the Creative Commons Attribution 4.0 License.
the Creative Commons Attribution 4.0 License.
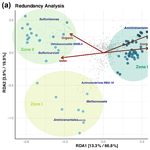
Microbial communities inhabiting 600-year-old sediments in the Inka-Coya Lake located in the Atacama Desert
Juan Castro-Severyn
Francisco Remonsellez
Antonio Maldonado
Inger Heine-Fuster
Hector Pizarro
Adriana Aránguiz-Acuña
Lacustrine sediments are natural archives for the surrounding area's biogeochemical dynamics; in particular, the isolation and extreme conditions in which desert lakes are located make them ideal study models for studying perturbations in the ecosystem. We aimed to study the microbial community dynamics in Inka-Coya Lake, located in the Atacama Desert, where active geological activity and the local mining industry influence biological dynamics in this ecosystem, as suggested for macroinvertebrates and plankton communities in the lake. In this study, we aimed to characterize the microbial communities that inhabit deep lacustrine sediments and their interaction with the surrounding environment. The results show that the microbial community from lacustrine sediments contains over 70 % unclassified organisms, highlighting this ecosystem's microbial taxonomic novelty. Our results indicate that the microbial communities cluster in three distinct zones: a superficial community, an intermediate and mixed community, and a more specialized anaerobic community in the deeper sediments. The microbial composition is dominated by chemoheterotrophic bacteria strongly associated with methane metabolism. Additionally, there is statistical evidence of strong correlations between particular taxa such as Sulfurimonadaceae, Metanoregulaceae, and Ktedonobacteroceae with elements like Cu, As, Fe, Ni, and V, and magnetic properties of the surrounding environment, evidencing the strong correlation between the surrounding geochemistry and microbial life that could be disrupted by the continuous mining activity in the area. Further detailed studies of the metabolic repertoire of these communities are necessary to understand the complex dynamics between microbial life and geochemical composition in this fragile and extreme environment.
- Article
(7369 KB) - Full-text XML
- BibTeX
- EndNote
The Atacama Desert is located on the driest part of the South American dry diagonal, which extends from 5° S on the west coast to almost 50° S on the east coast, over 4000 km with less than 200 mm mean annual rainfall (MAR). It is surrounded by the western slopes of the central Andes Cordillera between 15 and 30° S at elevations between sea level and 3500 m a.s.l. The Andes Cordillera represents a physical barrier that directly modulates climatic conditions and water availability in the Atacama Desert (Garreaud et al., 2003). Particular environmental conditions of the Atacama, such as high solar radiation, low atmospheric humidity, and other pressures associated with the natural composition of the desert, directly influence life occurring in these ecosystems (Demergasso et al., 2008; Albarracín et al., 2020; Kurth et al., 2021; Kereszturi et al., 2020; Borsodi et al., 2022). Thus, the Atacama Desert waterbodies are truly natural laboratories for understanding evolutionary processes, not only of the geomorphology of the landscape but also of different life forms promoted by environmental forces, such as climate changes at different timescales (Adrian et al., 2009).
Aquatic sediments are sources and/or sinks of elements participating in biogeochemical cycles, including both allochthonous and autochthonous lake processes, influencing the biodiversity and trophic dynamics of waterbodies (Trolle et al., 2010; Fernández et al., 2016; Usenko et al., 2007; Bandowe et al., 2018). Aquatic sediments have an advantage over terrestrial records, which is that they accumulate at measurable rates because they are often buffered from physical, chemical, and biotic disturbances, thus allowing the recording of past environmental conditions (Benito, 2020; Picard et al., 2022; Da Costa et al., 2023; Yan et al., 2024). A wide variety of abiotic (e.g., bulk density, dry mass, radioactive isotopes, mineralogy, chemical elements) and biotic proxies (e.g., fossils, species abundance, species presence/absence, resting structures, pigments, environmental DNA) preserved in the sediments are currently analyzed to reconstruct ecosystem change at timescales ranging from the fine scale (interannual or decadal) to the millennial scale (Cohen et al., 2023; Korosi et al., 2017).
The central Andes water systems have mainly originated after successive glaciations and volcanic and tectonic activity. In the Atacama Desert, lacustrine sediments are natural archives holding evidence of past precipitations, dust deposition, anthropic disturbances, and pollution, mainly due to mining activity that occurs in the area (Grosjean and Veit, 2005; Placzek et al., 2009; Cerda et al., 2019; Aránguiz-Acuña et al., 2020). Desert lakes are located in extremely arid and isolated areas, making them susceptible to perturbations (Valero-Garcés et al., 2003; Pueyo et al., 2011; Grosjean and Veit, 2005) and hosting extreme forms of life, especially microorganisms that have evolved physiological and life-history adaptations that allow them to thrive in challenging conditions (Dib et al., 2008; Ordoñez et al., 2009; Farías et al., 2014; Rasuk et al., 2014). Therefore, lacustrine sediments are expected to contain a great taxonomic diversity, including low-abundance and highly specialized taxa, directly influenced by small-scale conditions determining local environments (Borsodi et al., 2022).
