitat suitability for sensitive species can be applied to the designation of protected areas, fisheries regulations, and evaluation of cumulative impacts in environmental impact assessment (Dunn et al., 2018; Levine et al., 2023).
Tourism, recreation, and other livelihoods. Biological indicators of low oxygen in coral reefs or recreational fishing habitats as well as mass mortality events washing up on beaches represent important sentinels of oxygen effects on tourism and recreation. Having early warning signs of impending or existing hypoxic events can permit various forms of adaptation among those dependent on a healthy ocean, resilient fish populations, and clean beaches for income.
3.3 Research needs and opportunities
Recent reviews on deoxygenation have suggested research needs to improve our understanding and prediction of impacts on marine organisms (e.g., Breitburg et al., 2018; Woods et al., 2022; Zhan et al., 2023). There needs to be more research on the impacts of low oxygen and relevant stressors (especially temperature) on the various life stages of commercially harvested species. Studies on important commercial and keystone species should include the relevant timescales to assess the impacts of episodic, seasonal, annual, and interannual fluctuations of low-DO waters on individuals and populations. Research on the impacts of low-oxygen waters on marine organisms should include more studies that integrate the effects that cascade through the organism to population, community and ecosystem levels. Similarly, when addressing the impacts of deoxygenation on a commercially harvested species, low DO should be included in an ecosystem-based management (EBM) approach that includes predators, prey, and human influences. Investigators need to consider that oxygen concentration alone is not a predictor of organisms' fitness. While oxygen partial pressure is the relevant physiology measure, knowledge of exposure histories, life-stage sensitivity, and cumulative stressors is essential for holistic understanding.
Opportunities for national and international research on the impacts of deoxygenation include the Intergovernmental Oceanographic Commission of UNESCO's (IOC-UNESCO) Global Ocean Oxygen Network (GO2NE), United Nations Decade of Ocean Science for Sustainable Development program, and Global Ocean Oxygen Decade (GOOD). GO2NE is committed to providing a global and multidisciplinary view of deoxygenation, with a focus on understanding the multiple aspects and impacts. From 2021–2030 the Ocean Decade program GOOD will raise global awareness about ocean deoxygenation, provide knowledge for action, and develop mitigation and adaptation strategies to ensure continued provision of ecosystem services and minimize impacts on the ocean economy through local, regional, and global efforts. A GOOD programmatic focus on development and application of biological indicators of hypoxia, possibly tied to the Global Ocean Oxygen Database (GO2DAT; Grégoire et al., 2021), would facilitate the integration of deoxygenation more broadly into ocean management. Many of the biological indicators of oxygen stress described in this paper, if tied to specific DO response thresholds and in situ oxygen concentrations, can be used in monitoring and applied to management of water quality, biodiversity, and fisheries.
3.4 A global endeavor: challenges for equitable application of indicators
The ability to apply oxygen indicators across the global ocean in both coastal waters and open-ocean waters will depend on (a) improved oxygen literacy across various stakeholders, including managers, funders, and academics; (b) expanded technical capacity, such as instrumentation, associated infrastructure, and technical expertise; (c) improved data access according to Findability, Accessibility, Interoperability and Reusability (FAIR) implementation principles (Jacobsen et al., 2020); and (d) inclusive training and empowerment of the next generation of scientists and practitioners. Each of these represents a challenge that can be addressed by different elements of the Global Ocean Oxygen Decade program and other regional and international networks. One goal would be to enable small island developing states, the least-developed countries, and the global south more generally to join wealthier nations in having the knowledge, instruments, funding, and expertise to apply oxygen indicators for science and management. Training opportunities such as the recent summer schools generated by GO2NE represent a valuable mechanism for achieving these goals.
Data included in the paper can be found in the referenced citations.
MRR and LAL contributed equally to the concept development and led the writing of the paper. The other authors contributed essential scientific sections of the paper.
The contact author has declared that none of the authors has any competing interests.
Publisher’s note: Copernicus Publications remains neutral with regard to jurisdictional claims made in the text, published maps, institutional affiliations, or any other geographical representation in this paper. While Copernicus Publications makes every effort to include appropriate place names, the final responsibility lies with the authors.
We thank the Global Ocean Oxygen Network (GO2NE) expert working group of the Intergovernmental Oceanographic Commission of UNESCO (IOC-UNESCO) and its secretariat for encouraging this work. We greatly appreciate the helpful reviews by Jackson Chu and an anonymous reviewer, whose suggestions improved the clarity and content of the paper.
This paper was edited by Marilaure Grégoire and reviewed by Jackson Chu and one anonymous referee.
Aberhan, M. and Baumiller, T. K.: Selective extinction among Early Jurassic bivalves: a consequence of anoxia, Geology, 31, 1077–1080, https://doi.org/10.1130/G19938.1, 2003.
Adamack, A. T., Rose, K. A., and Cerco, C. F.: Simulating the Effects of Nutrient Loading Rates and Hypoxia on Bay Anchovy in Chesapeake Bay Using Coupled Hydrodynamic, Water Quality, and Individual-Based Fish Models, Modeling Coastal Hypoxia, Springer International Publishing, 319–357, https://doi.org/10.1007/978-3-319-54571-4_12, 2017.
Affatati, A., Scaini, C., and Salon, S.: Ocean sound propagation in a changing climate: Global sound speed changes and identification of acoustic hotspots, Earth's Future, 10, e2021EF002099, 2022.
Alderdice, R., Suggett, D. J., Cárdenas, A., Hughes, D. J., Kühl, M., Pernice, M., and Voolstra, C. R.: Divergent expression of hypoxia response systems under deoxygenation in reef-forming corals aligns with bleaching susceptibility, Glob. Change Biol., 27, 312–326, https://doi.org/10.1111/gcb.15436, 2020.
Alderdice, R., Perna, G., Cárdenas, A., Hume, B. C. C., Wolf, M., Kühl, M., Pernice, M., Suggett, D. J., and Voolstra, C. R.: Deoxygenation lowers the thermal threshold of coral bleaching, Sci. Rep., 12, 18273–18273, https://doi.org/10.1038/s41598-022-22604-3, 2022.
Altieri, A. H.: Dead zones enhance key fisheries species by providing predation refuge, Ecology, 89, 2808–2818, https://doi.org/10.1890/07-0994.1, 2008.
Altieri, A. H. and Witman, J. D.: Local extinction of a foundation species in a hypoxic estuary: integrating individuals to ecosystem, Ecology, 87, 717–730, https://doi.org/10.1890/05-0226, 2006.
Altieri, A. H., Harrison, S. B., Seemann, J., Collin, R., Diaz, R. J., and Knowlton, N.: Tropical dead zones and mass mortalities on coral reefs, P. Natl. Acad. Sci. USA, 114, 3660–3665, https://doi.org/10.1073/pnas.1621517114, 2017.
Alvarez Zarikian, C. A., Nadiri, C., Alonso-García, M., Rodrigues, T., Huang, H.-H. M., Lindhorst, S., Kunkelova, T., Kroon, D., Betzler, C., and Yasuhara, M.: Ostracod response to monsoon and OMZ variability over the past 1.2 Myr, Mar. Micropaleontol., 174, 102105, https://doi.org/10.1016/j.marmicro.2022.102105, 2022.
Ambler, J. W.: Seasonal factors affecting egg production and viability of eggs of Acartia tonsa Dana from East Lagoon, Galveston, Texas, Estuar. Coast. Shelf Sci., 20, 743–760, https://doi.org/10.1016/0272-7714(85)90030-7, 1985.
Ariyama, H. and Secor, D. H.: Effect of environmental factors, especially hypoxia and typhoons, on recruitment of the Gazami crab Portunus trituberculatus in Osaka Bay, Japan, Fisheries Sci., 76, 315–324, https://doi.org/10.1007/s12562-009-0198-6, 2010.
Arntz, W. E., Gallardo, V. A., Gutiérrez, D., Isla, E., Levin, L. A., Mendo, J., Neira, C., Rowe, G. T., Tarazona, J., and Wolff, M.: El Niño and similar perturbation effects on the benthos of the Humboldt, California, and Benguela Current upwelling ecosystems, Adv. Geosci., 6, 243–265, https://doi.org/10.5194/adgeo-6-243-2006, 2006.
Atkinson, D.: Temperature and Organism Size–A Biological Law for Ectotherms?, Adv. Ecol. Res., 25, 1–58, https://doi.org/10.1016/s0065-2504(08)60212-3, 1994.
Baker, S. M. and Mann, R.: Effects of Hypoxia and Anoxia on Larval Settlement, Juvenile Growth, and Juvenile Survival of the Oyster Crassostrea virginica, Biol. Bull., 182, 265–269, https://doi.org/10.2307/1542120, 1992.
Batiuk, R. A., Breitburg, D. L., Diaz, R. J., Cronin, T. M., Secor, D. H., and Thursby, G.: Derivation of habitat-specific dissolved oxygen criteria for Chesapeake Bay and its tidal tributaries, J. Exp. Mar. Biol. Ecol., 381, S204–S215, https://doi.org/10.1016/j.jembe.2009.07.023, 2009.
Berggreen, U., Hansen, B., and Kiørboe, T.: Food size spectra, ingestion and growth of the copepod Acartia tonsa during development: Implications for determination of copepod production, Mar. Biol., 99, 341–352, https://doi.org/10.1007/bf02112126, 1988.
Boyer, D. C., Boyer, H. J., Fossen, I., and Kreiner, A.: Changes in abundance of the northern Benguela sardine stock during the decade 1990–2000, with comments on the relative importance of fishing and the environment, S. Afr. J. Marine Sci., 23, 67–84, https://doi.org/10.2989/025776101784528854, 2001.
Brandt, S., Kolesar, S., Glaspie, C., Laurent, A., Sellinger, C., Pierson, J., Roman, M., and Boicourt, W.: Functional Seascapes: Understanding the Consequences of Hypoxia and Spatial Patterning in Pelagic Ecosystems, Oceanography, 36, 28–30, https://doi.org/10.5670/oceanog.2023.s1.8, 2023.
Breitburg, D.: Effects of hypoxia, and the balance between hypoxia and enrichment, on coastal fishes and fisheries, Estuaries, 25, 767–781, https://doi.org/10.1007/bf02804904, 2002.
