the Creative Commons Attribution 4.0 License.
the Creative Commons Attribution 4.0 License.
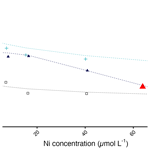
Phytoplankton response to increased nickel in the context of ocean alkalinity enhancement
Giulia Faucher
Ulf Riebesell
Ocean alkalinity enhancement (OAE) is considered one of the most promising approaches to actively remove carbon dioxide (CO2) from the atmosphere by accelerating the natural process of rock weathering. This approach involves introducing alkaline substances sourced from natural mineral deposits, such as olivine, basalt, and carbonates or obtained from industrial waste products such as steel slag, into seawater and dispersing them over coastal areas. Some of these natural and industrial substances contain trace metals, which would be released into the oceans along with the alkalinity enhancement. The trace metals could serve as micronutrients for marine organisms at low concentrations but could potentially become toxic at high concentrations, adversely affecting marine biota. To comprehensively assess the feasibility of OAE, it is crucial to understand how the phytoplankton, which forms the base of marine food webs, responds to ocean alkalinization and associated trace metal perturbations. As one of the most abundant metals in OAE source materials, understanding the impacts of nickel (Ni) on the phytoplankton is critical for OAE assessment. In this study, we investigated the influence of nickel (Ni) on three representative phytoplankton species over a gradient of nine Ni concentrations (from 0 to 100 µmol L−1 with 12 µmol L−1 synthetic organic ligand). The impacts of elevated Ni varied among the tested phytoplankton species. The coccolithophore Emiliania huxleyi and the dinoflagellate Amphidinium carterae exhibited a growth rate inhibition of about 30 % and 20 %, respectively, at the highest Ni concentrations. The half maximal inhibitory concentration (IC50, at which the growth rate is inhibited by 50 %) of both species exceeded the tested range of Ni. This suggests that both species were only mildly affected by the elevated Ni concentrations. In contrast, the diatom Thalassiosira weissflogii displayed a considerably higher sensitivity to Ni, with a 60 % growth rate inhibition at the highest Ni concentration and an IC50 value of 63.9 µmol L−1. In conclusion, the variability in phytoplankton sensitivity to Ni exposure suggests that for OAE applications with Ni-rich materials caution is required and critical toxic thresholds for Ni must be avoided.
- Article
(912 KB) - Full-text XML
-
Supplement
(364 KB) - BibTeX
- EndNote
The progressive release of anthropogenic carbon dioxide (CO2) into the atmosphere since the industrial revolution has resulted in a multitude of environmental challenges, including global warming, ocean acidification, ecosystem alteration, increasing frequency of extreme climatic events, and food insecurity (Pörtner et al., 2022). To keep the effects of climate change within acceptable limits, about 1 to 15 GtCO2 yr−1 must be captured by 2100 (Rogelj et al., 2018). Carbon capture activities, known as negative emission technologies (NETs), have become the focus of discussion as they will need to be implemented over the next 2 decades to meet the climate targets and limit global warming to <2 ∘C (Shepherd, 2009; Allan et al., 2021). One of the promising NETs is to accelerate natural rock weathering by introducing finely ground alkaline products on land (enhanced weathering, EW) or into the surface ocean (ocean alkalinity enhancement, OAE) to remove CO2 from the atmosphere (Minx et al., 2018; Bach et al., 2019). OAE, in addition to enhancing the buffering capacity of seawater, has the co-benefit of mitigating ocean acidification at the deployment sites (Köhler et al., 2010). Natural alkaline minerals, as well as by-products from industrial activity, are potential candidates for EW and OAE (Taylor et al., 2016; Renforth, 2019). Among the most recognized alkaline minerals, olivine rocks have gained considerable attention due to their relatively fast weathering rate, wide availability, and low cost (Schuiling and Krijgsman, 2006; Hartmann et al., 2013). These rocks contain high amounts of trace metals, e.g., nickel (Ni) and chromium (Cr) (Montserrat et al., 2017; Amann et al., 2020) that through OAE could affect coastal and offshore systems, possibly influencing marine communities (Gaillardet et al., 2003).
In seawater, Ni is present at low concentrations (Donat et al., 1994; Mackey et al., 2002; Saito et al., 2004) and acts as a micronutrient when urea serves as the nitrogen source (Muyssen et al., 2004; Egleston and Morel, 2008). However, this metal at elevated concentrations may emerge as a concern to marine ecosystems, due to its toxicity, bioaccumulation, and biogeochemical cycling (Sclater et al., 1976; Hall and Anderson, 1995; Horvatić and Peršić, 2007; Debelius et al., 2011; DeForest and Schlekat, 2013; Martínez-Ruiz and Martínez-Jerónimo, 2015; Karthikeyan et al., 2018). In olivine-based OAE scenarios, Ni concentrations could rapidly rise above critical levels that become harmful to marine organisms (Montserrat et al., 2017; Hartmann et al., 2023). To date, studies on high Ni concentrations are scarce. In this study, we examined the impacts of Ni on three representative marine phytoplankton species: the diatom Thalassiosira weissflogii, the dinoflagellate Amphidinium carterae, and the coccolithophore Emiliania huxleyi. These species were selected as they represent three dominant functional groups of phytoplankton. This research aimed to (1) investigate how different phytoplankton species respond to a gradient of Ni concentrations and (2) compare the inter-species differences in Ni sensitivity.
2.1 Strain and culture conditions
Experiments were conducted with cultures of the marine coccolithophore Emiliania huxleyi B92/11 (Plymouth Marine Laboratory), the dinoflagellate Amphidinium carterae CCAP1102 (University of Oldenburg), and the diatom Thalassiosira weissflogii CCMP1336 (Bigelow Laboratory for Ocean Sciences). Algae were cultivated in sterile-filtered (0.2 µm) media prepared with artificial seawater (Guillard and Ryther, 1962; Kester et al., 1967). Media were enriched with essential trace metals buffered by ethylenediaminetetraacetic acid (EDTA, 12 µmol L−1). Cells grew at 18 ∘C with a 12 : 12 h light and dark cycle under 200 µmol photons m−2 s−1 of photosynthetically active radiation (PAR). Media were acclimated to the incubation temperature prior to inoculation from the pre-cultures to avoid a potential thermal shock.
2.2 Experimental setup
To determine the toxicity of nickel, a stock solution (as NiCl2⋅6H2O) was prepared, with a nominal value of 50 mmol L−1. All bottles were soaked with 10 % HCl (Fisher) for 24 h and rinsed with Milli-Q water before the experiment. Stock solutions of Ni were added to the algal media for different Ni concentration treatments (0.01, 0.1, 1, 5, 10, 20, 50, and 100 µmol L−1) and the control with exclusive medium. Experiments were performed in triplicate independent 75 mL falcon flasks for each concentration. All cultures were gently turned by hand twice a day to avoid cells from settling. Samples were always collected at the same time of day between 09:00 and 10:00 to avoid an effect of the photocycle. Samples (1 mL) of each flask were collected into sterile 2 mL microtubes. The cell density was determined daily with a flow cytometer (BD Accuri™ C6). To minimize the impact of changes in the carbonate chemistry of the medium induced by cellular metabolism, the phytoplankton biomass at the harvest time should consume less than 5 % of the total dissolved inorganic carbon (Zondervan et al., 2002). The maximum density was determined based on the cell carbon quota of the tested species. Accordingly, the maximum cell densities of A. carterae, T. weissflogii, and E. huxleyi never exceeded 12 000, 15 000, and 130 000 cells mL−1, respectively (Zondervan et al., 2002; Olenina et al., 2006).