Inka-Coya Lake (22°20′ S, 68°35′ W; 2534 m a.s.l.) is located at the eastern margin of the Atacama Desert, close to the Salado River, in the San Francisco de Chiu-Chiu village, northern Chile. It is a karstic sinkhole developed during the Quaternary period by the dissolution of calcareous layers of the Chiu-Chiu Formation (El Loa Group). The Atacama Desert and its surroundings have a particular geological history; metals and metalloids found in the Inka-Coya area include Ti, Al, Fe, Ni, and Cr; also, As and Sb are associated with the local geological activity (Aszalós et al., 2020; Borsdorf and Stadel, 2015; Pérez-Portilla et al., 2024) that directly influences the chemical composition of the underground water (Vignale et al., 2021). The central Atacama Desert, specifically the Antofagasta Region, holds large porphyry copper deposits that support the great metal-mining industry (Dittmar, 2004; Salvarredy-Aranguren et al., 2008). In 2021, Chile was the world's top copper producer, producing 5 508 084 t, which is 26.6% of the world's production (Rodríguez-Luna et al., 2022). The mining industry in the Antofagasta region has developed extensively since the 19th century (Dittmar, 2004; Salvarredy-Aranguren et al., 2008) with increasing impacts on the national economy and development but also on the health of ecosystems, which have triggered social and environmental conflicts, affecting especially relevant groups such as ancient Indigenous communities from the Quechua and Lickan Antay people (see Ramírez et al., 2005; Tapia et al., 2019).
Previous studies that aimed to assess mining pollution records on environmental matrices have included the sediment records of different longitudes from the Inka-Coya Lake, showing variation through the geochemistry and magnetic properties (Cerda et al., 2019; Aránguiz-Acuña et al., 2020; Pérez-Portilla et al., 2024). Analyzed variables have allowed us to identify episodes associated with changes in water availability, flash flooding, and evidence of perturbations induced by mining activities. Overall, the lake is polluted at different degrees of severity with Cu, Sb, Mo, and As, and some elements like Cu and Ni have been enriched in the most recent periods (Cerda et al., 2019; Pérez-Portilla et al., 2024). Additionally, some biological proxies, such as macroinvertebrates and diatom communities, were found to be directly influenced by the accumulation of metals and metalloids as observed by changes in assembly composition (Aránguiz-Acuña et al., 2020). Surrounding metal-mining exploitation, which has been maintained and even increased through the last 200 years, in addition to stable-aridity conditions, makes Inka-Coya Lake an excellent site for understanding biological adaptations of aquatic populations to these anthropic pressures (Aránguiz-Acuña et al., 2018, 2020). Regarding microbial communities, previous studies have established that methanogens are abundant in cold desert lakes, and microorganisms inhabiting these lake oases are the basis for many ecological services (Cavicchioli, 2006; Stoeva et al., 2014; Olilo et al., 2023). Additionally, salinity and pH are the major drivers determining microbial community structure in other desert lakes (Casamayor et al., 2013; Banda et al., 2021; Santini et al., 2022). Therefore, it is necessary to establish a baseline regarding the dynamics between the microbial life and geochemical conditions in Inka-Coya Lake as a model for the Atacama Desert.
While microbial life in arid ecosystems plays a key role in maintaining biogeochemical cycles (Madsen, 2011), there is a high proportion of unclassified taxa that hold great interest in poly-extreme environments from an ecological, environmental, and biotechnological point of view (Farias et al., 2014; Castro-Severyn et al., 2021; Dong et al., 2022). Nevertheless, there are few records in which microbial assemblage has been used as a paleolimnological proxy for the possible responses to long-term sustained anthropogenic metal stress (Da Costa et al., 2023; Yan et al., 2024). The few studies considering this aim have focused on changes in primary producers' abundance, under-interpreting the impact on other metabolic functional groups (Benito, 2020; Picard et al., 2022).
This study aimed to characterize the microbial community along a lacustrine sediment core, capturing the depositional history of the Inka-Coya Lake over the last 600 years and to contribute to the knowledge of geo-microbial dynamics in the underexplored desert lakes as these microorganisms are the basis for most geochemical cycling of critical nutrients. We hypothesize that the microbial community assemblage of Inka-Coya Lake is strongly associated with sediment attributes shaped by autochthonous and allochthonous processes, particularly anthropogenic contributions from the metal-mining industry that has operated near the lake for the past century. To our knowledge, this is a pioneering study in the microbial characterization of a sedimentary core of this length (136 cm) and date (600 years) from lake sediment in the Atacama Desert. Results show that the microbial communities have changed through time, identifying three clear periods in which alpha and beta diversity has been associated with organic matter content, magnetic susceptibility, and metal and metalloid concentrations. Additional studies of the metabolic functions of the microorganisms inhabiting these sediments are required to understand the interactions between microbial life and the geochemical components of the Inka-Coya Lake further.
2.1 Study site and sampling
Inka-Coya Lake (San Francisco de Chiu-Chiu village, Antofagasta, Chile; 22°20.300′ S, 068°35.981′ W) has a surface area of 500 m2 and a maximum depth of 18 m, and it is located in the Preandean Depression of the Antofagasta Region at an elevation of 2520 m a.s.l. (Fig. 1a, b). Around the lake, the predominant vegetation is vegas, a type of wetland typical of the Andean pre-puna zone, strongly associated with the hydric variability in the emerging groundwaters.