Breitburg, D., Rose, K., and Cowan, J.: Linking water quality to larval survival:predation mortality of fish larvae in an oxygen-stratified water column, Mar. Ecol. Prog. Ser., 178, 39–54, https://doi.org/10.3354/meps178039, 1999.
Breitburg, D., Levin, L. A., Oschlies, A., Grégoire, M., Chavez, F. P., Conley, D. J., Garçon, V., Gilbert, D., Gutiérrez, D., Isensee, K., Jacinto, G. S., Limburg, K. E., Montes, I., Naqvi, S. W. A., Pitcher, G. C., Rabalais, N. N., Roman, M. R., Rose, K. A., Seibel, B. A., Telszewski, M., Yasuhara, M., and Zhang, J.: Declining oxygen in the global ocean and coastal waters, Science, 359, 46, https://doi.org/10.1126/science.aam7240, 2018.
Breitburg, D. L., Loher, T., Pacey, C. A., and Gerstein, A.: Varying effects of low dissolved oxygen on trophic interactions in an estuarine food web, Ecol. Monogr., 67, 489–507, https://doi.org/10.1890/0012-9615(1997)067[0489:veoldo]2.0.co;2, 1997.
Breitburg, D. L., Hondorp, D. W., Davias, L. A., and Diaz, R. J.: Hypoxia, Nitrogen, and Fisheries: Integrating Effects Across Local and Global Landscapes, Annu. Rev. Mar. Sci., 1, 329–349, https://doi.org/10.1146/annurev.marine.010908.163754, 2009.
Breitburg, D. L, Baumann, H., Sokolova, I. M., and Frieder, C. A.: Multiple stressors-forces that combine to worsen deoxygenation and its effects, in: Ocean deoxygenation: Everyone's problem Causes, impacts, consequences and solutions, International Union for Conservation of Nature and Natural Resources, Gland, Switzerland, 225–247, ISBN 978-2-8317-2013-5, 2019.
Buchheister, A., Bonzek, C., Gartland, J., and Latour, R.: Patterns and drivers of the demersal fish community of Chesapeake Bay, Mar. Ecol. Prog. Ser., 481, 161–180, https://doi.org/10.3354/meps10253, 2013.
Burnett, K. G. and Burnett, L. E.: Immune Defense in Hypoxic Waters: Impacts of CO2 Acidification, Biol. Bull., 243, 120–133, https://doi.org/10.1086/721322, 2022.
Cadiz, L., Zambonino-Infante, J.-L., Quazuguel, P., Madec, L., Le Delliou, H., and Mazurais, D.: Metabolic response to hypoxia in European sea bass (Dicentrarchus labrax) displays developmental plasticity, Comp. Biochem. Phys. B, 215, 1–9, https://doi.org/10.1016/j.cbpb.2017.09.005, 2018.
Canfield, D. E.: Factors influencing organic carbon preservation in marine sediments, Chem. Geol., 114, 315–322, https://doi.org/10.1016/0009-2541(94)90061, 1994.
Casini, M., Käll, F., Hansson, M., Plikshs, M., Baranova, T., Karlsson, O., Lundström, K., Neuenfeldt, S., Gårdmark, A., and Hjelm, J.: Hypoxic areas, density-dependence and food limitation drive the body condition of a heavily exploited marine fish predator, R Soc. Open Sci., 3, 160416–160416, https://doi.org/10.1098/rsos.160416, 2016.
Caswell, B. A., Frid, C. L. J., and Borja, A.: An ecological status indicator for all time: Are AMBI and M-AMBI effective indicators of change in deep time?, Mar. Pollut. Bull., 140, 472–484, https://doi.org/10.1016/j.marpolbul.2019.01.068, 2019.
Cavole, L. M., Limburg, K. E., Gallo, N. D., Vea Salvanes, A. G., Ramírez-Valdez, A., Levin, L. A., Oropeza, O. A., Hertwig, A., Liu, M.-C., and McKeegan, K. D.: Otoliths of marine fishes record evidence of low oxygen, temperature and pH conditions of deep Oxygen Minimum Zones, Deep-Sea Res. Pt. I, 191, 103941, https://doi.org/10.1016/j.dsr.2022.103941, 2023.
Chalar, G., Arocena, R., Pacheco, J. P. and Fabián, D: Trophic assessment of streams in Uruguay: a trophic State Index for Benthic Invertebrates (TSI-BI), Ecol. Indic., 11, 362–369, https://doi.org/10.1016/j.ecolind.2010.06.004, 2011.
Chapelle, G. and Peck, L. S.: Polar gigantism dictated by oxygen availability, Nature, 399, 114–115, https://doi.org/10.1038/20099, 1999.
Chesney, E. J., Baltz, D. M., and Thomas, R. G.: Louisiana estuarine and coastal fisheries and habitats: perspectives from a fish's eye view, Ecol. Appl., 10, 350–366, https://doi.org/10.1890/1051-0761(2000)010[0350:leacfa]2.0.co;2, 2000.
Cheung, W. W., Sarmiento, J. L., Dunne, J., Frölicher, T. L., Lam, V. W., Deng Palomares, M. L., Watson, R., Pauly, D.: Shrinking of fishes exacerbates impacts of global ocean changes on marine ecosystems, Nat. Clim. Change, 3, 254–258, 2013.
Christensen, V. and Walters, C. J.: Ecopath with Ecosim: methods, capabilities and limitations, Ecol. Model., 172, 109–139, https://doi.org/10.1016/j.ecolmodel.2003.09.003, 2004.
Chu, J. W. F. and Gale, K. S. P.: Ecophysiological limits to aerobic metabolism in hypoxia determine epibenthic distributions and energy sequestration in the northeast Pacific ocean, Limnol. Oceanogr., 62, 59–74, https://doi.org/10.1002/lno.10370, 2016.
Chu, J. W. F., Nephin, J., Georgian, S., Knudby, A., Rooper, C., and Gale, K. S. P.: Modeling the environmental niche space and distributions of cold-water corals and sponges in the Canadian northeast Pacific Ocean, Deep-Sea Res. Pt. I, 151, 103063, https://doi.org/10.1016/j.dsr.2019.06.009, 2019.
Chung, M.-T., Trueman, C. N., Godiksen, J. A., Holmstrup, M. E., and Grønkjær, P.: Field metabolic rates of teleost fishes are recorded in otolith carbonate, Commun. Biol., 2, 24–24, https://doi.org/10.1038/s42003-018-0266-5, 2019.
Clarke, T. M., Wabnitz, C. C. C., Striegel, S., Frölicher, T. L., Reygondeau, G., and Cheung, W. W. L.: Aerobic growth index (AGI): An index to understand the impacts of ocean warming and deoxygenation on global marine fisheries resources, Prog. Oceanogr., 195, 102588, https://doi.org/10.1016/j.pocean.2021.102588, 2021.
Cosme, N. and Hauschild, M. Z.: Effect Factors for marine eutrophication in LCIA based on species sensitivity to hypoxia, Ecol. Indic., 69, 453–462, https://doi.org/10.1016/j.ecolind.2016.04.006, 2016.
Cosme, N., Jones, M. C., Cheung, W. W. L., and Larsen, H. F.: Spatial differentiation of marine eutrophication damage indicators based on species density, Ecol. Indic., 73, 676–685, https://doi.org/10.1016/j.ecolind.2016.10.026, 2017.
Costantini, M., Ludsin, S. A., Mason, D. M., Zhang, X., Boicourt, W. C., and Brandt, S. B.: Effect of hypoxia on habitat quality of striped bass (Morone saxatilis) in Chesapeake Bay, Can. J. Fish. Aquat. Sci., 65, 989–1002, https://doi.org/10.1139/f08-021, 2008.
Craig, J.: Aggregation on the edge: effects of hypoxia avoidance on the spatial distribution of brown shrimp and demersal fishes in the Northern Gulf of Mexico, Mar. Ecol. Prog. Ser., 445, 75–95, https://doi.org/10.3354/meps09437, 2012.
Cronin, T. M. and Vann, C. D.: The sedimentary record of climatic and anthropogenic influence on the Patuxent estuary and Chesapeake Bay ecosystems, Estuaries, 26, 196–209, https://doi.org/10.1007/BF02695962, 2003.
Dai, M., Zhao, Y., Chai, F., Chen, M., Chen, N., Chen, Y., Cheng, D., Gan, J., Guan, D., Hong, Y., Huang, J., Lee, Y., Leung, K. M. Y., Lim, P. E., Lin, S., Lin, X., Liu, X., Liu, Z., Luo, Y.-W., Meng, F., Sangmanee, C., Shen, Y., Uthaipan, K., Wan Talaat, W. I. A., Wan, X. S., Wang, C., Wang, D., Wang, G., Wang, S., Wang, Y., Wang, Y., Wang, Z., Wang, Z., Xu, Y., Yang, J.-Y. T., Yang, Y., Yasuhara, M., Yu, D., Yu, J., Yu, L., Zhang, Z., and Zhang, Z.: Persistent eutrophication and hypoxia in the coastal ocean, Cambridge Prisms: Coastal Futures, 1, https://doi.org/10.1017/cft.2023.7, 2023.
Dauvin, J. C.: Twenty years of application of Polychaete/Amphipod ratios to assess diverse human pressures in estuarine and coastal marine environments: A review, Ecol. Indic., 95, 427–435, https://doi.org/10.1016/j.ecolind.2018.07.049, 2018.
Davies, S. M., Sánchez-Velasco, L., Beier, E., Godínez, V. M., Barton, E. D., and Tamayo, A.: Three-dimensional distribution of larval fish habitats in the shallow oxygen minimum zone in the eastern tropical Pacific Ocean off Mexico, Deep-Sea Res. Pt. I, 101, 118–129, https://doi.org/10.1016/j.dsr.2015.04.003, 2015.
Deutsch, C., Ferrel, A., Seibel, B., Pörtner, H.-O., and Huey, R. B.: Climate change tightens a metabolic constraint on marine habitats, Science, 348, 1132–1135, https://doi.org/10.1126/science.aaa1605, 2015.
Deutsch, C., Penn, J. L., and Seibel, B.: Metabolic trait diversity shapes marine biogeography, Nature, 585, 557–562, https://doi.org/10.1038/s41586-020-2721-y, 2020.