2.3 Growth rate and IC50 value determination
Growth rates (μ; d−1) were calculated from cell density as follows:
where c0 and cf are the cell densities at the beginning and end of the experiment, respectively; d is the duration of the experiment.
The toxic response was expressed as
where I is the growth inhibition and μ is the growth rate.
Dose–response curves were constructed for growth rate following Stephenson et al. (2000). Nonlinear regression models were determined by the least square method. Model equations were chosen based on scatterplots of the growth rates of the different species. The sigmoidal model was applied as
where Y is the growth rate and C is the Ni concentration. The parameter t is the control response, and u and B define the location and shape of the equation, respectively. The half maximal inhibitory concentration (IC50), at which the growth rate is inhibited by 50 %, was determined from the dose–response curve.
2.4 Nickel measurement
At the end of the growth experiment, 30 mL media of each sample were filtered with 0.2 µm sterile disk filters to remove the algae. The filtered media were collected for Ni measurements. The total nickel concentrations were measured with ThermoFisher Scientific ElementXR to ensure that the target concentrations were reached. The measured Ni concentrations were used as input data for the program Visual Minteq 3.1 (Gustafsson, 2013). The software utilizes a chemical equilibrium model to calculate metal speciation and obtain the concentrations of free Ni2+.
2.5 Statistical analysis
Data represent means ± standard deviations (N=3). ANOVA was performed on the cell density and growth rates to assess the effect of Ni concentrations. Differences among treatments were tested with Tukey's HSD ANOVA test. Significant differences were reported at the 95 % confidence level. All statistics were conducted in the RStudio environment (R packages “tidyverse” and “ggplot2”; Posit team, 2023; R Core Team, 2023).
3.1 Ni concentrations
Table 1Target, measured, and free concentrations of Ni for the different phytoplankton cultures at the end of the experiment. SD is standard deviation.
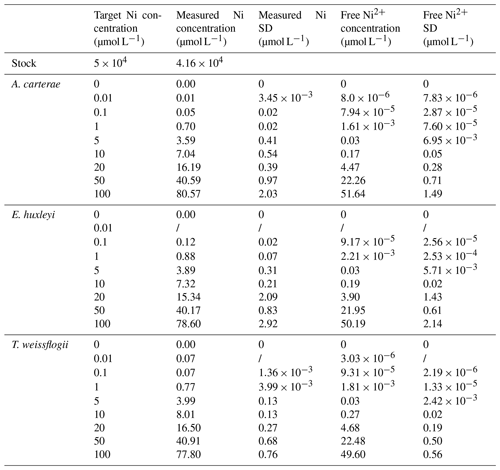
The stock solution was not acidified to avoid pH changes in the culture media. For this reason, nickel carbonate precipitation occurred in the stock solution, and approximately 80 % of the target Ni concentrations were achieved (Table 1, Fig. S1; Gad, 2023). Free Ni2+ was chelated by a ligand at low concentrations, but concentrations of free Ni2+ increased with elevated total Ni (Table 1, Fig. 1). The ligand chelated more Ni2+ with elevated total Ni concentration, while the binding ability decreased. More than 60 % free Ni2+ was beyond ligand binding capacity at the highest Ni concentration.
3.2 Growth response and cell density accumulation
All three species survived the highest tested concentrations. With increasing Ni, the cell densities and growth rates of the three species decreased, albeit differently.
3.2.1 Amphidinium carterae
The cell densities of A. carterae decreased significantly from 7.1 µmol L−1 and the growth rates from 16.2 µmol L−1 Ni concentrations (p<0.05; Fig. 2a, b). Growth was not inhibited until day 4 after the exposure to Ni (Fig. 2c). From 16.2 to 80.6 µmol L−1 Ni concentrations, we observed the maximum decrease in cell density of about 59 %–66 %, with the growth rate decreasing up to 30 % at the highest Ni concentration compared to the control (Fig. 2a, b, Table S1).
3.2.2 Emiliania huxleyi
The cell densities and growth rates of E. huxleyi increased with the addition of Ni up to 3.89 µmol L−1. At 0.9 µmol L−1 Ni, the maximum cell density with a 63 % increase was observed (p<0.01). The growth rate increased by about 11 %, but this value is not statistically significant (p=0.07).
From 15.3 to 78.6 µmol L−1, the cell densities decreased significantly between 57 %–72 % compared to the control (p<0.05; Fig. 3a, Table S1). The decrease in growth rate reached up to 24 % at the highest Ni concentration compared to the control (Fig. 3b, Table S1). The growth variance of E. huxleyi started on day 3 after being exposed to Ni (Fig. 3c).
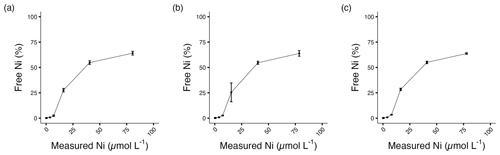
Figure 1Percentage of free Ni2+ in (a) A. carterae, (b) E. huxleyi, and (c) T. weissflogii cultures at the end of the experiment. Error bars denote standard deviations (N=3).
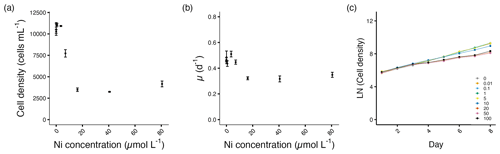
Figure 2Growth performance of A. carterae. (a) Cell densities (cells mL−1) and (b) growth rates (d−1) plotted against measured Ni concentrations on the final experimental day. (c) Log-transformed cell densities plotted against time (day) to indicate the time of response; target Ni concentrations (µmol L−1) used for clarity. Error bars denote standard deviations (N=3). If not visible, error bars are smaller than the symbols.
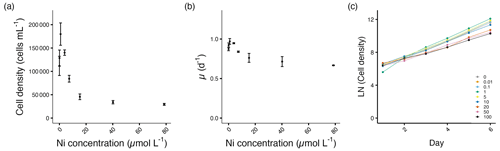
Figure 3Growth performance of E. huxleyi. (a) Cell densities (cells mL−1) and (b) growth rates (d−1) plotted against measured Ni concentrations on the final experimental day. (c) Log-transformed cell densities plotted against time (day) to indicate the time of response; target Ni concentrations (µmol L−1) were used for clarity. Error bars denote standard deviations (N=3). Note that the data point at 0.01 µmol L−1 was excluded in (a) and (b) due to a technical measurement error. If not visible, error bars are smaller than the symbols.