2.2 Sediment core sampling
A fieldwork campaign was undertaken in August 2021. The topography of the lake bottom was modeled using the Echo-Map Plus 42CV from Garmin. Afterward, three sediment cores from the depocenter of Inka-Coya Lake, where maximum sedimentation rates are expected, were obtained. The cores were obtained using a 9.0 cm diameter Uwitec gravity corer. This study shows the most extended core analysis results, measuring 136 cm (labeled LIC-SHC03). X-ray and photography images were captured before obtaining sections of sediment subsamples from the core. For subsampling, the frozen core was sliced every 0.5 cm to a depth of 12 cm and then every 1 cm until the end of the core, totaling 146 sediment samples. Sediment subsamples for molecular analysis were first taken from each segment center within a laminar flow hood using ethanol-sterilized tools. These subsamples were collected in sterile 15 mL tubes, labeled and kept frozen. Other subsamples were also taken for the geochemical analyses. Additionally, subsamples for every 1 cm interval were obtained to develop the magnetic susceptibility analysis. The cores' detailed treatment, geochemical analysis, and magnetic properties can be reviewed in Pérez-Portilla et al. (2024).
2.3 Sediment core dating
The geochronology of the sediment core from Inka-Coya Lake was determined through radiocarbon dating (14C) on the remaining macroscopic carbon along the record. The measurements were done using accelerator mass spectrometry (AMS), and the results were corrected for isotopic fractionation with an unreported δ13C value. Subsequently, the age–depth model for this sedimentary core was established using the Bayesian radiocarbon chronology package Bchron in R, using the “shcal20” as the calibration curve (Hogg et al., 2020; Haslett and Parnell, 2008). A detailed description of the procedure is available in Pérez-Portilla et al. (2024).
2.4 Magnetic and geochemical properties analysis
A total of 5 g of each sediment subsample was placed into paleomagnetic boxes of 8 cm3 to measure the mass magnetic susceptibility (χ) using a Kappabridge MFK1_FA instrument (AGICO Co) under environmental conditions (22–24 °C) and a magnetic field of 200 A m−1. The samples were measured at a low frequency of 976 Hz (χlf or simply χ) and a high frequency of 15 616 Hz (χhf). The magnetic susceptibility dependent on the frequency was calculated using both measurements, as described by Pérez-Portilla et al. (2024). The χfd% parameter is used to indicate the presence of magnetic particles near the limit of the superparamagnetic/single domain (SP/SD) magnetic size (Verosub and Roberts, 1995), which can be linked to the presence of magnetic particles of authigenic origin (Dearing et al., 1996). Additionally, subsamples of each 1 cm slice were dried in an oven at 50 °C. Afterwards, they were homogenized using an agate mortar in the Geochemistry Laboratory of Universidad Católica del Norte (UCN), Antofagasta, Chile. The sediments were then digested using reverse aqua regia (4 mL HCl + 12 mL of HNO3+300 mg of sediment sample) and a microwave digester (Perkin Elmer MPS 320; EPA 3052 method), following Tapia et al. (2022), at the Centro de Investigación Tecnológica del Agua en el Desierto (CEITSAZA-UCN). The elements aluminum (Al), titanium (Ti), vanadium (V), manganese (Mn), iron (Fe), nickel (Ni), Cu, zinc (Zn), arsenic (As), molybdenum (Mo), and antimony (Sb) were measured by the inductively coupled plasma atomic emission spectroscopy (ICP-OES) Perkin Elmer Optima 7000 in the digested residue at CEITSAZA. The organic matter, inorganic matter, and carbonate contents were estimated using the loss-on-ignition (LOI) method, which was assessed at a contiguous 1 cm interval following Heiri et al. (2001). This procedure involved drying 1 cm3 of each sediment sample in crucibles at 105 °C for 2 h and weighing them. The dry samples were weighed before heating to 550 °C in a flask over 1.5 h, left at 550 °C for 2 h, and then allowed to cool. The samples were weighed, and then the crucibles were transferred to the flask and burned at 925 °C. Finally, the crucibles were weighed again once they cooled.
2.5 Sediment sample processing and DNA extraction
Sediment samples were obtained every 1 cm from the top to the bottom of the core under sterile conditions within a laminar flow hood using ethanol-sterilized tools. According to the manufacturer's instructions, total DNA was extracted from the 250 mg of sediment samples using the DNeasy PowerSoil kit (Qiagen Inc., Hilden, Germany). DNA integrity, quality, and quantity were verified by 1 % agarose gel electrophoresis, the 260/280 nm ratio, and fluorescence using a Qubit4 fluorometer and the Qubit dsDNA HS assay kit (Thermo Fisher Scientific, MA, USA). Subsequently, DNA samples were sent to AUSTRAL-omics, Chile, for amplification of the bacterial 16S rRNA gene V4 region (∼450 bp) using tBakt_341F and Bakt_805R primers (Herlemann et al., 2011), for the construction of 250 bp paired-end libraries, and for the sequencing on a MiSeq (Illumina) platform.