Deutsch, C., Penn, J. L., Verberk, W. C. E. P., Inomura, K., Endress, M.-G., and Payne, J. L.: Impact of warming on aquatic body sizes explained by metabolic scaling from microbes to macrofauna, P. Natl. Acad. Sci. USA, 119, e2201345119–e2201345119, https://doi.org/10.1073/pnas.2201345119, 2022.
Deutsch, C., Penn, J. L., and Lucey, N.: Climate, Oxygen, and the Future of Marine Biodiversity, Annu. Rev. Mar. Sci., 16, 217–245, https://doi.org/10.1146/annurev-marine-040323-095231, 2024.
Diaz, R. J. and Breitburg, D. L.: Chapter 1 The Hypoxic Environment, Fish Physiol., 27, 1–23, https://doi.org/10.1016/s1546-5098(08)00001-0, 2009.
Diaz, R. J. and Rosenberg, R.: Marine benthic hypoxia: a review of its ecological effects and the behavioural responses of benthic macrofauna, Oceanography and marine biology, An annual review, 33, 245–303, 1995.
Diaz, R. J. and Rosenberg, R.: Spreading Dead Zones and Consequences for Marine Ecosystems, Science, 321, 926–929, https://doi.org/10.1126/science.1156401, 2008.
Doak, D. F., Waddle, E., Langendorf, R. E., Louthan, A. M., Isabelle Chardon, N., Dibner, R. R., Keinath, D. A., Lombardi, E., Steenbock, C., Shriver, R. K., Linares, C., Begoña Garcia, M., Funk, W. C., Fitzpatrick, S. W., Morris, W. F., and DeMarche, M. L.: A critical comparison of integral projection and matrix projection models for demographic analysis, Ecol. Monogr., 91, e01447, https://doi.org/10.1002/ecm.1447, 2021.
Ducrotoy, J.-P., Michael, E., Cutts, N. D., Franco, A., Little, S., Mazik, K., and Wilkinson, M.: Temperate Estuaries: Their Ecology Under Future Environmental Changes, Coast. Estuar., Elsevier, 577–594, https://doi.org/10.1016/b978-0-12-814003-1.00033-2, 2019.
Dunn, D. C., Van Dover, C. L., Etter, R. J., Smith, C. R., Levin, L. A., Morato, T., Colaço, A., Dale, A. C., Gebruk, A. V., Gjerde, K. M., Halpin, P. N.: A strategy for the conservation of biodiversity on mid-ocean ridges from deep-sea mining, Sci. Adv., 4, eaar4313, https://doi.org/10.1126/sciadv.aar4313, 2018.
DePasquale, E., Baumann, H., and Gobier, C. J.: Vulnerability of early life stage Northwest Atlantic forage fish to ocean acidification and low oxygen., Mar. Ecol. Prog. Ser., 523, 145–156, https://doi.org/10.3354/meps11142, 2015.
Duskey, E.: Metabolic prioritization of fish in hypoxic waters: an integrative modeling approach, Front. Mar. Sci., 10, 1206506, https://doi.org/10.3389/fmars.2023.1206506, 2023.
Duskey, E., Casini, M., Limburg, K., and Gårdmark, A.: Declining food availability and habitat shifts 600 drive community responses to marine hypoxia, bioRxiv (Cold Spring Harbor Laboratory), 04, https://doi.org/10.1101/2023.04.14.536810, 2023.
Eby, L. A. and Crowder, L. B.: Hypoxia-based habitat compression in the Neuse River Estuary: context-dependent shifts in behavioral avoidance thresholds, Can. J. Fish. Aquat. Sci., 59, 952–965, https://doi.org/10.1139/f02-067, 2002.
Eby, L., Crowder, L., McClellan, C., Peterson, C., and Powers, M.: Habitat degradation from intermittent hypoxia: impacts on demersal fishes, Mar. Ecol. Prog. Ser., 291, 249–262, https://doi.org/10.3354/meps291249, 2005.
Ekau, W., Auel, H., Pörtner, H.-O., and Gilbert, D.: Impacts of hypoxia on the structure and processes in pelagic communities (zooplankton, macro-invertebrates and fish), Biogeosciences, 7, 1669–1699, https://doi.org/10.5194/bg-7-1669-2010, 2010.
Enomoto, M., Ito, S., Takahashi, M., Sassa, C., Higuchi, T., and Shirai, K.: Vertical habitat shifts of juvenile jack mackerel estimated using otolith oxygen stable isotope, Prog. Oceanogr., 208, 102897, https://doi.org/10.1016/j.pocean.2022.102897, 2022.
Franco, A. C., Kim, H., Frenzel, H., Deutsch, C., Ianson, D., Sumaila, U. R., and Tortell, P. D.: Impact of warming and deoxygenation on the habitat distribution of Pacific halibut in the Northeast Pacific, Fish. Oceanogr., 31, 601–614, https://doi.org/10.1111/fog.12610, 2022.
Friedland, R., Macias, D., Cossarini, G., Daewel, U., Estournel, C., Garcia-Gorriz, E., Grizzetti, B., Grégoire, M., Gustafson, B., Kalaroni, S., Kerimoglu, O., Lazzari, P., Lenhart, H., Lessin, G., Maljutenko, I., Miladinova, S., Müller-Karulis, B., Neumann, T., Parn, O., Pätsch, J., Piroddi, C., Raudsepp, U., Schrum, C., Stegert, C., Stips, A., Tsiaras, K., Ulses, C., and Vandenbulcke, L.: Effects of Nutrient Management Scenarios on Marine Eutrophication Indicators: A Pan-European, Multi-Model Assessment in Support of the Marine Strategy Framework Directive, Front. Mar. Sci., 8, 596126, https://doi.org/10.3389/fmars.2021.596126, 2021.
Fry, F. E. T. and Hart, J. S.: The relation of temperature and oxygen consumption in goldfish, Biol. Bull., 94, 66–77, 1948.
Gallo, N. D.: Influence of ocean deoxygenation on demersal fish communities: Lessons from upwelling margins and oxygen minimum zones, UC San Diego, 369 pp., https://escholarship.org/uc/item/6bb6v4z8 (last access: 7 November 2024), 2018.
Gallo, N., Beckwith, M., Wei, C., Levin, L., Kuhnz, L., and Barry, J.: Dissolved oxygen and temperature best predict deep-sea fish community structure in the Gulf of California with climate change implications, Mar. Ecol. Prog. Ser., 637, 159–180, https://doi.org/10.3354/meps13240, 2020.
Gallo, N. D. and Levin, L. A.: Fish Ecology and Evolution in the World's Oxygen Minimum Zones and Implications of Ocean Deoxygenation, Adv. Mar. Biol., 117–198, https://doi.org/10.1016/bs.amb.2016.04.001, 2016.
Gallo, N. D., Levin, L. A., Beckwith, M., and Barry, J. P.: Home sweet suboxic home: remarkable hypoxia tolerance in two demersal fish species in the Gulf of California, Ecology, 100, 5, https://doi.org/10.1002/ecy.2539, 2018.
Garçon, V., Karstensen, J., Palacz, A., Telszewski, M., Aparco Lara, T., Breitburg, D., Chavez, F., Coelho, P., Cornejo-D'Ottone, M., Santos, C., Fiedler, B., Gallo, N. D., Grégoire, M., Gutierrez, D., Hernandez-Ayon, M., Isensee, K., Koslow, T., Levin, L., Marsac, F., Maske, H., Mbaye, B. C., Montes, I., Naqvi, W., Pearlman, J., Pinto, E., Pitcher, G., Pizarro, O., Rose, K., Shenoy, D., Van der Plas, A., Vito, M. R., and Weng, K.: Multidisciplinary Observing in the World Ocean's Oxygen Minimum Zone Regions: From Climate to Fish – The VOICE Initiative, Front. Mar. Sci., 6, 2296–7745, https://doi.org/10.3389/fmars.2019.00722, 2019.
Gascuel, D., Bozec, Y.-M., Chassot, E., Colomb, A., and Laurans, M.: The trophic spectrum: theory and application as an ecosystem indicator, ICES J. Mar. Sci., 62, 443–452, https://doi.org/10.1016/j.icesjms.2004.12.013, 2005.
Gillooly, J. F., Brown, J. H., West, G. B., Savage, V. M., and Charnov, E. L.: Effects of Size and Temperature on Metabolic Rate, Science, 293, 2248–2251, https://doi.org/10.1126/science.1061967, 2001.
Glaspie, C. N., Clouse, M. A., Adamack, A. T., Cha, Y., Ludsin, S. A., Mason, D. M., Roman, M. R., Stow, C. A., and Brandt, S. B.: Effect of Hypoxia on Diet of Atlantic Bumpers in the Northern Gulf of Mexico, T. Am. Fish. Soc., 147, 740–748, https://doi.org/10.1002/tafs.10063, 2018.
Glud, R. N., Thamdrup, B., Stahl, H., Wenzhoefer, F., Glud, A., Nomaki, H., Oguri, K., Revsbech, N. P., and Kitazato, H.: Nitrogen cycling in a deep ocean margin sediment (Sagami Bay, Japan), Limnol. Oceanogr., 54, 723–734, https://doi.org/10.4319/lo.2009.54.3.0723, 2009.
Gooday, A. J., Bernhard, J. M., Levin, L. A., and Suhr, S. B.: Foraminifera in the Arabian Sea oxygen minimum zone and other oxygen-deficient settings: taxonomic composition, diversity, and relation to metazoan faunas, Deep-Sea Res. Pt. II, 47, 25–54, https://doi.org/10.1016/s0967-0645(99)00099-5, 2000.
Gooday, A. J., Levin, L. A., Aranda da Silva, A., Bett, B. J., Cowie, G. L., Dissard, D., Gage, J. D., Hughes, D. J., Jeffreys, R., Lamont, P. A., Larkin, K. E., Murty, S. J., Schumacher, S., Whitcraft, C., and Woulds, C.: Faunal responses to oxygen gradients on the Pakistan margin: A comparison of foraminiferans, macrofauna and megafauna, Deep-Sea Res. Pt. II, 56, 488–502, https://doi.org/10.1016/j.dsr2.2008.10.003, 2009a.