3.2.3 Thalassiosira weissflogii
The cell densities of T. weissflogii remained relatively stable until 8.0 µmol L−1 Ni, above which the densities started to decrease significantly (p<0.05; Fig. 4a, Table S1). Similarly, the growth rates of T. weissflogii remained relatively stable until 40.9 µmol L−1 Ni, above which the growth rates started to decrease significantly (p<0.05; Fig. 4b, Table S1). After being exposed to Ni, T. weissflogii reacted immediately from day 2 onwards (Fig. 4c).
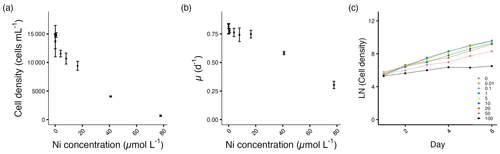
Figure 4Growth performance of T. weissflogii. (a) Cell densities (cells mL−1) and (b) growth rates (d−1) plotted against measured Ni concentrations on the final experimental day. (c) Log-transformed cell densities plotted against time (day) to indicate the time of response; target Ni concentrations (µmol L−1) used for clarity. Error bars denote standard deviations (N=3). If not visible, error bars are smaller than the symbols.
3.3 Determination of IC50
The diatom T. weissflogii has the lowest IC50 value with a concentration of 63.9 µmol L−1, while the IC50 values for A. carterae and E. huxleyi exceed the highest tested Ni concentration (Fig. 5).
4.1 Effects of Ni on marine plankton
Trace metals are required by phytoplankton for numerous physiological processes and biochemical reactions; however, it is difficult to disentangle the distinct role of each element. Ni, for example, is widely recognized to be “bio-required” in several species when urea is utilized as a nitrogen source (Bartha and Ordal, 1965; Pederson et al., 1986; Price and Morel, 1991). However, to our knowledge, no studies have reported that phytoplankton could benefit from supplemented Ni when cultivated in nitrate-enriched media, while only a few studies have documented the tolerances of different taxa to progressively increased (possibly toxic) Ni concentrations (Horvatić and Peršić, 2007; DeForest and Schlekat, 2013; Martínez-Ruiz and Martínez-Jerónimo, 2015; Panneerselvam et al., 2018).
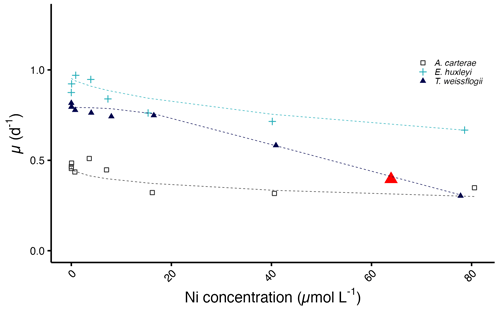
Figure 5Predicted growth curves plotted against measured Ni concentrations. The red triangle denotes the IC50 value of T. weissflogii. Note that the predicted IC50 values of A. carterae and E. huxleyi are not shown as they exceed the highest tested Ni concentration.
Nickel in natural seawater has a concentration lower than 10 nmol L−1 (Gerringa et al., 2021; John et al., 2022) and exists mainly in the form of free Ni2+ (Donat et al., 1994; Achterberg and Van Den Berg, 1997; Saito et al., 2004). Basic and ultrabasic rocks, which are widely recognized source minerals for OAE, would introduce high amounts of Ni into seawater during mineral dissolution (Renforth, 2019). A wide range of Ni content in olivine (0 wt %–0.44 wt %) suggests that the Ni release is source-dependent (Simkin and Smith, 1970). In a previous batch reaction experiment using forsterite olivine sand with 0.26 wt % Ni, an increase of 100 µmol L−1 alkalinity was associated with a parallel increase of approximately 3 µmol L−1 dissolved Ni during the non-stoichiometric dissolution process (Montserrat et al., 2017). According to these results, the concentration of released Ni could potentially reach the highest concentration tested in this study with a doubling of the current ocean alkalinity level, e.g., at the point source of alkalinity release. In real-world applications, the release of alkaline solutions at discrete locations could potentially lead to “hotspots” of alkalinity and associated increases in Ni in seawater (Bach et al., 2019; Caserini et al., 2021). Alkalinity enhancement modeling studies suggest that the phytoplankton may be impacted by the cumulative effects of alkalinity and released trace metals from recurring local addition (Ilyina et al., 2013; Feng et al., 2017).
In this study, we focused on the impacts of a gradient of Ni concentrations on three key species that belong to different phytoplankton groups. The results showed that while the three tested species were able to survive in all treatments, they displayed adverse responses to high Ni concentrations. The diatom species T. weissflogii was the most sensitive species, with an instant reaction to the exposure of Ni and a decrease in cell density when Ni increased to 4.0 µmol L−1. The growth rates of T. weissflogii were unperturbed up to about 40 µmol L−1 Ni and dropped rapidly beyond this threshold. At the highest Ni concentration, its growth rate was reduced by 60 %. The dinoflagellate A. carterae and coccolithophore E. huxleyi are more tolerant to Ni enrichment, with less inhibition in growth rate. The growth rates of A. carterae and E. huxleyi remained relatively constant beyond a certain threshold, and the IC50 values of these two species exceeded the highest tested Ni concentration. Considering the high tolerance of E. huxleyi to several other trace metals such as copper and cadmium (Brand et al., 1986), it is not surprising that this species was found to be mostly unaffected by Ni in our study. For example, to counteract high Cu concentrations, E. huxleyi, regardless of the needs of the cells, can continuously produce an organic Cu ligand (Echeveste et al., 2018). Another study postulates that E. huxleyi survives the Cu stress through an efficient efflux system by exporting intracellular metals (Walsh and Ahner, 2014). We speculate that E. huxleyi may apply analogous strategies to grow at high nickel concentrations. Furthermore, Ni was shown to interact with Ca2+ and Mg2+ transport systems; the uptake of Ca2+ and Mg2+ may compete with Ni for the transport pathways and reduce the uptake of Ni in E. huxleyi (Deleebeeck et al., 2009). Similar strategies to counteract metal stress were observed for the other species. The dinoflagellate A. carterae produces strong ligands to reduce free metal levels (Croot et al., 2000). The production of metal chelators was reported in diatoms and green algae under metal stress (Gerringa et al., 1995; Gonzalez-Davila et al., 1995). The release of a phytochelatin–metal complex might work as a detoxification mechanism of the diatom T. weissflogii (Lee et al., 1996). Sequestering metals into a vacuole or storage complexes or binding metals with small chaperones are also adaptive strategies to buffer the uptake of metals (Blaby-Haas and Merchant, 2012).