2.6 Taxonomic composition analysis
This analysis was conducted in R v4.0.3 and RStudio v1.3.1093 following the DADA2 v1.16.0 R package pipeline (Callahan et al., 2016) to infer amplicon sequence variants (ASVs) for each subsample. Briefly, the reads were evaluated for quality control and subsequently trimmed (Ns = 0, length ≥ 150 bp, expected errors ≤2), followed by dereplication, denoising, and merging of paired reads. Subsequently, an ASV table was built to allow a maximum of two expected errors, removing chimeras and assigning taxonomy using the SILVA v138 database (Quast et al., 2012). Also, all ASVs identified as Eukarya, chloroplast, and mitochondria were removed. In addition, ASVs with very low abundance (< 0.0005 %) and those only observed in a few samples (< 10 %) were eliminated; samples with very few reads (< 1000) were eliminated as well. Next, a multi-sequence alignment was created to infer phylogeny using FastTree v2.1.10 (Price et al., 2009), phyloseq-object (containing the ASVs, taxonomy assignment, phylogenetic tree, and the sample metadata) was created using the R package Phyloseq v1.34.0 (McMurdie and Holmes, 2013), and the variance stabilizing transformation was used for normalization. Finally, taxa relative abundance and taxonomic composition at different ranks were visualized using the ggplot2 v3.3.3 (Wickham, 2016), Fantaxtic v0.2.0 (Teunisse, 2022), and ampvis2 v2.7.4 (Andersen et al., 2018) R packages.
2.7 Diversity analysis
Alpha diversity metrics (Shannon, Chao, phylogenetic diversity, and Simpson indexes) were calculated for each segment along the core using the microbiome v1.24.0 (Lahti et al., 2017) and btools v0.0.1 R packages. Also, Wilcoxon statistical tests to compare means with Bonferroni correction were carried out between the identified zones and visualized using the DESeq2 v1.42.0 (Love et al., 2014) and ggpubr v0.6.0 (Kassambara, 2017) packages. Moreover, beta diversity was evaluated by principal coordinate analysis using Hellinger-transformed Bray–Curtis distances based on the ASV abundance matrix, which were calculated using the Phyloseq v1.34.0 (McMurdie and Holmes, 2013) and ampvis2 v2.4.5 (Andersen et al., 2018) R package. Also, redundancy analysis (RDA) was calculated using depth gradient and zone parameters to constrain the multivariate space, and ANOVA tested the statistical significance of the selected geochemical variables.
2.8 Functional predictions
Functional potential signatures and metabolic pathways abundances were inferred based on the ASV abundance and taxonomy matrices using PICRUSt2 v2.4.1 software (Douglas et al., 2020) with the Kyoto Encyclopedia of Genes and Genomes (KEGG) (Kanehisa et al., 2012) and MetaCyc (Caspi et al., 2018) pathway databases. We used the three identified zones along the core that present significant differences at the taxonomic composition level, and then the differential abundance of inferred pathways was tested with the Kruskal–Wallis test (confidence interval = 0.95) and the Benjamini–Hochberg corrected false discovery rate using the ggpicrust2 v1.7.2 R package (Yang et al., 2023). Also, we use the Functional Annotation of Prokaryotic Taxa (FAPROTAX) database v1.2.7 (Louca et al., 2016) to map the identified ASVs and quantify changes in established ecologically relevant functions.
The samples analyzed correspond to a sediment core from Inka-Coya Lake, located in the Atacama Desert, with a water depth of 18.5 m (Fig. 1). The sediment core age–depth model was constructed based on six charcoal sample dates, where the more superficial at 41 cm corresponds to 75±32 cal years BP, and the deepest found at 94 cm corresponds to an age value of 505±22 cal years BP. Based on the age–depth model constructed, the sediment core of Inka-Coya Lake analyzed had 630 years of age (Fig. 2).
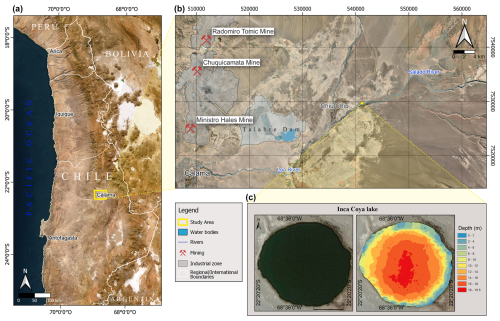
Figure 1Sampling site location in northern Chile (a), Inka-Coya Lake and important surrounding mining and urban centers (b), and the bathymetry of the lake (c).
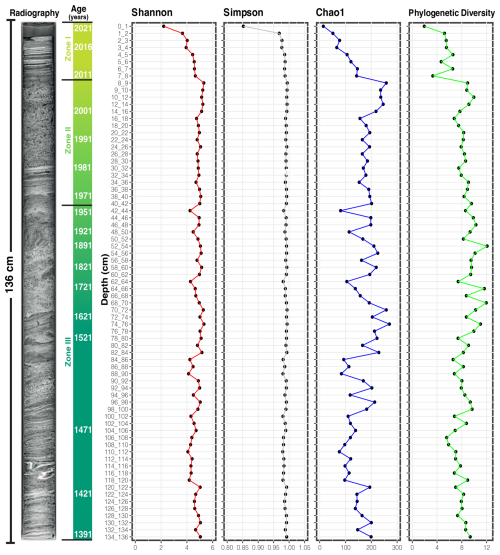
Figure 2Diversity of the microbial community of the Inka-Coya sediment core. The core sedimentary radiography, dating, and alpha diversity index variation along the core depth are displayed.