Gooday, A. J., Bett, B. J., Escobar, E., Ingole, B., Levin, L. A., Neira, C., Raman, A. V., and Sellanes, J.: Habitat heterogeneity and its influence on benthic biodiversity in oxygen minimum zones, Mar. Ecol., 31, 125–147, https://doi.org/10.1111/j.1439-0485.2009.00348.x, 2010.
Grégoire, M., Garçon, V., Garcia, H., Breitburg, D., Isensee, K., Oschlies, A., Telszewski, M., Barth, A., Bittig, H. C., Carstensen, J., Carval, T., Chai, F., Chavez, F., Conley, D., Coppola, L., Crowe, S., Currie, K., Dai, M., Deflandre, B., Dewitte, B., Diaz, R., Garcia-Robledo, E., Gilbert, D., Giorgetti, A., Glud, R., Gutierrez, D., Hosoda, S., Ishii, M., Jacinto, G., Langdon, C., Lauvset, S. K., Levin, L. A., Limburg, K. E., Mehrtens, H., Montes, I., Naqvi, W., Paulmier, A., Pfeil, B., Pitcher, G., Pouliquen, S., Rabalais, N., Rabouille, C., Recape, V., Roman, M., Rose, K., Rudnick, D., Rummer, J., Schmechtig, C., Schmidtko, S., Seibel, B., Slomp, C., Sumalia, U. R., Tanhua, T., Thierry, V., Uchida, H., Wanninkhof, R., and Yasuhara, M.: A Global Ocean Oxygen Database and Atlas for Assessing and Predicting Deoxygenation and Ocean Health in the Open and Coastal Ocean, Front. Mar. Sci., 8, 724913, https://doi.org/10.3389/fmars.2021.724913, 2021.
Gregory, T. R. and Wood, C. M.: The effects of chronic plasma cortisol elevation on the feeding behaviour, growth, competitive ability, and swimming performance of juvenile rainbow trout, Phys. Biochem. Zool., 72, 286–295, 1999.
Guo, Y., Wu, C., and Sun, J.: Pathogenic bacteria significantly increased under oxygen depletion in coastal waters: A continuous observation in the central Bohai Sea, Front. Microbiol., 13, 1035904–1035904, https://doi.org/10.3389/fmicb.2022.1035904, 2022.
Gutiérrez, D., Enríquez, E., Purca, S., Quipúzcoa, L., Marquina, R., Flores, G., and Graco, M.: Oxygenation episodes on the continental shelf of central Peru: Remote forcing and benthic ecosystem response, Prog. Oceanogr., 79, 177–189, https://doi.org/10.1016/j.pocean.2008.10.025, 2008.
Hale, S. S., Cicchetti, G., and Deacutis, C. F.: Eutrophication and Hypoxia Diminish Ecosystem Functions of Benthic Communities in a New England Estuary, Front. Mar. Sci., 3, 249, https://doi.org/10.3389/fmars.2016.00249, 2016.
Herbinger, C. M. and Friars, G. W.: Correlation between condition factor and total lipid content in Atlantic salmon, Salmo salar L., parr, Aquac. Res., 22, 527–529, https://doi.org/10.1111/j.1365-2109.1991.tb00766.x, 1991.
Hofmann, A. F., Peltzer, E. T., Walz, P. M., and Brewer, P. G.: Hypoxia by degrees: Establishing definitions for a changing ocean, Deep-Sea Res. Pt. I, 58, 1212–1226, https://doi.org/10.1016/j.dsr.2011.09.004, 2011.
Hou, Z.-S., Wen, H.-S., Li, J.-F., He, F., Li, Y., and Qi, X.: Environmental hypoxia causes growth retardation, osteoclast differentiation and calcium dyshomeostasis in juvenile rainbow trout (Oncorhynchus mykiss), Sci. Total Environ., 705, 135272, https://doi.org/10.1016/j.scitotenv.2019.135272, 2020.
Houde, E. D.: Patterns and consequences of selective processes in teleost early life histories, Early Life History and Recruitment in Fish Populations, Springer, Dordrecht, 173–196, https://doi.org/10.1007/978-94-009-1439-1_6, 1997.
Howard, E. M., Penn, J. L., Frenzel, H., Seibel, B. A., Bianchi, D., Renault, L., Kessouri, F., Sutula, M. A., McWilliams, J. C., and Deutsch, C.: Climate-driven aerobic habitat loss in the California Current System, Sci. Adv., 15, eaay3188, https://doi.org/10.1126/sciadv.aay3188, 2020.
Hughes, A. C. and Grumbine, R. E.: The Kunming-Montreal Global Biodiversity Framework: what it does and does not do, and how to improve it, Front. Environ. Sci., 11, 281536, https://doi.org/10.3389/fenvs.2023.1281536, 2023.
Hunter, W. R., Levin, L. A., Kitazato, H., and Witte, U.: Macrobenthic assemblage structure and organismal stoichiometry control faunal processing of particulate organic carbon and nitrogen in oxygen minimum zone sediments, Biogeosciences, 9, 993–1006, https://doi.org/10.5194/bg-9-993-2012, 2012.
Ingham, M. C., Cook, S. K., and Hausknecht, K. A.: Oxycline characteristics and skipjack tuna distribution in the southeastern tropical Atlantic, Fish. Bull., 75, 857–865, 1977.
Itakura, H., O'Brien, M. H. P., and Secor, D.: Tracking oxy-thermal habitat compression encountered by Chesapeake Bay striped bass through acoustic telemetry, ICES J. Mar. Sci., 78, 1049–1062, https://doi.org/10.1093/icesjms/fsab009, 2021.
Jackson, L. E., Kurtz, J. C., and Fisher, W. S.: Evaluation Guidelines for Ecol. Indic., United States, U.S. Environmental Protection Agency, Office of Research and Development, EPA/620/R-99/005, 2000.
Jacobsen, A., de Miranda Azevedo, R., Juty, N., Batista, D., Coles, S., Cornet, R., Courtot, M., Crosas, M., Dumontier, M., Evelo, C. T., Goble, C., Guizzardi, G., Hansen, K. K., Hasnain, A., Hettne, K., Heringa, J., Hooft, R. W. W., Imming, M., Jeffery, K. G., Kaliyaperumal, R., Kersloot, M. G., Kirkpatrick, C. R., Kuhn, T., Labastida, I., Magagna, B., McQuilton, P., Meyers, N., Montesanti, A., van Reisen, M., Rocca-Serra, P., Pergl, R., Sansone, S.-A., da Silva Santos, L. O. B., Schneider, J., Strawn, G., Thompson, M., Waagmeester, A., Weigel, T., Wilkinson, M. D., Willighagen, E. L., Wittenburg, P., Roos, M., Mons, B., and Schultes, E.: FAIR Principles: Interpretations and Implementation Considerations, Data Intelligence, 2, 10–29, https://doi.org/10.1162/dint_r_00024, 2020.
Jeffreys, R., Levin, L., Lamont, P., Woulds, C., Whitcraft, C., Mendoza, G., Wolff, G., and Cowie, G.: Living on the edge: single-species dominance at the Pakistan oxygen minimum zone boundary, Mar. Ecol. Prog. Ser., 470, 79–99, https://doi.org/10.3354/meps10019, 2012.
Jones, J., Hunter, E., Hambach, B., Wilding, M., and Trueman, C. N.: Individual variation in field metabolic rates of wild living fish have phenotypic and ontogenetic underpinnings: insights from stable isotope compositions of otoliths, Frontiers in Ecology and Evolution, 11, 1161105, https://doi.org/10.3389/fevo.2023.1161105, 2023.
Jung, S. and Houde, E.: Production of bay anchovy Anchoa mitchilli in Chesapeake Bay: application of size-based theory, Mar. Ecol. Prog. Ser., 281, 217–232, https://doi.org/10.3354/meps281217, 2004.
Kallio, P. J., Okamoto, K., O'Brien, S., Carrero, P., Makino, Y., Tanaka, H., and Poellinger, L.: Signal transduction in hypoxic cells: inducible nuclear translocation and recruitment of the CBP/p300 coactivator by the hypoxia-inducible factor-1alpha, EMBO J., 17, 6573–6586, https://doi.org/10.1093/emboj/17.22.6573, 1998.
Karlsen, A. W., Cronin, T. M., Ishman, S. E., Willard, D. A., Kerhin, R., Holmes, C. W., and Marot, M.: Historical Trends in Chesapeake Bay Dissolved Oxygen Based on Benthic Foraminifera from Sediment Cores, Estuaries, 23, 488–508, https://doi.org/10.2307/1353141, 2000.
Keister, J., Houde, E., and Breitburg, D.: Effects of bottom-layer hypoxia on abundances and depth distributions of organisms in Patuxent River, Chesapeake Bay, Mar. Ecol. Prog. Ser., 205, 43–59, https://doi.org/10.3354/meps205043, 2000.
Keister, J. E. and Tuttle, L. B.: Effects of bottom-layer hypoxia on spatial distributions and community structure of mesozooplankton in a sub-estuary of Puget Sound, Washington, USA, Limnol. Oceanogr., 58, 667–680, https://doi.org/10.4319/lo.2013.58.2.0667, 2013.
Keller, A. A., Ciannelli, L., Wakefield, W. W., Simon, V., Barth, J. A., and Pierce, S. D.: Occurrence of demersal fishes in relation to near-bottom oxygen levels within the California Current large marine ecosystem, Fish. Oceanogr., 24, 162–176, https://doi.org/10.1111/fog.12100, 2015.
Kim, H., Franco, A. C., and Sumaila, U. R.: A Selected Review of Impacts of Ocean Deoxygenation on Fish and Fisheries, Fishes, 8, 316, https://doi.org/10.3390/fishes8060316, 2023.
Kimmel, D. G., Boynton, W. R., and Roman, M. R.: Long-term decline in the calanoid copepod Acartia tonsa in central Chesapeake Bay, USA: An indirect effect of eutrophication?, Estuar. Coast. Shelf Sci., 101, 76–85, https://doi.org/10.1016/j.ecss.2012.02.019, 2012.
Kraus, R. T., Secor, D. H., and Wingate, R. L.: Testing the thermal-niche oxygen-squeeze hypothesis for estuarine striped bass, Environ. Biol. Fish., 98, 2083–2092, https://doi.org/10.1007/s10641-015-0431-3, 2015.