Interestingly, we observed an enhancement in the cell densities of E. huxleyi at low Ni concentrations. One possible explanation might be that the introduction of low-dose toxins prompted an increased rate of cell division, a phenomenon known as hormesis. This interpretation differs from the notion of metal limitation. Studies on various phytoplankton groups revealed a similar dose–response pattern, where low doses exhibited beneficial effects and high doses led to toxicity. In these investigations, hormesis was attributed to low increased levels of Cd (Brand et al., 1986) and Cu (Brand et al., 1986; Pérez et al., 2006; Yang et al., 2019). Considering Ni, a slight increase in concentrations positively impacted multiple chlorophyll fluorescence parameters associated with photosynthesis in terrestrial plants, which was explained as a hormetic response (Moustakas et al., 2022). Another potential explanation is that Ni may, to some extent, contribute to the functionality of superoxide dismutase enzymes which are vital components in an organism's defense against oxidative stress (Sunda, 2012). Either way, this growth alteration should not be dismissed, as it could indirectly impact the competitive dynamics within ecosystems containing multiple phytoplankton species.
Due to the increasing interest in olivine-based alkalization applications, recent studies investigated the effects of Ni on marine phytoplankton in the context of OAE. In the study by Guo et al. (2022), most of the tested phytoplankton species did not exhibit growth inhibition in response to the tested Ni concentrations, ranging between 0 and 50 µmol L−1. The inconsistency between our results and those of Guo et al. (2022) could be attributed to the different amounts of bio-available Ni. Guo et al. (2022) utilized a chelator at a high concentration (100 µmol L−1 EDTA), while in our experiment 12 µmol L−1 EDTA was added. EDTA can chelate free trace metal ions, forming metal-EDTA complexes. The ligand serves as a buffer by increasing trace metal availability when trace metal concentrations are low and decreasing trace metal reactivity at excess levels (Van den Berg and Nimmo, 1987). Several studies documented that phytoplankton is sensitive to free Ni2+ rather than total dissolved Ni (Canterford and Canterford, 1980; Morel et al., 1991; Dupont et al., 2010). Indeed, the lower amount of EDTA employed in our study led to 5 orders of magnitude higher concentration of free Ni2+ at the target concentration of 50 µmol L−1, compared to that of Guo et al. (2022). We presume that the variance in the growth inhibition between this study and that of Guo et al. (2022) arises from the discrepancy of free Ni2+ determined by the different amounts of EDTA used in the two studies. Contrarily, Hutchins et al. (2023) showed that most phytoplankton taxa were irresponsive to Ni independent of the concentrations of Ni species and EDTA. However, the study was conducted in a coastal enhanced weathering scenario where the Ni-release process would be gradual (i.e., years) and the olivine utilized for the experiment contained a low amount of Ni, measuring 0.13 µmol L−1 at the highest concentration. Thus, the synthetic olivine dissolution yielded lower Ni concentrations, possibly without reaching threshold values of toxicity compared to those tested in our experiment.
Several studies investigated the impact of Ni at high concentrations on various marine organisms, albeit outside the specific context of OAE. These studies showed a range of sensitivities to Ni among different plankton. For example, certain diatom species with low IC50 and copepod species with LC50 (concentration expected to be lethal to 50 % of the tested organisms) could potentially be vulnerable to the nickel released in the context of OAE. By contrast, the lethal concentration of Ni for the dinoflagellate Prorocentrum donghaiense and the diatom Skeletonema costatum was found to be 1.7 mmol L−1 (Huang et al., 2016), which is unlikely to be encountered during the process of OAE.
DeForest and Schlekat (2013) suggested a threshold of 20.9 µg L−1 Ni (0.35 µmol L−1) as the predicted no effect concentration (PNEC) for chronic Ni toxicity in marine organisms. In a coastal OAE scenario, with a short water residence time, the low Ni released from alkaline particles is unlikely to impact the ecosystem due to the slow dissolution rate (Hutchins et al., 2023; Table 2). In the open ocean, olivine must be ground to a very small size (less than 1 µm) before sinking out of the surface mixed layer (Köhler et al., 2013; Meysman and Montserrat, 2017). Thus, olivine has the potential to release a high quantity of Ni above the IC50 and LC50 values reported in Table 2 for most species. The perturbation could be minimal if the mixing with surrounding waters could rapidly dilute the alkaline solution before impacts in plankton species occur. Therefore, the deployment of alkalinity enhancement in zones with high mixing dynamics could meet the PNEC requirement. Taken together, the introduction of Ni through olivine-based OAE has the potential to shift the taxonomic composition of natural phytoplankton communities. Hence, the observed species-specific sensitivities towards the release of Ni underline that caution is needed in terms of magnitude and temporal mode (e.g., weekly, monthly, seasonal, and annual release) of ocean alkalization to alleviate the cumulative effects of Ni.
4.2 Implication for the deployment of ocean alkalinity enhancement
To prevent the potential ecological impacts of Ni in the process of OAE, Ni could be removed during the preparation of alkaline solutions. Numerous techniques that have been employed to remove Ni from wastewater could provide insights into the removal of Ni in olivine (Kadirvelu et al., 2001; Kim et al., 2002; Kalyani et al., 2004; Papadopoulos et al., 2004; Fu et al., 2007; Decostere et al., 2009). For example, chemical precipitation is an effective and the most widely used method in the industry. Though varying success has been achieved, these methods are associated with high costs, operational drawbacks, and the potential for secondary pollution (Fu and Wang, 2011). Nowadays Ni is a highly demanded metal resource for battery manufacture (Turcheniuk et al., 2021). A novel approach has been proposed to recover Ni for enhancing the supply of critical battery metals (Wang et al., 2018; Wang and Dreisinger, 2023). This technique could be useful in the context of OAE, contributing to the mitigation of ecological impacts on the one hand and reducing the costs of OAE application on the other. For OAE applications, minerals containing fewer heavy metals, such as quicklime produced from limestone, could also be considered (Gabe and Rodella, 1999; Šiler et al., 2018). These minerals could provide a viable option for the required application with less harmful elements introduced into the ocean (Bach et al., 2019; Caserini et al., 2022). The limestone is abundantly available and could meet the requirement for large-scale deployment of OAE. In addition, the economic costs for its extraction and transportation are relatively low, and the duration required for dissolution is shorter compared to olivine (Caserini et al., 2022; Fuhr et al., 2022). However, it is essential to acknowledge that the calcination of limestone demands a substantial amount of energy and necessitates proper capture and storage of the released CO2. To comprehensively assess the applicability and scalability of various material deployments, further investigation and research are warranted.
The goal of this study was to examine the response of three phytoplankton species representative of different taxonomic groups to the exposure of elevated Ni, which may occur in the process of OAE. The results demonstrated that the tested phytoplankton species exhibited varying responses to excess Ni. The diatom T. weissflogii displayed a high sensitivity to elevated Ni, evident from its rapid growth inhibition response, high growth inhibition, and low IC50 value. In contrast, the low growth inhibition and high IC50 values of A. carterae and E. huxleyi indicate that these two species are more tolerant to excess Ni. The variability in sensitivity to Ni among different species highlights the importance of avoiding critical toxic thresholds of Ni concentrations. The recovery of Ni from Ni-rich materials and the usage of alternative clean minerals would avoid adverse impacts on the phytoplankton community, enhancing the feasibility and scalability of ocean alkalization. In summary, the varying responses to Ni among different species make it clear that the impacts of Ni cannot be neglected and that caution is needed in setting the threshold for Ni in OAE applications with Ni-rich materials. Future studies focusing on the taxonomical shift in natural communities and on incorporation and potential bioaccumulation of Ni in different plankton species are planned to provide a more comprehensive understanding of the potential effects and risks of metal release associated with OAE.