The variation in diversity within the sample was measured to determine the changes in the microbial community along the sediment core by calculating the Shannon, Simpson, Chao, and Phylogenetic indices as standard measures of the taxonomic diversity within a sample (Thukral, 2017). The microbial community observed along the core was diverse, based on the DNA samples analyzed. The quantification of diversity showed that it increased with depth, and the Simpson index remained stable after the 2 cm surface layer. At the same time, Chao1 considered low-abundance taxa and the phylogenetic index based on the phylogenetic history of the species (Fig. 2). The diversity increases in all cases at 2 cm length, and maximum values were observed between 8 and 86 cm, with minor peaks at 96, 120, and 130 cm near the bottom samples.
Three distinct zones in terms of microbial taxonomy could be identified and are statistically different for the four evaluated diversity indices, except between Zones II and III in the context of phylogenetic diversity (Fig. 3a). These three disjunct clustering zones of microbial community diversity along the sediment core were also identified in the beta diversity analysis, in which Zone I including the less diverse upper layer (0–8 cm), Zone II the middle zone of the core (9–42 cm) with significantly greater diversity, and Zone III the deepest are sampled in this study, depicted in three shades of green going from lighter (Zone I) to darker (Zone III) in the principal coordinate analysis (PCoA) clustering analysis (Fig. 3b).
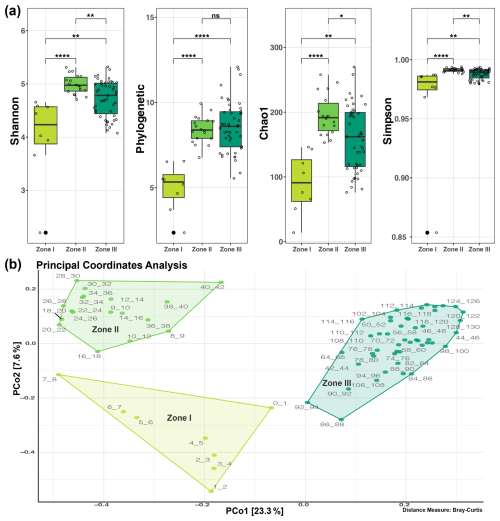
Figure 3Clustering of the microbial communities inhabiting the deep sediment of Inka-Coya Lake. (a) Statistical differences in alpha diversity between depth zones. (b) Principal coordinate analysis (PCoA) with the ASVs' relative abundance using Bray–Curtis as the distance metric; each point corresponds to a community, tagged by depth and colored by zone.
The taxonomic composition and abundance along the communities at phylum rank also reflect the clustering in three distinct zones, where Zone I is dominated by Actinobacteriota and includes a great abundance of Firmicutes in the top layers and Campylobacterota; there is also the presence of Bacteroidota, Halobacteriota, and Planctomycetota. Zone II is more diverse and composed mainly of Campylobacterota, Chloroflexi, Acidobacteriota, and Actinobacteriota. While Zone III is the largest and more homogeneous, composed of several low-abundance taxa, dominated by Chloroflexi, Acidobacteriota, and Actinobacteriota, there is also a higher representation of Crenarchaeota, Nitrospira, Aenigmarchaeota, and Armatimonadota than in the rest of the zones (Fig. 4). The taxonomic composition along the sediment core provides evidence of the metabolic adaptions that occur in each layer that presents endogenous characteristics. For instance the upper layer includes generalist groups like microorganisms that would break down complex organic groups and light-driven metabolic pathways, and they have the metabolic flexibility that would allow the use of different electron acceptors for energy production, supporting heterotrophic communities and mutualistic dynamics. On the other hand, Zone III includes more adaptable and ancient phylogenetic lineages that also have the ability to maintain geochemical cycles (C, N, S, Fe) in low-oxygen or anaerobic conditions, like those found in early Earth.
Notably, 76.6 % of the taxa could not be identified at the genus level (0 % matched any known species). Thus, Fig. 5 shows the abundance at the “best hit,” where Campylobacterota (Sulfuricurvum, Sulfurimonas), Mycobacterium, and Methanolinea dominated the overall community and where many representatives of chemo-lithotrophic, S-oxidizing, and nitrate-reducing capabilities would thrive, while in each zone, there are particular taxa associated with it. For instance, species belonging to the Aminicenantales phylum are very common in Zone I; Pseudarcobacter is prevalent in Zone II like the anaerobic bacterium Pelolinea is in Zone III.
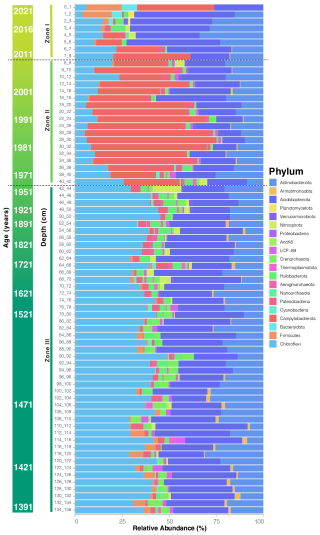
Figure 4Taxonomic composition of the microbial community in the deep sediments of Inka-Coya Lake. Stacked bar of the taxonomic composition at the phylum level.