Laffoley, D. and Baxter, J, M.: Ocean deoxygenation: Everyone's problem-causes, impacts, consequences and solutions, Gland, Switzerland, IUCN, 2019.
Lai, K. P., Wang, S. Y., Li, J. W., Tong, Y., Chan, T. F., Jin, N., Tse, A., Zhang, J. W., Wan, M. T., Tam, N., Au, D. W. T., Lee, B.-Y., Lee, J.-S., Wong, A. S. T., Kong, R. Y. C., and Wu, R. S. S.: Hypoxia Causes Transgenerational Impairment of Ovarian Development and Hatching Success in Fish, Environ. Sci. Technol., 53, 3917–3928, https://doi.org/10.1021/acs.est.8b07250, 2019.
Léger, J. A., Athanasio, C. G., Zhera, A., Chauhan, M. F., and Simmons, D. B.: Hypoxic responses in Oncorhynchus mykiss involve angiogenesis, lipid, and lactate metabolism, which may be triggered by the cortisol stress response and epigenetic methylation, Comparative Biochemistry and Physiology Part D: Genomics and Proteomics, 39, 100860, 2021.
Leung, J. Y. S. and McAfee, D.: Stress across life stages: Impacts, responses and consequences for marine organisms, Sci. Total Environ., 700, 134491, https://doi.org/10.1016/j.scitotenv.2019.134491, 2020.
Levin, L. A.: Oxygen minimum zone benthos: adaptations and community responses to hypoxia. Oceanography and Marine Biology: An Annual Review, 41, 1–45, 2003.
Levin, L., Gutiérrez, D., Rathburn, A., Neira, C., Sellanes, J., Muñoz, P., Gallardo, V., and Salamanca, M.: Benthic processes on the Peru margin: a transect across the oxygen minimum zone during the 1997–98 El Niño, Prog. Oceanogr., 53, 1–27, https://doi.org/10.1016/s0079-6611(02)00022-8, 2002.
Levin, L. A. and Gage, J. D.: Relationships between oxygen, organic matter and the diversity of bathyal macrofauna, Deep-Sea Res. Pt. II, 45, 129–163, https://doi.org/10.1016/s0967-0645(97)00085-4, 1998.
Levin, L. A., Huggett, C. L., and Wishner, K. F.: Control of deep-sea benthic community structure by oxygen and organic-matter gradients in the eastern Pacific Ocean, J. Mar. Res., 49, 763–800, https://doi.org/10.1357/002224091784995756, 1991.
Levin, L. A., Rathburn, A. E., Gutiérrez, D., Muñoz, P., and Shankle, A.: Bioturbation by symbiont-bearing annelids in near-anoxic sediments: Implications for biofacies models and paleo-oxygen assessments, Palaeogeogr. Palaeocl., 199, 129–140, https://doi.org/10.1016/s0031-0182(03)00500-5, 2003.
Levin, L. A., Ekau, W., Gooday, A. J., Jorissen, F., Middelburg, J. J., Naqvi, S. W. A., Neira, C., Rabalais, N. N., and Zhang, J.: Effects of natural and human-induced hypoxia on coastal benthos, Biogeosciences, 6, 2063–2098, https://doi.org/10.5194/bg-6-2063-2009, 2009a.
Levin, L. A., Whitcraft, C. R., Mendoza, G. F., Gonzalez, J. P., and Cowie, G.: Oxygen and organic matter thresholds for benthic faunal activity on the Pakistan margin oxygen minimum zone (700–1100m), Deep-Sea Res. Pt. II, 56, 449–471, https://doi.org/10.1016/j.dsr2.2008.05.032, 2009b.
Levin, L. A., McGregor, A. L., Mendoza, G. F., Woulds, C., Cross, P., Witte, U., Gooday, A. J., Cowie, G., and Kitazato, H.: Macrofaunal colonization across the Indian margin oxygen minimum zone, Biogeosciences, 10, 7161–7177, https://doi.org/10.5194/bg-10-7161-2013, 2013.
Levin, L. A., Alfaro-Lucas, J. M., Colaço, A., Cordes, E. E., Craik, N., Danovaro, R., Hoving, H.-J., Ingels, J., Mestre, N. C., Seabrook, S., Thurber, A. R., Vivian, C., and Yasuhara, M.: Deep-sea impacts of climate interventions, Science, 379, 978–981, https://doi.org/10.1126/science.ade7521, 2023.
Lim, H.-S., Diaz, R. J., Hong, J.-S., and Schaffner, L. C.: Hypoxia and benthic community recovery in Korean coastal waters, Mar. Pollut. Bull., 52, 1517–1526, https://doi.org/10.1016/j.marpolbul.2006.05.013, 2006.
Limburg, K. E. and Casini, M.: Effect of Marine Hypoxia on Baltic Sea Cod Gadus morhua: Evidence From Otolith Chemical Proxies, Front. Mar. Sci., 5, 482, https://doi.org/10.3389/fmars.2018.00482, 2018.
Limburg, K. E. and Casini, M.: Otolith chemistry indicates recent worsened Baltic cod condition is linked to hypoxia exposure, Biol. Lett., 15, 20190352–20190352, https://doi.org/10.1098/rsbl.2019.0352, 2019.
Limburg, K. E., Walther, B. D., Lu, Z., Jackman, G., Mohan, J., Walther, Y., Nissling, A., Weber, P. K., and Schmitt, A. K.: In search of the dead zone: Use of otoliths for tracking fish exposure to hypoxia, J. Mar. Syst., 141, 167–178, https://doi.org/10.1016/j.jmarsys.2014.02.014, 2015.
Limburg, K. E., Wuenschel, M. J., Hüssy, K., Heimbrand, Y., and Samson, M.: Making the otolith magnesium chemical calendar-clock tick: plausible mechanism and empirical evidence, Rev. Fish. Sci. Aquac., 26, 479–493, https://doi.org/10.1080/23308249.2018.1458817, 2018.
Lindstrom, E., Gunn, J., Fischer, A., McCurdy, A., Glover, L. K., and Members, T. T.: A Framework for Ocean Observing, European Space Agency, https://doi.org/10.5270/oceanobs09-foo, 2012.
Long, W. C., Seitz, R. D., Brylawski, B. J., and Lipcius, R. N.: Individual, population, and ecosystem effects of hypoxia on a dominant benthic bivalve in Chesapeake Bay, Ecol. Monogr., 84, 303–327, https://doi.org/10.1890/13-0440.1, 2014.
Maximov, A. A. and Berezina, N. A.: Benthic opportunistic polychaete/amphipod ratio: an indicator of pollution or modification of the environment by macroinvertebrates?, J. Mar. Sci. Eng., 12, 190, https://doi.org/10.3390/jmse11010190, 2023.
May, E. B.: Extensive oxygen depletion in Mobile Bay, Alabama, Limnol. Oceanogr., 18, 353–366, https://doi.org/10.4319/lo.1973.18.3.0353, 1973.
McCormick, L. R. and Levin, L. A.: Physiological and ecological implications of ocean deoxygenation for vision in marine organisms, Philos. Trans. A Math. Phys. Eng. Sci., 375, 20160322, https://doi.org/10.1098/rsta.2016.0322, 2017.
McCormick, L. R., Levin, L. A., and Oesch, N. W.: Vision is highly sensitive to oxygen availability in marine invertebrate larvae, J. Exp. Biol., 15, 222, https://doi.org/10.1242/jeb.200899, 2019.
McCormick, L. R., Gangrade, S., Garwood, J. C., Oesch, N. W., and Levin, L. A.: Oxygen and irradiance constraints on visual habitat in a changing ocean: The luminoxyscape, Limnol. Oceanogr. Letters, 8, 220–228, https://doi.org/10.1002/lol2.10296, 2022.
Middelburg, J. J. and Levin, L. A.: Coastal hypoxia and sediment biogeochemistry, Biogeosciences, 6, 1273–1293, https://doi.org/10.5194/bg-6-1273-2009, 2009.
Miller, M.-E. C. and Graham, W. M.: Environmental evidence that seasonal hypoxia enhances survival and success of jellyfish polyps in the northern Gulf of Mexico, J. Exp. Mar. Biol. Ecol., 432–433, 113–120, https://doi.org/10.1016/j.jembe.2012.07.015, 2012.
Miloslavich, P., Bax, N. J., Simmons, S. E., Klein, E., Appeltans, W., Aburto-Oropeza, O., Andersen Garcia, M., Batten, S. D., Benedetti-Cecchi, L., Checkley, D. M., Chiba, S., Duffy, J. E., Dunn, D. C., Fischer, A., Gunn, J., Kudela, R., Marsac, F., Muller-Karger, F. E., Obura, D., and Shin, Y.: Essential ocean variables for global sustained observations of biodiversity and ecosystem changes, Glob. Change Biol., 24, 2416–2433, https://doi.org/10.1111/gcb.14108, 2018.
Moffitt, S. E., Hill, T. M., Ohkushi, K., Kennett, J. P., and Behl, R. J.: Vertical oxygen minimum zone oscillations since 20 ka in Santa Barbara Basin: A benthic foraminiferal community perspective, Paleoceanography, 29, 44–57, https://doi.org/10.1002/2013pa002483, 2014.
Moffitt, S. E., Moffitt, R. A., Sauthoff, W., Davis, C. V., Hewett, K., and Hill, T. M.: Paleoceanographic insights on recent oxygen minimum zone expansion: lessons for modern oceanography, PLoS One, 10, e0115246–e0115246, https://doi.org/10.1371/journal.pone.0115246, 2015.
Murphy, C. A., Rose, K. A., Rahman, M. S., and Thomas, P.: Testing and applying a fish vitellogenesis model to evaluate laboratory and field biomarkers of endocrine disruption in Atlantic croaker (Micropogonias undulatus) exposed to hypoxia, Environ. Toxicol. Chem., 28, 1288–1303, https://doi.org/10.1897/08-304.1, 2009.