The data are available at Pangaea (https://doi.org/10.1594/PANGAEA.964086, Xin et al., 2023).
The supplement related to this article is available online at: https://doi.org/10.5194/bg-21-761-2024-supplement.
XX and UR designed the experiments and XX carried them out. XX conducted statistical analyses and prepared the paper with contributions from all authors.
The contact author has declared that none of the authors has any competing interests.
Publisher’s note: Copernicus Publications remains neutral with regard to jurisdictional claims made in the text, published maps, institutional affiliations, or any other geographical representation in this paper. While Copernicus Publications makes every effort to include appropriate place names, the final responsibility lies with the authors.
This article is part of the special issue “Environmental impacts of ocean alkalinity enhancement”. It is not associated with a conference.
We gratefully acknowledge the technical support of Mathias Haunost and Tim Steffens in this study and the invaluable comments and discussions with Fengjie Liu, Jan Taucher, and Markus Schartau on the draft. We also thank Birte Matthiessen and Julia Romberg for kindly providing the A. carterae culture.
This study was supported by the German Federal Ministry of Education and Research (grant no. 03F0895) Project RETAKE, in the framework of the DAM Mission “Marine carbon sinks in decarbonization pathways (CDRmare)”. The study also received funding from the Carbon to Sea Initiative via the project OCEAN ALK-ALIGN. Xiaoke Xin was financially supported by the China Scholarship Council (CSC) (grant no. 201909370067).
The article processing charges for this open-access
publication were covered by Carbon to Sea Initiative.
This paper was edited by Jaime Palter and reviewed by two anonymous referees.
Achterberg, E. P. and Van Den Berg, C. M. G.: Chemical speciation of chromium and nickel in the western Mediterranean, Deep-Sea Res. Pt. II, 44, 693–720, https://doi.org/10.1016/s0967-0645(96)00086-0, 1997.
Allan, R. P., Hawkins, E., Bellouin, N., and Collins, B., IPCC, 2021: Summary for Policymakers, In: Masson-Delmotte, V., Zhai, P., Pirani, A., Connors, S. L., Péan, C., Berger, S., Caud, N., Chen, Y., Goldfarb, L., Gomis, M. I., Huang, M., Leitzell, K., Lonnoy, E., Matthews, J. B. R., Maycock, T. K., Waterfield, T., Yelekçi, O., Yu, R. and Zhou, B. (eds.) Climate Change 2021: The Physical Science Basis. Contribution of Working Group I to the Sixth Assessment Report of the Intergovernmental Panel on Climate Change, Cambridge University Press, 3–32, https://doi.org/10.1017/9781009157896.001, 2021.
Amann, T., Hartmann, J., Struyf, E., de Oliveira Garcia, W., Fischer, E. K., Janssens, I., Meire, P., and Schoelynck, J.: Enhanced Weathering and related element fluxes – a cropland mesocosm approach, Biogeosciences, 17, 103–119, https://doi.org/10.5194/bg-17-103-2020, 2020.
Bach, L. T., Gill, S. J., Rickaby, R. E., Gore, S., and Renforth, P.: CO2 removal with enhanced weathering and ocean alkalinity enhancement: potential risks and co-benefits for marine pelagic ecosystems, Front. Clim., 1, 1–21, https://doi.org/10.3389/fclim.2019.00007, 2019.
Barka, S., Pavillon, J. F., and Amiard, J. C.: Influence of different essential and non-essential metals on MTLP levels in the copepod Tigriopus brevicornis, Comp. Biochem. Phys. C, 128, 479–493, https://doi.org/10.1016/S1532-0456(00)00198-8, 2001.
Bartha, R. and Ordal, E.: Nickel-dependent chemolithotrophic growth of two Hydrogenomonas strains, J. Bacteriol., 89, 1015–1019, https://doi.org/10.1128/jb.89.4.1015-1019.1965, 1965.
Blaby-Haas, C. E. and Merchant, S. S.: The ins and outs of algal metal transport, BBA-Mol. Cell. Res., 1823, 1531–1552, https://doi.org/10.1016/j.bbamcr.2012.04.010, 2012.
Brand, L. E., Sunda, W. G., and Guillard, R. R.: Reduction of marine phytoplankton reproduction rates by copper and cadmium, J. Exp. Mar. Biol. Ecol., 96, 225–250, https://doi.org/10.1016/0022-0981(86)90205-4, 1986.
Canterford, G. and Canterford, D.: Toxicity of heavy metals to the marine diatom Ditylum brightwellii (West) Grunow: correlation between toxicity and metal speciation, J. Mar. Biolog. Assoc. U.K., 60, 227–242, https://doi.org/10.1017/S0025315400024280, 1980.
Caserini, S., Pagano, D., Campo, F., Abbà, A., De Marco, S., Righi, D., Renforth, P., and Grosso, M.: Potential of maritime transport for ocean liming and atmospheric CO2 removal, Front. Clim., 22, 575900, https://doi.org/10.3389/fclim.2021.575900, 2021.
Caserini, S., Storni, N., and Grosso, M.: The availability of limestone and other raw materials for ocean alkalinity enhancement, Global Biogeochem. Cy., 36, e2021GB007246, https://doi.org/10.1029/2021GB007246, 2022.
Croot, P. L., Moffett, J. W., and Brand, L. E.: Production of extracellular Cu complexing ligands by eucaryotic phytoplankton in response to Cu stress, Limnol. Oceanogr., 45, 619–627, https://doi.org/10.4319/lo.2000.45.3.0619, 2000.
Debelius, B., Forja, J. M., and Lubián, L. M.: Toxicity of copper, nickel and zinc to Synechococcus populations from the Strait of Gibraltar, J. Marine Syst., 88, 113–119, https://doi.org/10.1016/j.jmarsys.2011.02.009, 2011.
Decostere, B., Hogie, J., Dejans, P., and Van Hulle, S. W.: Removal of heavy metals occurring in the washing water of flue gas purification, Chem. Eng. J., 150, 196–203, https://doi.org/10.1016/j.cej.2008.12.025, 2009.
DeForest, D. K. and Schlekat, C. E.: Species sensitivity distribution evaluation for chronic nickel toxicity to marine organisms, Integr. Environ. Asses., 9, 580–589, https://doi.org/10.1002/ieam.1419, 2013.