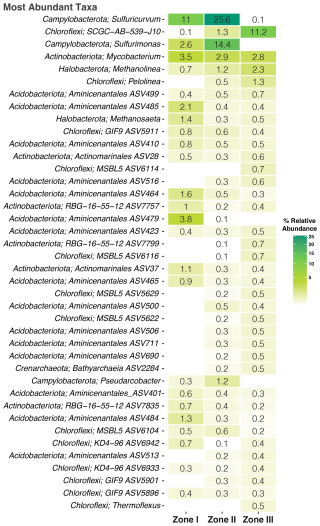
Figure 5Heatmap of the abundance of the microbial community at the family level. The color gradient indicates the abundance of the specific taxa.
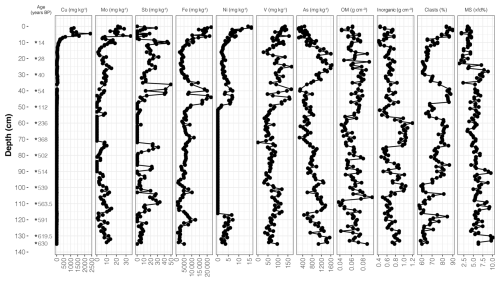
Figure 6Depth variation in metal and metalloid concentrations (mg kg−1), organic matter (OM) and inorganic matter concentrations (g cm−3), clasts percent, and magnetic susceptibility (MS) measured in the Inka-Coya Lake sediment core (modified from Pérez-Portilla et al., 2024).
Variations in analyzed sediment properties along the core, such as magnetic susceptibility, organic matter, and carbonate-clast composition, are shown in Fig. 6. Copper (Cu) and nickel (Ni) were in lower concentrations and variability at greater depths and showed concentration peaks in surface sediments. Elements such as iron (Fe), molybdenum (Mo), and vanadium (V) also showed top sediment peaks. Still, overall, they had more variable behavior than previously mentioned elements in the middle and bottom sediments (> 40 cm). Metalloids, arsenic (As), and antimony (Sb) exhibited the highest concentrations between 10–45 cm depth.
The mean organic matter and carbonate contents are around 8.7 % and 19.5 %, respectively, while the inorganic density (91 %) showed the highest averaged values (0.71 g cm−3). The sediment composition shifts to clay and silt from 28 cm to the top of the core. The content of clasts was predominant in the inorganic fraction, with 71.8 % along the core. The carbonate peaks were observed at 10–36 cm and 46–56 cm, but there were more significant and variable proportions below 96 cm, which did not exceed 40 %.
Magnetic susceptibility (MS, χ) values range from to m3 kg−1, with an average value of m3 kg−1. Frequency-dependent susceptibility (χfd%) values range between 1.31 % and 10.17 %, with an average of 5.62 %. Zone I has the highest values of χ and the lowest values of χfd%, while Zone II shows the lowest χ values and intermediate χfd% values. Zone III presents intermediate χ values and the highest χfd% values.
Geochemical and magnetic variables are associated with microbial diversity found in the sediment of Inka-Coya Lake, differentiated into zones (I, II, and III). Deeper and older fractions of sediments (dark blue), especially the Aminicenantales phylum, are positively influenced by the magnetic susceptibility and inorganic elements in the sediments. Microbial assemblage found in the middle sediments (Zone II) of the core is driven by organic matter content and water availability, where taxa like Sulfurimonas, Sulfuricurvum, and Dehalococcoidia were the most represented. The middle zone is associated with a significant As peak, which suggests that the presence of metals and/or metalloids positively affects the microorganism assemblage inhabiting the middle to superficial layers. In Zone II, microbial diversity is mainly associated with low but stable concentrations of organic matter, a more significant proportion of clasts, and the higher peak of As and Sb in the sediments, where χ values decreased. Upper Zone I is mainly characterized by metal enrichment, with elevated concentrations of Cu, Zn, Ni, Fe, and Mo, among other elements. These peaks correlate with high χ values (Fig. 7).
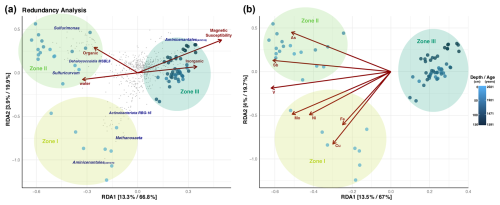
Figure 7Redundancy analysis on Hellinger-transformed Bray–Curtis distances (corrected by unobserved species) for the microbial communities along the core distance. (a) Influence of physicochemical parameters and (b) elemental composition. Depth gradient and zone parameters were chosen to constrain the multivariate space in a supervised approach. Each axis in the graph shows the percentage of variance explained in an unsupervised and supervised analysis.
There is statistical significance between some key taxa and the physicochemical and elemental composition along the sediment gradient in Inca-Coya Lake; for instance, Methanoregulaceae, Ktedonobacteriaceae, and Sulfurimonadaceae are some of the taxa with the strongest correlation to the presence of Cu, Fe, Ni, and V, while Zones II and III are the most influenced by these dynamics (Fig. A1).