Muto, D., Ishimura, T., Takahashi, M., and Nishida, K.: Extracting daily isotopic records on fish otolith (Trachurus japonicus) by combining micro-milling and micro-scale isotopic analysis (MICAL-CF-IRMS), Rapid Commun. Mass Spectrom., 36, e9366, https://doi.org/10.1002/rcm.9366, 2022.
de Mutsert, K., Steenbeek, J., Cowan, J. H., and Christensen, V.: Using Ecosystem Modeling to Determine Hypoxia Effects on Fish and Fisheries, Modeling Coastal Hypoxia, Springer, 377–400, https://doi.org/10.1007/978-3-319-54571-4_14, 2017.
Navarro, M. O., Kwan, G. T., Batalov, O., Choi, C. Y., Pierce, N. T., and Levin, L. A.: Development of Embryonic Market Squid, Doryteuthis opalescens, under Chronic Exposure to Low Environmental pH and [O2], PLoS One, 11, e0167461–e0167461, https://doi.org/10.1371/journal.pone.0167461, 2016.
Neira, C., Sellanes, J., Levin, L. A., and Arntz, W. E.: Meiofaunal distributions on the Peru margin, Deep-Sea Res. Pt. I, 48, 2453–2472, https://doi.org/10.1016/s0967-0637(01)00018-8, 2001.
Ng, C. A. and Micheli, F.: Short-term effects of hypoxia are more important than effects of ocean acidification on grazing interactions with juvenile giant kelp (Macrocystis pyrifera), Sci. Rep.-UK, 10, 5403–5403, https://doi.org/10.1038/s41598-020-62294-3, 2020.
Nixon, S. W. and Buckley, B. A.: “A strikingly rich zone”—Nutrient enrichment and secondary production in coastal marine ecosystems, Estuaries, 25, 782–796, https://doi.org/10.1007/bf02804905, 2002.
Norris, R. D., Turner, S. K., Hull, P. M., and Ridgwell, A.: Marine Ecosystem Responses to Cenozoic Global Change, Science, 341, 492–498, https://doi.org/10.1126/science.1240543, 2013.
Ogino, T. and Toyohara, H.: Identification of possible hypoxia sensor for behavioral responses in a marine annelid, Capitella teleta, Biol. Open, 8, bio037630, https://doi.org/10.1242/bio.037630, 2019.
Osterman, L. E. Benthic foraminifers from the continental shelf and slope of the Gulf of Mexico: an indicator of shelf hypoxia, Estuar. Coast. Shelf Sci., 58, 17–35, https://doi.org/10.1016/S0272-7714(02)00352-9, 2003.
Overstreet R. M.: Parasitic diseases of fishes and their relationship with toxicants and other environmental factors, in: Pathobiology of marine and estuarine organisms, 111–156, CRC Press, https://doi.org/10.1201/9781003069058-5, 2021.
Parouffe, A., Garçon, V., Dewitte, B., Paulmier, A., Montes, I., Parada, C., Mecho, A., and Veliz, D.: Evaluating future climate change exposure of marine habitat in the South East Pacific based on metabolic constraints, Front. Mar. Sci., 9, 1055875, https://doi.org/10.3389/fmars.2022.1055875, 2023.
Pearson, T. A.: Macrobenthic succession in relation to organic enrichment and pollution of the marine environment, Oceanography and marine biology: an annual review, 16, 229–311, 1987.
Penn, J. L., Deutsch, C., Payne, J. L., and Sperling, E. A.: Temperature-dependent hypoxia explains biogeography and severity of end-Permian marine mass extinction, Science, 162, eaat1327, https://doi.org/10.1126/science.aat1327, 2018.
Pierson, J. J., Roman, M. R., Kimmel, D. G., Boicourt, W. C., and Zhang, X.: Quantifying changes in the vertical distribution of mesozooplankton in response to hypoxic bottom waters, J. Exp. Mar. Biol. Ecol., 381, S74–S79, https://doi.org/10.1016/j.jembe.2009.07.013, 2009.
Pierson, J. J., Testa, J. M., and Roman, M. R.: Copepod habitat suitability estimates vary among oxygen metrics in Chesapeake Bay, ICES J. Mar. Sci., 79, 855–867, https://doi.org/10.1093/icesjms/fsac019, 2022.
Piló, D., Pereira, F., Carriço, A., Cúrdia, J., Pereira, P., Gaspar, M. B., and Carvalho, S.: Temporal variability of biodiversity patterns and trophic structure of estuarine macrobenthic assemblages along a gradient of metal contamination, Estuar. Coast. Shelf Sci., 167, 286–299, https://doi.org/10.1016/j.ecss.2015.06.018, 2015.
Piña-Ochoa, E., Koho, K., Geslin, E., and Risgaard-Petersen, N.: Survival and life strategy of the foraminiferan Globobulimina turgida through nitrate storage and denitrification, Mar. Ecol. Prog. Ser., 417, 39–49, https://doi.org/10.3354/meps08805, 2010.
Pineda, J., Cho, W., Starczak, V., Govindarajan, A. F., Guzman, H. M., Girdhar, Y., Holleman, R. C., Churchill, J., Singh, H., and Ralston, D. K.: A crab swarm at an ecological hotspot: patchiness and population density from AUV observations at a coastal, tropical seamount, PeerJ, 4, e1770–e1770, https://doi.org/10.7717/peerj.1770, 2016.
Pörtner, H. O. and Knust, R.: Climate Change Affects Marine Fishes Through the Oxygen Limitation of Thermal Tolerance, Science, 315, 95–97, https://doi.org/10.1126/science.1135471, 2007.
Pothoven, S. A., Vanderploeg, H. A., Höök, T. O., and Ludsin, S. A.: Hypoxia modifies planktivore–zooplankton interactions in Lake Erie, Can. J. Fish. Aquat. Sci., 69, 2018–2028, https://doi.org/10.1139/cjfas-2012-0144, 2012.
Purcell, J. E.: Jellyfish and Ctenophore Blooms Coincide with Human Proliferations and Environmental Perturbations, Annu. Rev. Mar. Sci., 4, 209–235, https://doi.org/10.1146/annurev-marine-120709-142751, 2012.
Rabalais, N. N. and Baustian, M. M.: Historical Shifts in Benthic Infaunal Diversity in the Northern Gulf of Mexico since the Appearance of Seasonally Severe Hypoxia, Diversity, 12, 49, https://doi.org/10.3390/d12020049, 2020.
Rahman, M. S. and Thomas, P.: Molecular and biochemical responses of hypoxia exposure in Atlantic croaker collected from hypoxic regions in the northern Gulf of Mexico, PLoS One, 12, e0184341–e0184341, https://doi.org/10.1371/journal.pone.0184341, 2017.
Reddin, C. J., Nätscher, P. S., Kocsis, Á. T., Pörtner, H. O., and Kiessling, W.: Marine clade sensitivities to climate change conform across timescales, Nat. Clim. Change, 10, 249–253, https://doi.org/10.1038/s41558-020-0690-7, 2020.
Reynolds, J. H., Knutson, M. G., Newman, K. B., Silverman, E. D., and Thompson, W. L.: A road map for designing and implementing a biological monitoring program, Environ. Monit. Assess., 188, 1–25, https://doi.org/10.1007/s10661-016-5397-x , 2016.
Rice, A. M.: Extension Programming In Support of Public Policy For The Management of Aquaculture In Common Water Bodies, Aquacultura Indonesiana, 15, 26–31, https://doi.org/10.21534/ai.v15i1.22, 2015.
Richmond, C., Marcus, N. H., Sedlacek, C., Miller, G. A., and Oppert, C.: Hypoxia and seasonal temperature: Short-term effects and long-term implications for Acartia tonsa dana, J. Exp. Mar. Biol. Ecol., 328, 177–196, https://doi.org/10.1016/j.jembe.2005.07.004, 2006.
Risgaard-Petersen, N., Langezaal, A. M., Ingvardsen, S., Schmid, M. C., Jetten, M. S., Op den Camp, H. J., and van der Zwaan, G. J.: Evidence for complete denitrification in a benthic foraminifer, Nature, 443, 93–96, https://doi.org/10.1038/nature05070, 2006.
Roman, M. R., Gauzens, A. L., Rhinehart, W. K., and White, J. R.: Effects of low oxygen waters on Chesapeake Bay zooplankton, Limnol. Oceanogr., 38, 1603–1614, https://doi.org/10.4319/lo.1993.38.8.1603, 1993.
Roman, M. R., Pierson, J. J., Kimmel, D. G., Boicourt, W. C., and Zhang, X.: Impacts of Hypoxia on Zooplankton Spatial Distributions in the Northern Gulf of Mexico, Estuar. Coasts, 35, 1261–1269, https://doi.org/10.1007/s12237-012-9531-x, 2012.
Roman, M. R., Brandt, S. B., Houde, E. D., and Pierson, J. J.: Interactive Effects of Hypoxia and Temperature on Coastal Pelagic Zooplankton and Fish, Front. Mar. Sci., 6, 139, https://doi.org/10.3389/fmars.2019.00139, 2019.
Rose, K. A., Cowan, J. H., Winemiller, K. O., Myers, R. A., and Hilborn, R.: Compensatory density dependence in fish populations: importance, controversy, understanding and prognosis, Fish Fish., 2, 293–327, https://doi.org/10.1046/j.1467-2960.2001.00056.x, 2001.
Rose, K. A., Creekmore, S., Thomas, P., Craig, J. K., Rahman, M. S., and Neilan, R. M.: Modeling the Population Effects of Hypoxia on Atlantic Croaker (Micropogonias undulatus) in the Northwestern Gulf of Mexico: Part 1–Model Description and Idealized Hypoxia, Estuar. Coasts, 41, 233–254, https://doi.org/10.1007/s12237-017-0266-6, 2017a.
Rose, K. A., Creekmore, S., Justić, D., Thomas, P., Craig, J. K., Neilan, R. M., Wang, L., Rahman, M. S., and Kidwell, D.: Modeling the Population Effects of Hypoxia on Atlantic Croaker (Micropogonias undulatus) in the Northwestern Gulf of Mexico: Part 2–Realistic Hypoxia and Eutrophication, Estuar. Coasts, 41, 255–279, https://doi.org/10.1007/s12237-017-0267-5, 2017b.