Deleebeeck, N. M., De Schamphelaere, K. A., and Janssen, C. R.: Effects of Mg2+ and H+ on the toxicity of Ni2+ to the unicellular green alga Pseudokirchneriella subcapitata: Model development and validation with surface waters, Sci. Total Environ., 407, 1901–1914, https://doi.org/10.1016/j.scitotenv.2008.11.052, 2009.
Donat, J. R., Lao, K. A., and Bruland, K. W.: Speciation of dissolved copper and nickel in South San Francisco Bay: a multi-method approach, Anal. Chim. Acta., 284, 547–571, https://doi.org/10.1016/0003-2670(94)85061-5, 1994.
Dupont, C. L., Buck, K. N., Palenik, B., and Barbeau, K.: Nickel utilization in phytoplankton assemblages from contrasting oceanic regimes, Deep-Sea Res. Pt. I, 57, 553–566, https://doi.org/10.1016/j.dsr.2009.12.014, 2010.
Echeveste, P., Croot, P., and von Dassow, P.: Differences in the sensitivity to Cu and ligand production of coastal vs offshore strains of Emiliania huxleyi, Sci. Total Environ., 625, 1673–1680, https://doi.org/10.1016/j.scitotenv.2017.10.050, 2018.
Egleston, E. S. and Morel, F. M.: Nickel limitation and zinc toxicity in a urea-grown diatom, Limnol. Oceanogr., 53, 2462–2471, https://doi.org/10.4319/lo.2008.53.6.2462, 2008.
Feng, E. Y., Koeve, W., Keller, D. P., and Oschlies, A.: Model-Based Assessment of the CO2 Sequestration Potential of Coastal Ocean Alkalinization, Earth's Future, 5, 1252–1266, https://doi.org/10.1002/2017EF000659, 2017.
Fu, F. and Wang, Q.: Removal of heavy metal ions from wastewaters: a review, J. Environ. Manage., 92, 407–418, https://doi.org/10.1016/j.jenvman.2010.11.011, 2011.
Fu, F., Chen, R., and Xiong, Y.: Comparative investigation of N, N'-bis-(dithiocarboxy) piperazine and diethyldithiocarbamate as precipitants for Ni (II) in simulated wastewater, J. Hazard. Mater., 142, 437–442, https://doi.org/10.1016/j.jhazmat.2006.08.036, 2007.
Fuhr, M., Geilert, S., Schmidt, M., Liebetrau, V., Vogt, C., Ledwig, B., and Wallmann, K.: Kinetics of olivine weathering in seawater: an experimental study, Front. Clim., 4, 831587, https://doi.org/10.3389/fclim.2022.831587, 2022.
Gabe, U. and Rodella, A. A.: Trace elements in Brazilian agricultural limestones and mineral fertilizers, Commun. Soil. Sci. Plan., 30, 605–620, https://doi.org/10.1080/00103629909370231, 1999.
Gad, S. C.: Nickel chloride, Reference Module in Biomedical Sciences, Elsevier, https://doi.org/10.1016/B978-0-12-824315-2.00704-1, 2023.
Gaillardet, J., Viers, J., and Dupré, B.: Trace elements in river waters, Treatise Geochem., 5, 605, https://doi.org/10.1016/B0-08-043751-6/05165-3, 2003.
Gerringa, L., Herman, P., and Poortvliet, T.: Comparison of the linear van den Berg/Ružić transformation and a non-linear fit of the Langmuir isotherm applied to Cu speciation data in the estuarine environment, Mar. Chem., 48, 131–142, https://doi.org/10.1016/0304-4203(94)00041-B, 1995.
Gerringa, L., Rijkenberg, M., Slagter, H., Laan, P., Paffrath, R., Bauch, D., Rutgers van der Loeff, M., and Middag, R.: Dissolved Cd, Co, Cu, Fe, Mn, Ni, and Zn in the Arctic Ocean, J. Geophys. Res.-Oceans, 126, e2021JC017323, https://doi.org/10.1029/2021JC017323, 2021.
Gonzalez-Davila, M., Santana-Casiano, J. M., Perez-Pena, J., and Millero, F. J.: Binding of Cu (II) to the surface and exudates of the alga Dunaliella tertiolecta in seawater, Environ. Sci. Technol., 29, 289–301, https://doi.org/10.1021/es00002a004, 1995.
Guillard, R. R., and Ryther, J. H.: Studies of marine planktonic diatoms: I. Cyclotella nana Hustedt, and Detonula confervacea (Cleve) Gran, Can. J. Microbiol., 8, 229–239, https://doi.org/10.1139/m62-029, 1962.
Guo, J. A., Strzepek, R., Willis, A., Ferderer, A., and Bach, L. T.: Investigating the effect of nickel concentration on phytoplankton growth to assess potential side-effects of ocean alkalinity enhancement, Biogeosciences, 19, 3683–3697, https://doi.org/10.5194/bg-19-3683-2022, 2022.
Gustafsson J. P.: Visual Minteq (Version 3.1), KTH, Sweden [code], https://vminteq.lwr.kth.se/ (last access: 17 January 2024), 2013.
Hagopian-Schlekat, T., Chandler, G., and Shaw, T. J.: Acute toxicity of five sediment-associated metals, individually and in a mixture, to the estuarine meiobenthic harpacticoid copepod Amphiascus tenuiremis, Mar. Environ. Res., 51, 247–264, https://doi.org/10.1016/S0141-1136(00)00102-1, 2001.
Hall, L. W. and Anderson, R. D.: The influence of salinity on the toxicity of various classes of chemicals to aquatic biota, Crit. Rev. Toxicol., 25, 281–346, https://doi.org/10.3109/10408449509021613, 1995.
Hartmann, J., West, A. J., Renforth, P., Köhler, P., De La Rocha, C. L., Wolf-Gladrow, D. A., Dürr, H. H., and Scheffran, J.: Enhanced chemical weathering as a geoengineering strategy to reduce atmospheric carbon dioxide, supply nutrients, and mitigate ocean acidification, Rev. Geophys., 51, 113–149, https://doi.org/10.1002/rog.20004, 2013.
Hartmann, J., Suitner, N., Lim, C., Schneider, J., Marín-Samper, L., Arístegui, J., Renforth, P., Taucher, J., and Riebesell, U.: Stability of alkalinity in ocean alkalinity enhancement (OAE) approaches – consequences for durability of CO2 storage, Biogeosciences, 20, 781–802, https://doi.org/10.5194/bg-20-781-2023, 2023.
Horvatić, J. and Peršić, V.: The effect of Ni2+, Co2+, Zn2+, Cd2+ and Hg2+ on the growth rate of marine diatom Phaeodactylum tricornutum Bohlin: microplate growth inhibition test, B. Environ. Contam. Tox., 79, 494–498, https://doi.org/10.1007/s00128-007-9291-7, 2007.
Huang, X., Lin, X., Li, S., Xu, S., and Liu, F.: The influence of urea and nitrate nutrients on the bioavailability and toxicity of nickel to Prorocentrum donghaiense (Dinophyta) and Skeletonema costatum (Bacillariophyta), Aquat. Toxicol., 181, 22–28, https://doi.org/10.1016/j.aquatox.2016.10.027, 2016.