Regarding metabolic approximation, chemoheterotrophy is the most abundant function in all three zones, while aerobic chemoheterotrophs are the most prevalent in Zone II. Still, chemoheterotrophs (including several electron acceptors) thrive in Zones II and III (Fig. B1). Other functions, such as methanogenesis, were abundant only in deep sediments (Zone III). Moreover, as expected, phototrophy and photoautotrophy were present only in low abundance in Zone I, where little light could reach the community (Fig. B1). Methane metabolism is very relevant in all three zones, especially in Zone III, where acetate is the primary source for this pathway (Fig. B1). Other relevant forms of energy transformation are nitrate reduction VI (assimilation) and starch degradation in Zone II. Nitrotoluene degradation and biosynthesis of unsaturated fatty acids are also crucial in the benthic microbial community as a whole (Fig. B1). This is a somewhat homogeneous prediction for metabolic ability regarding energy production among microorganisms inhabiting sediments more than 1 m deep in the Inka-Coya Lake sediments, where geochemical and magnetic dynamics directly influence microbial activities.
3.1 Discussion
Microbial dynamics along the length of the sediment core of Inka-Coya Lake are tightly associated with analyzed sediment attributes, such as metal and metalloid concentrations and χfd%; organic compounds; and water availability. The geochemical characteristics of the area surrounding Inka-Coya Lake, where active volcanic activity results in the enrichment of elements such as arsenic, sulfur, copper, and others (Romero et al., 2003; Tapia et al., 2018), suggest that microbial assemblages inhabiting the Atacama area (1) have showed structure changes during the last 600 years and (2) have developed a broad tolerance range to these potential toxic compounds. Microbial biomarkers serve as criteria to assess anthropogenic impact (Yan et al., 2024), and microorganisms can alter the speciation and bioavailability of metals and metalloids in an ecosystem (Niu et al., 2020).
Five stratigraphic zones in the sedimentary core in Inka-Coya Lake were defined from the sediment core here analyzed by Pérez-Portilla et al. (2024). In this sedimentary core, the concentrations of rock-forming elements such as Cr, Zn, and V are found in concentrations expected for the Atacama Desert, while Cu, Mo, Sb, and As are higher than expected, suggesting influence from nearby mining activities (Pérez-Portilla et al., 2024). Copper production in the region generates by-products such as Mo, As, and to a lesser extent Zn (Ramírez et al., 2005; Tapia et al., 2019). Also, mining wastes contain high concentrations of chemical products such as Pb, Cr, Cd, Cu, Zn, Hg, and Ni and metalloids, which are often stored in dams or reservoirs (Csavina et al., 2012) or passed through lotic systems, making them an important source of contamination of inorganic chemical elements for the aquatic biological communities (Keller et al., 1992; Pollard et al., 2003; Pigati et al., 2011; Hamilton et al., 2017; Ritter et al., 2019). Previous studies have shown impacts and metal enrichment from the mining industry in the Antofagasta region surrounding the city of Calama and the Loa River basin near the Inka-Coya Lake. Cerda et al. (2019), Vargas-Machuca et al. (2021), Aránguiz-Acuña et al. (2020), and Zanetta-Colombo et al. (2022, 2024), using both abiotic and biological proxies, have provided evidence of an increase in the concentration of metals in different environmental matrices during post-industrial times, attributing this difference to the mining activities in the area. Additionally, changes in the composition of the zooplankton community (inferred by diapausing egg banks) and benthic diatoms could be attributed to the increase in Cu concentration evidenced in the sedimentary cores obtained in this lake (Aránguiz-Acuña et al., 2020). It is therefore to be expected that other components of the aquatic community, which are as or more sensitive than planktonic invertebrates to changes in metal concentrations in the environment, may also be affected by the impacts of increases in metals associated with the development of the mining industry.
A strong correlation between mineral composition and microbial diversity in other arid region waterbodies, such as salt flats and brines, is expected (Farías et al., 2014; Castro-Severyn et al., 2021; Dong et al., 2022), as demonstrated in pre-puna salt lakes, such as Tebenchique and La Brava (Farías et al., 2014; Ramos-Tapia et al., 2023). The diversity of microbial life in these shallow salty lakes is dominated by Bacteroidetes, Proteobacteria, and Euryarchaeota (Farías et al., 2014; Fernandez et al., 2016; Kurth et al., 2021), and hypersaline lakes are mainly composed of Bacteroidetes, Chloroflexi, Cyanobacteria, and Proteobacteria (Dorador et al., 2018). Inka-Coya Lake, one of the few brackish water lakes located in the Antofagasta Region below 3000 m a.s.l., and its sediment communities are dominated by the phyla Acidobacteriota, Chloroflexi (Pelolinea, among others), Actinobacteriota (Mycobacterium), and Campylobacterota (Sulfuricurvum and Sulfurimonas), sharing some similarities when considering changes in taxonomy (Oren and Garrity, 2021). Overall, desert lake sediment microbial communities are very diverse, accounting for the variable conditions and different niches that microorganisms can utilize. In this study, we found great taxonomic diversity and potential metabolic richness where several electron acceptors would sustain the community, including complex carbon complexes, nitrogen, sulfur, and iron, each in different oxidation states. Heterotrophic and autotrophic aerobes and anaerobic representatives were found along the length of the sediment core, indicating that mutualistic relationships are vital for the maintenance of the ecosystem and ecological functions.