Rose, K. A., Gutiérrez, D., Breitburg, D., Conley, D., Craig, K. J., Froehlich, H. E., Jeyabaskaran, R., Kripa, V., Mbaye, B. C., Mohamed, K. S., and Padua, S.: Impacts of ocean deoxygenation on fisheries.. In: Ocean deoxygenation: Everyone’s problem Causes, impacts, consequences and solutions, International Union for Conservation of Nature and Natural Resources, Gland, Switzerland, 519–544, ISBN 978-2-8317-2013-5, 2019.
Rosenberg, R.: Benthic Faunal Recovery in a Swedish Fjord Following the Closure of a Sulphite Pulp Mill, Oikos, 23, 92–108, https://doi.org/10.2307/3543930, 1972.
Rosenberg, R., Arntz, W. E., de Flores, E. C., Flores, L. A., Carbajal, G., Finger, I., and Tarazona, J.: Benthos biomass and oxygen deficiency in the upwelling system off Peru, J. Mar. Res., 41, 263–279, https://doi.org/10.1357/002224083788520153, 1983.
Rosenzweig, M. L. and Abramsky, Z.: How are diversity and productivity related?, in: Species diversity in ecological communities, 52–65, University of Chicago Press, 1993.
Rubalcaba, J. G., Verberk, W. C. E. P., Hendriks, A. J., Saris, B., and Woods, H. A.: Oxygen limitation may affect the temperature and size dependence of metabolism in aquatic ectotherms, P. Natl. Acad. Sci. USA, 117, 31963–31968, https://doi.org/10.1073/pnas.2003292117, 2020.
Rytkönen, K. T., Williams, T. A., Renshaw, G. M., Primmer, C. R., and Nikinmaa, M.: Molecular Evolution of the Metazoan PHD–HIF Oxygen-Sensing System, Mol. Biol. Evol., 28, 1913–1926, https://doi.org/10.1093/molbev/msr012, 2011.
Sadoul, B. and Geffroy, B.: Measuring cortisol, the major stress hormone in fishes, J. Fish Biol., 94, 540–555, https://doi.org/10.1111/jfb.13904, 2019.
Sakamoto, T., Takahashi, M., Chung, M., Rykaczewski, R. R., Komatsu, K., Shirai, K., Ishimura, T., and Higuchi, T.:Contrasting life-history responses to climate variability in eastern and western North Pacific sardine populations, Nat. Commun., 13, 5298, https://doi.org/10.1038/s41467-022-33019-z, 2022.
Sampaio, E., Santos, C., Rosa, I. C., Ferreira, V., Pörtner, H.-O., Duarte, C. M., Levin, L. A., and Rosa, R.: Impacts of hypoxic events surpass those of future ocean warming and acidification, Nat. Ecol. Evol., 5, 311–321, https://doi.org/10.1038/s41559-020-01370-3, 2021.
Sánchez-García, M. A., Zottoli, S. J., and Roberson, L. M.: Hypoxia Has a Lasting Effect on Fast-Startle Behavior of the Tropical Fish, Haemulon plumieri, Biol. Bull., 237, 48–62, https://doi.org/10.1086/704337, 2019.
Scavia, D. and Donnelly, K. A.: Reassessing Hypoxia Forecasts for the Gulf of Mexico, Environ. Sci. Technol., 41, 8111–8117, https://doi.org/10.1021/es0714235, 2007.
Schwacke, L. H., Gulland, F. M., and White, S.: Sentinel Species in Oceans and Human Health, Environ. Toxicol., Springer, New York, NY, 503–528, https://doi.org/10.1007/978-1-4614-5764-0_18, 2012.
Seitz, R. D., Dauer, D. M., Llansó, R. J., and Long, W. C.: Broad-scale effects of hypoxia on benthic community structure in Chesapeake Bay, USA, J. Exp. Mar. Biol. Ecol., 381, S4–S12, https://doi.org/10.1016/j.jembe.2009.07.004, 2009.
Sen Gupta, B. K. and Machain-Castillo, M. L.: Benthic foraminifera in oxygen-poor habitats, Mar. Micropaleontol., 20, 183–201, https://doi.org/10.1016/0377-8398(93)90032-s, 1993.
Shelton, A. O., Gold, Z. J., Jensen, A. J., D'Agnese, E., Andruszkiewicz Allan, E., Van Cise, A., Gallego, R., Ramón-Laca, A., Garber-Yonts, M., Parsons, K., and Kelly, R. P.: Toward quantitative metabarcoding, Ecology, 104, e3906, https://doi.org/10.1002/ecy.3906, 2023.
Shin, P. K. S., Leung, J. Y. S., Qiu, J. W., Ang, P. O., Chiu, J. M. Y., Thiyagarajan, V., and Cheung, S. G.: Hypoxia induces abnormal larval development and affects biofilm–larval interaction in the serpulid polychaete Hydroides elegans, Mar. Pollut. Bull., 76, 291–297, https://doi.org/10.1016/j.marpolbul.2013.08.022, 2013.
Shin, P. K. S., Leung, J. Y. S., Qiu, J. W., Ang, P. O., Chiu, J. M. Y., Thiyagarajan, V., and Cheung, S. G.: Acute hypoxic exposure affects gamete quality and subsequent fertilization success and embryonic development in a serpulid polychaete, Mar. Pollut. Bull., 85, 439–445, https://doi.org/10.1016/j.marpolbul.2014.03.009, 2014.
Singh, A. D., Rai, A. K., Verma, K., Das, S., and Bharti, S. K.: Benthic foraminiferal diversity response to the climate induced changes in the eastern Arabian Sea oxygen minimum zone during the last 30kaBP, Quatern. Int., 374, 118–125, https://doi.org/10.1016/j.quaint.2014.11.052, 2015.
Smith, C. R., Levin, L. A., Hoover, D. J., McMurtry, G., and Gage, J. D.: Variations in bioturbation across the oxygen minimum zone in the northwest Arabian Sea, Deep-Sea Res. Pt. II, 47, 227–257, https://doi.org/10.1016/S0967-0645(99)00108-3, 2000.
Smith, M. D. and Crowder, L. B.: Valuing Ecosystem Services with Fishery Rents: A Lumped-Parameter Approach to Hypoxia in the Neuse River Estuary, Sustainability, 3, 2229–2267, https://doi.org/10.3390/su3112229, 2011.
Sperling, E. A., Frieder, C. A., Raman, A. V., Girguis, P. R., Levin, L. A., and Knoll, A. H.: Oxygen, ecology, and the Cambrian radiation of animals, P. Natl. Acad. Sci. USA, 110, 13446–13451, https://doi.org/10.1073/pnas.1312778110, 2013.
Sperling, E. A., Frieder, C. A., and Levin, L. A.: Biodiversity response to natural gradients of multiple stressors on continental margins, Proc. Biol. Sci., 283, 20160637, https://doi.org/10.1098/rspb.2016.0637, 2016.
Stalder, L. C. and Marcus, N. H.: Zooplankton responses to hypoxia: behavioral patterns and survival of three species of calanoid copepods, Mar. Biol., 127, 599–607, https://doi.org/10.1007/s002270050050, 1997.
Steckbauer, A., Duarte, C. M., Carstensen, J., Vaquer-Sunyer, R., and Conley, D. J.: Ecosystem impacts of hypoxia: thresholds of hypoxia and pathways to recovery, Environ. Res. Lett., 6, 025003, https://doi.org/10.1088/1748-9326/6/2/025003, 2011.
Stierhoff, K. L., Targett, T. E., and Power, J. H.: Hypoxia-induced growth limitation of juvenile fishes in an estuarine nursery: assessment of small-scale temporal dynamics using RNA:DNA, Can. J. Fish. Aquat. Sci., 66, 1033–1047, https://doi.org/10.1139/f09-066, 2009.
Stoicescu, S.-T., Lips, U., and Liblik, T.: Assessment of Eutrophication Status Based on Sub-Surface Oxygen Conditions in the Gulf of Finland (Baltic Sea), Front. Mar. Sci., 6, 54, https://doi.org/10.3389/fmars.2019.00054, 2019.
Stramma, L., Johnson, G. C., Sprintall, J., and Mohrholz, V.: Expanding Oxygen-Minimum Zones in the Tropical Oceans, Science, 320, 655–658, https://doi.org/10.1126/science.1153847, 2008.
Stramma, L., Schmidtko, S., Levin, L. A., and Johnson, G. C.: Ocean oxygen minima expansions and their biological impacts, Deep-Sea Res. Pt. I, 57, 587–595, https://doi.org/10.1016/j.dsr.2010.01.005, 2010.
Stramma, L., Prince, E. D., Schmidtko, S., Luo, J., Hoolihan, J. P., Visbeck, M., Wallace, D. W. R., Brandt, P., and Körtzinger, A.: Expansion of oxygen minimum zones may reduce available habitat for tropical pelagic fishes, Nat. Clim. Change, 2, 33–37, https://doi.org/10.1038/nclimate1304, 2011.
Suzue, T., Wu, G. B., and Furukawa, T.: High susceptibility to hypoxia of afferent synaptic transmission in the goldfish sacculus, J. Neurophysiol., 58, 1066–1079, https://doi.org/10.1152/jn.1987.58.5.1066, 1987.
Thomas, P. and Rahman, Md. S.: Biomarkers of hypoxia exposure and reproductive function in Atlantic croaker: A review with some preliminary findings from the northern Gulf of Mexico hypoxic zone, J. Exp. Mar. Biol. Ecol., 381, S38–S50, https://doi.org/10.1016/j.jembe.2009.07.008, 2009.
Thomas, P., Rahman, Md. S., Picha, M. E., and Tan, W.: Impaired gamete production and viability in Atlantic croaker collected throughout the 20,000 km2 hypoxic region in the northern Gulf of Mexico, Mar. Pollut. Bull., 101, 182–192, https://doi.org/10.1016/j.marpolbul.2015.11.001, 2015.
Thronson, A. and Quigg, A.: Fifty-Five Years of Fish Kills in Coastal Texas, Estuar. Coasts, 31, 802–813, https://doi.org/10.1007/s12237-008-9056-5, 2008.
Tigert, L. R. and Porteus, C. S.: Invited review - the effects of anthropogenic abiotic stressors on the sensory systems of fishes, Comp. Biochem. Phys. A, 277, 111366, https://doi.org/10.1016/j.cbpa.2022.111366, 2023.