Hutchins, D. A., Fu, F.-X., Yang, S.-C., John, S. G., Romaniello, S. J., Andrews, M. G., and Walworth, N. G.: Responses of globally important phytoplankton species to olivine dissolution products and implications for carbon dioxide removal via ocean alkalinity enhancement, Biogeosciences, 20, 4669–4682, https://doi.org/10.5194/bg-20-4669-2023, 2023.
Ilyina, T., Wolf-Gladrow, D., Munhoven, G., and Heinze, C.: Assessing the potential of calcium-based artificial ocean alkalinization to mitigate rising atmospheric CO2 and ocean acidification, Geophys. Res. Lett., 40, 5909–5914, https://doi.org/10.1002/2013GL057981, 2013.
John, S. G., Kelly, R. L., Bian, X., Fu, F., Smith, M. I., Lanning, N. T., Liang, H., Pasquier, B., Seelen, E. A., and Holzer, M.: The biogeochemical balance of oceanic nickel cycling, Nat. Geosci., 1–7, https://doi.org/10.1038/s41561-022-01045-7, 2022.
Kadirvelu, K., Thamaraiselvi, K., and Namasivayam, C.: Adsorption of nickel (II) from aqueous solution onto activated carbon prepared from coirpith, Sep. Purif. Technol., 24, 497–505, https://doi.org/10.1016/S1383-5866(01)00149-6, 2001.
Kalyani, S., Rao, P. S., and Krishnaiah, A.: Removal of nickel (II) from aqueous solutions using marine macroalgae as the sorbing biomass, Chemosphere, 57, 1225–1229, https://doi.org/10.1016/j.chemosphere.2004.08.057, 2004.
Karthikeyan, P., Marigoudar, S., and Mohan, D.: Toxicity of nickel on the selected species of marine diatoms and copepods, B. Environ. Contam. Tox., 100, 331–337, https://doi.org/10.1007/s00128-018-2279-7, 2018.
Kester, D. R., Duedall, I. W., Connors, D. N., and Pytkowicz, R. M.: Preparation of artificial seawater 1, Limnol. Oceanogr., 12, 176–179, https://doi.org/10.4319/lo.1967.12.1.0176, 1967.
Kim, B., Gaines, W., Szafranski, M., Bernath, E., and Miles, A.: Removal of heavy metals from automotive wastewater by sulfide precipitation, J. Environ. Eng., 128, 612–623, https://doi.org/10.1061/(ASCE)0733-9372(2002)128:7(612), 2002.
Köhler, P., Hartmann, J., and Wolf-Gladrow, D. A.: Geoengineering potential of artificially enhanced silicate weathering of olivine, P. Natl. Acad. Sci. USA, 107, 20228–20233, https://doi.org/10.1073/pnas.1000545107, 2010.
Köhler, P., Abrams, J. F., Völker, C., Hauck, J., and Wolf-Gladrow, D. A.: Geoengineering impact of open ocean dissolution of olivine on atmospheric CO2, surface ocean pH and marine biology, Environ. Res. Lett., 8, 014009, https://doi.org/10.1088/1748-9326/8/1/014009, 2013.
Lee, J. G., Ahner, B. A., and Morel, F. M.: Export of cadmium and phytochelatin by the marine diatom Thalassiosira weissflogii, Environ. Sci. Technol., 30, 1814–1821, https://doi.org/10.1021/es950331p, 1996.
Mackey, D., O'sullivan, J., Watson, R., and Dal Pont, G.: Trace metals in the Western Pacific: temporal and spatial variability in the concentrations of Cd, Cu, Mn and Ni, Deep-Sea Res. Pt. I, 49, 2241–2259, https://doi.org/10.1016/S0967-0637(02)00124-3, 2002.
Martínez-Ruiz, E. B. and Martínez-Jerónimo, F.: Nickel has biochemical, physiological, and structural effects on the green microalga Ankistrodesmus falcatus: an integrative study, Aquat. Toxicol., 169, 27–36, https://doi.org/10.1016/j.aquatox.2015.10.007, 2015.
Meysman, F. J. and Montserrat, F.: Negative CO2 emissions via enhanced silicate weathering in coastal environment, Biol. Lett., 13, 20160905, https://doi.org/10.1098/rsbl.2016.0905, 2017.
Minx, J. C., Lamb, W. F., Callaghan, M. W., Fuss, S., Hilaire, J., Creutzig, F., Amann, T., Beringer, T., De Oliveira Garcia, W., Hartmann, J., Khanna, T., Lenzi, D., Luderer, G., Nemet, G. F., Rogelj, J., Smith, P., Vicente Vicente, J. L., Wilcox, J., and Del Mar Zamora Dominguez, M.: Negative emissions – Part 1: Research landscape and synthesis, Environ. Res. Lett., 13, 063001, https://doi.org/10.1088/1748-9326/aabf9b, 2018.
Montserrat, F., Renforth, P., Hartmann, J., Leermakers, M., Knops, P., and Meysman, F. J. R.: Olivine dissolution in seawater: implications for CO2 sequestration through enhanced weathering in coastal environments, Environ. Sci. Technol., 51, 3960–3972, https://doi.org/10.1021/acs.est.6b05942, 2017.
Morel, F. M., Hudson, R. J., and Price, N. M.: Limitation of productivity by trace metals in the sea, Limnol. Oceanogr., 36, 1742–1755, https://doi.org/10.4319/lo.1991.36.8.1742, 1991.
Moustakas, M., Moustaka, J., and Sperdouli, I.: Hormesis in photosystem II: a mechanistic understanding, Curr. Opin. Toxicol., 29, 57–64, https://doi.org/10.1016/j.cotox.2022.02.003, 2022.
Muyssen, B. T., Brix, K., DeForest, D., and Janssen, C.: Nickel essentiality and homeostasis in aquatic organisms, Environ. Rev., 12, 113–131, https://doi.org/10.1139/a04-004, 2004.
Olenina, I., Hajdu, S., Edler, L., Andersson, A., Wasmund, N., Busch, S., Göbel, J., Gromisz, S., Huseby, S., Huttunen, M., Jaanus, A., Kokkonen, P., Ledaine, I., and Niemkiewicz, E.: Biovolumes and size-classes of phytoplankton in the Baltic Sea HELCOM Balt, Sea Environ. Proc., 106, 144, 2006.
Panneerselvam, K., Marigoudar, S. R., and Dhandapani, M.: Toxicity of nickel on the selected species of marine diatoms and copepods, B. Environ. Contam. Tox., 100, 331–337, https://doi.org/10.1007/s00128-018-2279-7, 2018.
Papadopoulos, A., Fatta, D., Parperis, K., Mentzis, A., Haralambous, K. J., and Loizidou, M.: Nickel uptake from a wastewater stream produced in a metal finishing industry by combination of ion-exchange and precipitation methods, Sep. Purif. Technol., 39, 181–188, https://doi.org/10.1016/j.seppur.2003.10.010, 2004.