Tremblay, N., Hünerlage, K., and Werner, T.: Hypoxia Tolerance of 10 Euphausiid Species in Relation to Vertical Temperature and Oxygen Gradients, Front. Physiol., 11, 248–248, https://doi.org/10.3389/fphys.2020.00248, 2020.
Trouwborst, R. E., Clement, B. G., Tebo, B. M., Glazer, B. T., and Luther, G. W.: Soluble Mn(III) in Suboxic Zones, Science, 313, 1955–1957, https://doi.org/10.1126/science.1132876, 2006.
Tsujimoto, A., Nomura, R., Yasuhara, M., Yamazaki, H., and Yoshikawa, S.: Impact of eutrophication on shallow marine benthic foraminifers over the last 150 years in Osaka Bay, Japan, Mar. Micropaleontol., 60, 258–268, https://doi.org/10.1016/j.marmicro.2006.06.001, 2006.
Tsujimoto, A., Yasuhara, M., Nomura, R., Yamazaki, H., Sampei, Y., Hirose, K., and Yoshikawa, S.: Development of modern benthic ecosystems in eutrophic coastal oceans: the foraminiferal record over the last 200 years, Osaka Bay, Japan, Mar. Micropaleontol., 69, 225–239, https://doi.org/10.1016/j.marmicro.2008.08.001, 2008.
Turner, R. E.: Some effects of eutrophication on pelagic and demersal marine food webs, Coast. Estuar. Stud., 58, 371–398, https://doi.org/10.1029/ce058p0371, 2001.
Uye, S.: Replacement of large copepods by small ones with eutrophication of embayments: cause and consequence, Ecology and Morphology of Copepods, 513–519, https://doi.org/10.1007/978-94-017-1347-4_64, 1994.
Valenza, A. N., Altenritter, M. E., and Walther, B. D.: Reconstructing consequences of lifetime hypoxia exposure on metabolism of demersal fish in the northern Gulf of Mexico using otolith chemistry, Environ. Biol. Fish., 106, 2045–2057, https://doi.org/10.1007/s10641-023-01483-1, 2023.
Vaquer-Sunyer, R. and Duarte, C. M.: Thresholds of hypoxia for marine biodiversity, P. Natl. Acad. Sci. USA, 105, 15452–15457, https://doi.org/10.1073/pnas.0803833105, 2008.
Vedor, M., Queiroz, N., Mucientes, G., Couto, A., Costa, I. da, Santos, A. D., Vandeperre, F., Fontes, J., Afonso, P., Rosa, R., Humphries, N. E., and Sims, D. W.: Climate-driven deoxygenation elevates fishing vulnerability for the ocean's widest ranging shark, Elife, 10, e62508, https://doi.org/10.7554/eLife.62508, 2021.
Verberk, W. C. E. P., Atkinson, D., Hoefnagel, K. N., Hirst, A. G., Horne, C. R., and Siepel, H.: Shrinking body sizes in response to warming: explanations for the temperature-size rule with special emphasis on the role of oxygen, Biol. Rev. Camb. Philos., 96, 247–268, https://doi.org/10.1111/brv.12653, 2021.
Wang, S. Y., Lau, K., Lai, K.-P., Zhang, J.-W., Tse, A. C.-K., Li, J.-W., Tong, Y., Chan, T.-F., Wong, C. K.-C., Chiu, J. M.-Y., Au, D. W.-T., Wong, A. S.-T., Kong, R. Y.-C., and Wu, R. S.-S.: Hypoxia causes transgenerational impairments in reproduction of fish, Nat. Commun., 7, 12114–12114, https://doi.org/10.1038/ncomms12114, 2016.
Warwick, R. M.: The nematode/copepod ratio and its use in pollution ecology, Marine Pollution Bulletin, 12, 329–333, https://doi.org/10.1016/0025-326X(81)90105-3, 1981
von Weissenberg, E., Jansson, A., Vuori, K. A., and Engström-Öst, J.: Copepod reproductive effort and oxidative status as responses to warming in the marine environment, Ecol. Evol., 12, e8594–e8594, https://doi.org/10.1002/ece3.8594, 2022.
Wishner, K. F., Ashjian, C. J., Gelfman, C., Gowing, M. M., Kann, L., Levin, L. A., Mullineaux, L. S., and Saltzman, J.: Pelagic and benthic ecology of the lower interface of the Eastern Tropical Pacific oxygen minimum zone, Deep-Sea Res. Pt. I, 42, 93–115, https://doi.org/10.1016/0967-0637(94)00021-j, 1995.
Wishner, K. F., Outram, D. M., Seibel, B. A., Daly, K. L., and Williams, R. L.: Zooplankton in the eastern tropical north Pacific: Boundary effects of oxygen minimum zone expansion, Deep-Sea Res. Pt. I, 79, 122–140, https://doi.org/10.1016/j.dsr.2013.05.012, 2013.
Wong, M. K., Nobata, S., Ito, S., and Hyodo, S.: Development of species-specific multiplex real time PCR assays for tracing the small pelagic fishes of North Pacific with environmental DNA, Environmental DNA, 4, 510-522, https://doi.org/10.1002/edn3.275, 2022.
Woods, H. A., Moran, A. L., Atkinson, D., Audzijonyte, A., Berenbrink, M., Borges, F. O., Burnett, K. G., Burnett, L. E., Coates, C. J., Collin, R., Costa-Paiva, E. M., Duncan, M. I., Ern, R., Laetz, E. M. J., Levin, L. A., Lindmark, M., Lucey, N. M., McCormick, L. R., Pierson, J. J., Rosa, R., Roman, M. R., Sampaio, E., Schulte, P. M., Sperling, E. A., Walczyńska, A., and Verberk, W. C. E. P.: Integrative Approaches to Understanding Organismal Responses to Aquatic Deoxygenation, Biol. Bull., 243, 85–103, https://doi.org/10.1086/722899, 2022.
Word, J. Q.: Classification of benthic invertebrates into infaunal trophic index feeding groups, Coastal Water Research Project Biennial Report, 103–121, 1978.
Woulds, C., Cowie, G. L., Levin, L. A., Andersson, J. H., Middelburg, J. J., Vandewiele, S., Lamont, P. A., Larkin, K. E., Gooday, A. J., Schumacher, S., Whitcraft, C., Jeffreys, R. M., and Schwartz, M.: Oxygen as a control on sea floor biological communities and their roles in sedimentary carbon cycling, Limnol. Oceanogr., 52, 1698–1709, https://doi.org/10.4319/lo.2007.52.4.1698, 2007.
Wu, R. S. S.: Hypoxia: from molecular responses to ecosystem responses, Mar. Pollut. Bull., 45, 35–45, https://doi.org/10.1016/s0025-326x(02)00061-9, 2002.
Xing, Q., Yu, H., Ito, S., and Chai, F.: Mesoscale eddies modulate the dynamics of human fishing activities in the global midlatitude ocean, Fish Fish., 24, 527–543, https://doi.org/10.1111/faf.12742, 2023.
Yasuhara, M., Yamazaki, H., Tsujimoto, A., and Hirose, K.: The effect of long-term spatiotemporal variations in urbanization-induced eutrophication on a benthic ecosystem, Osaka Bay, Japan, Limnol. Oceanogr., 52, 1633–1644, https://doi.org/10.4319/lo.2007.52.4.1633, 2007.
Yasuhara, M., Hunt, G., Breitburg, D., Tsujimoto, A., and Katsuki, K.: Human-induced marine ecological degradation: micropaleontological perspectives, Ecol. Evol., 2, 3242–3268, https://doi.org/10.1002/ece3.425, 2012.
Yoccoz, N. G., Nichols, J. D., and Boulinier, T.: Monitoring of biological diversity in space and time, Trends Ecol. Evol., 16, 446–453, https://doi.org/10.1016/S0169-5347(01)02205-4, 2002.
Zamorano, P., Hendrickx, M. E., and Toledano-Granados, A.: Distribution and ecology of deep-water mollusks from the continental slope, southeastern Gulf of California, Mexico, Mar. Biol., 150, 883–892, https://doi.org/10.1007/s00227-006-0390-5, 2006.
Zettler, M., Bochert, R., and Pollehne, F.: Macrozoobenthic biodiversity patterns in the northern province of the Benguela upwelling system, Afr. J. Mar. Sci., 35, 283–290, https://doi.org/10.2989/1814232x.2013.798592, 2013.
Zettler, M. L., Bochert, R., and Pollehne, F.: Macrozoobenthos diversity in an oxygen minimum zone off northern Namibia, Mar. Biol., 156, 1949–1961, https://doi.org/10.1007/s00227-009-1227-9, 2009.
Zhan, Y., Ning, B., Sun, J., and Chang, Y.: Living in a hypoxic world: A review of the impacts of hypoxia on aquaculture, Mar. Pollut. Bull., 194, 115207, https://doi.org/10.1016/j.marpolbul.2023.115207, 2023.
Zhang, J., Gilbert, D., Gooday, A. J., Levin, L., Naqvi, S. W. A., Middelburg, J. J., Scranton, M., Ekau, W., Peña, A., Dewitte, B., Oguz, T., Monteiro, P. M. S., Urban, E., Rabalais, N. N., Ittekkot, V., Kemp, W. M., Ulloa, O., Elmgren, R., Escobar-Briones, E., and Van der Plas, A. K.: Natural and human-induced hypoxia and consequences for coastal areas: synthesis and future development, Biogeosciences, 7, 1443–1467, https://doi.org/10.5194/bg-7-1443-2010, 2010.
Zhang, J., Zhu, Z., Mo, W. Y., Liu, S. M., Wang, D. R., and Zhang, G. S.: Hypoxia and nutrient dynamics affected by marine aquaculture in a monsoon-regulated tropical coastal lagoon, Environ. Monit. Assess., 190, 656, https://doi.org/10.1007/s10661-018-7001-z, 2018a.
Zhang, Q., Tango, P. J., Murphy, R. R., Forsyth, M. K., Tian, R., Keisman, J., and Trentacoste, E. M.: Chesapeake Bay Dissolved Oxygen Criterion Attainment Deficit: Three Decades of Temporal and Spatial Patterns, Front. Mar. Sci., 5, 422, https://doi.org/10.3389/fmars.2018.00422, 2018b.