Pederson, D. M., Daday, A., and Smith, G. D.: The use of nickel to probe the role of hydrogen metabolism in cyanobacterial nitrogen fixation, Biochimie, 68, 113–120, https://doi.org/10.1016/S0300-9084(86)81076-8, 1986.
Pérez, P., Estévez-Blanco, P., Beiras, R., and Fernández, E.: Effect of copper on the photochemical efficiency, growth, and chlorophyll a biomass of natural phytoplankton assemblages, Environ. Toxicol. Chem., 25, 137–143, https://doi.org/10.1897/04-392R1.1, 2006.
Posit team: RStudio: Integrated Development Environment for R, Posit Software, PBC, Boston, MA [code], https://posit.co/ (last access: 17 January 2024), 2023.
Pörtner, H. O., Roberts, D. C., Adams, H., Adler, C., Aldunce, P., Ali, E., Begum, R. A., Betts, R., Kerr, R. B., and Biesbroek, R.: Climate change 2022: Impacts, adaptation and vulnerability, IPCC Sixth Assessment Report, https://doi.org/10.1017/9781009325844, 2022.
Price, N. M. and Morel, F. M. M.: Colimitation of phytoplankton growth by nickel and nitrogen, Limnol. Oceanogr., 36, 1071–1077, https://doi.org/10.4319/lo.1991.36.6.1071, 1991.
R Core Team: R: A language and environment for statistical computing, R Foundation for Statistical Computing, Vienna, Austria [code], https://www.r-project.org/ (last access: 18 January 2024), 2023.
Renforth, P.: The negative emission potential of alkaline materials, Nat. Commun., 10, 1401, https://doi.org/10.1038/s41467-019-09475-5, 2019.
Rogelj, J., Popp, A., Calvin, K. V., Luderer, G., Emmerling, J., Gernaat, D., Fujimori, S., Strefler, J., Hasegawa, T., and Marangoni, G.: Scenarios towards limiting global mean temperature increase below 1.5 ∘C, Nat. Clim. Change, 8, 325–332, https://doi.org/10.1038/s41558-018-0091-3, 2018.
Saito, M. A., Moffett, J. W., and DiTullio, G. R.: Cobalt and nickel in the Peru upwelling region: A major flux of labile cobalt utilized as a micronutrient, Global Biogeochem. Cy., 18, 1–14, https://doi.org/10.1029/2003GB002216, 2004.
Schuiling, R. and Krijgsman, P.: Enhanced weathering: an effective and cheap tool to sequester CO2, Climatic Change, 74, 349–354, https://doi.org/10.1007/s10584-005-3485-y, 2006.
Sclater, F. R., Boyle, E., and Edmond, J. M.: On the marine geochemistry of nickel, Earth Planet Sc. Lett., 31, 119–128, https://doi.org/10.1016/0012-821X(76)90103-5, 1976.
Shepherd, J., Caldeira, K., Cox, P., Haigh, J., Keith, D., Launder, D., Mace, G., MacKerron, G., Pyle, J., Rayner, S., Redgwell, C., and Watson, A.: Geoengineering the Climate: Science, Governance and Uncertainty, The Royal Society, London, UK, ISBN 9780854037735, 2009.
Šiler, P., Kolářová, I., Bednárek, J., Janča, M., Musil, P., and Opravil, T.: The possibilities of analysis of limestone chemical composition, IOP C. Ser-Mat. Sci., 379, 012033, https://doi.org/10.1088/1757-899X/379/1/012033, 2018.
Simkin, T. and Smith, J. V.: Minor-element distribution in olivine, J. Geol., 78, 304–325, https://doi.org/10.1086/627519, 1970.
Stephenson, G. L., Koper, N., Atkinson, G. F., Solomon, K. R., and Scroggins, R. P.: Use of nonlinear regression techniques for describing concentration – response relationships of plant species exposed to contaminated site soils, Environ. Toxicol. Chem., 19, 2968–2981, https://doi.org/10.1002/etc.5620191218, 2000.
Sunda, W. G.: Feedback interactions between trace metal nutrients and phytoplankton in the ocean, Front. Microbiol., 3, 204, https://doi.org/10.3389/fmicb.2012.00204, 2012.
Taylor, L. L., Quirk, J., Thorley, R., Kharecha, P. A., Hansen, J., Ridgwell, A., Lomas, M. R., Banwart, S. A., and Beerling, D. J.: Enhanced weathering strategies for stabilizing climate and averting ocean acidification, Nat. Clim. Change, 6, 402–406, https://doi.org/10.1038/nclimate2882, 2016.
Turcheniuk, K., Bondarev, D., Amatucci, G. G., and Yushin, G.: Battery materials for low-cost electric transportation, Mater. Today, 42, 57–72, https://doi.org/10.1016/j.mattod.2020.09.027, 2021.
Van den Berg, C. and Nimmo, M.: Determination of interactions of nickel with dissolved organic material in seawater using cathodic stripping voltammetry, Sci. Total Environ., 60, 185–195, https://doi.org/10.1016/0048-9697(87)90415-3, 1987.
Verriopoulos, G. and Dimas, S.: Combined toxicity of copper, cadmium, zinc, lead, nickel, and chrome to the copepod Tisbe holothuriae, B. Environ. Contam. Tox., 41, 378–384, https://doi.org/10.1007/BF01688882, 1988.
Walsh, M. J. and Ahner, B. A.: Copper export contributes to low copper levels and copper tolerance in Emiliania huxleyi, Limnol. Oceanogr., 59, 827–839, https://doi.org/10.4319/lo.2014.59.3.0827, 2014.
Wang, F. and Dreisinger, D. B.: Enhanced CO2 mineralization and selective critical metal extraction from olivine and laterites, Sep. Purif. Technol., 321, 124268, https://doi.org/10.1016/j.seppur.2023.124268, 2023.
Wang, F., Dreisinger, D. B., Jarvis, M., and Hitchins, T.: The technology of CO2 sequestration by mineral carbonation: current status and future prospects, Can. Metall. Quart., 57, 46–58, https://doi.org/10.1080/00084433.2017.1375221, 2018.
Xin, X., Faucher, G., and Riebesell, U.: Nickel toxicity to the dinoflagellate Amphidinium carterae, the coccolithophore Emiliania huxleyi, the diatom Thalassiosira weissflogii during laboratory experiments in 2021, PANGAEA [data set], https://doi.org/10.1594/PANGAEA.964086, 2023.
Yang, T., Chen, Y., Zhou, S., and Li, H.: Impacts of aerosol copper on marine phytoplankton: A review, Atmosphere-Basel, 10, 414, https://doi.org/10.3390/atmos10070414, 2019.
Zondervan I., Rost B., and Riebesell U.: Effect of CO2 concentration on the PIC/POC ratio in the coccolithophore Emiliania huxleyi grown under light-limiting conditions and different daylengths, J. Exp. Mar. Biol. Ecol., 272, 55–70, https://doi.org/10.1016/S0022-0981(02)00037-0, 2002.