At the lower taxonomic rank, the community is dominated by microorganisms with a broad repertoire for mineral interactions; e.g., there is experimental evidence of organomineralization in extracellular S0 formation by a species of the sulfur-oxidizing bacteria Sulfuricurvum (Cron et al., 2019). Another remarkable microorganism found along the lacustrine sediment is the cosmopolitan and highly diverse Sulfurimonas, which can grow using sulfur, hydrogen, nitrogen, oxygen, and organic compounds, suggesting it is critical in maintaining trophic dynamics (Han and Perner, 2015). Additionally, Mycobacterium is a saprophytic bacterium commonly found in lakes, rivers, and other water sources (Falkinham, 2015); there are some species representatives of this genus that have bioremediation potential for polycyclic aromatic hydrocarbons (Deng et al., 2023), suggesting adaptability and a broad range of metabolic capacities. There are extensive studies on Mycobacteriaceae representatives that cause human disease, as reviewed by Falkinham III (2009). However, environmental representatives with the capacity to inhabit poly-extreme environments have yet to be determined.
Furthermore, several representatives of the candidate phylum Aminicenantales are common in current sediments from Zone I, i.e., recently deposited sediments during the last 10 years. So far, these microorganisms are associated with a fermentative saccharolytic lifestyle that does not have an isolated representative yet (Kadnikov et al., 2019). Zone II, comprising the period between 10 and 50 years ago approximately, is mainly represented by microorganisms classified as the Pseudarcobacter genus that was recently separated from the Arcobacter genus (Pérez-Cataluña et al., 2018) and is characterized as a mesophilic bacteria that can grow in microaerophilic conditions (Collado et al., 2011). Finally, in the deeper and older sediments, where anaerobic (or facultative anaerobes) microorganisms can thrive, there is a particular abundance of an ASV from the Pelolinea genus that has only one described species that was isolated from the subseafloor sediment (Imachi et al., 2014) and an unknown Chloroflexi species that is associated with the Dehalococcoidia class, a common sub-seafloor bacterium (Wasmund et al., 2014). This finding suggests past conditions of higher salinities for the lake than the current conditions of around 5 g L−1 (Aránguiz-Acuña et al., 2020), close to marine salinity. Archaea representatives found inhabiting the lacustrine sediments include the hydrogenotrophic methanogen Methanolinea (Imachi et al., 2008; Rainey et al., 2015), which is distributed along the length of the core with an abundance ranging from 0.7 %–2.3 % and is very abundant towards the older and more anoxic environment (from 1400 until 1950, Zone III), where methanogenesis is the central predicted metabolism at play. These results suggest that the microbial community in Inka-Coya Lake is potentially heterotrophic with a special enrichment in methanogenic organisms in the oldest deposits, where oxygen levels are lower as evidenced by the metabolic approximation done in this study. Another kind of metabolism, depending on CO2 concentrations, could have dominated during this period.
Overall, our results suggest a great taxonomic and potential metabolic diversity is associated with the microbial community from this lacustrine sediment. There is a vast taxonomic novelty harbored in Inka-Coya Lake sediment; over 70 % of taxa cannot be identified to the genus level, indicating there is a significant amount of “microbial dark matter”, a term associated with unknown microbial representatives that can potentially harbor novel bioactive compounds with numerous applications (Zha et al., 2022; Jiao et al., 2021). This microbial dark matter is also associated with a great metabolic diversity, especially for the production of bioactive compounds with biotechnological potential. For example, we determined that there are abundant representatives of Actinobacteriota, a taxonomic group traditionally associated with the production of antimicrobial, antitumoral, and anti-inflammatory compounds (Al-Shaibani et al., 2021; Taj and Chattopadhyay, 2024); thus we hypothesize there is a high probability of finding novel compounds produced by microorganisms inhabiting the sediment of Inka Coya Lake.
The main drivers for microbial community composition in the Coya sink sediments were As, Sb, V, Mo, Mi, Fe, and Cu, which suggests that there are numerous strategies that microorganisms use to resist high concentrations of metals and metalloids that thrive in this ecosystem (Rahman, 2020; Mathivanan et al., 2021), as observed in the Atacama and Altiplano area (Orellana et al., 2018; Donati et al., 2019; Aszalós et al., 2020; Castro-Severyn et al., 2019). Additionally, microorganisms can use oxido-reduction processes to obtain energy from metal ions (Raab and Feldman, 2003; Staicu and Stolz, 2021). Given the known geochemical characteristics of the area, it is expected that strong relationships are found between the microbial life and inorganic compounds, as they can dissolve and precipitate ores and influence metal and metalloid transformations (Raab and Feldmann, 2003; Zhou and van Hullebusch, 2022). In this extreme environment where competition is strong and abiotic pressures are constant, organic matter and water availability – both parameters critical for most life forms – govern community abundance and composition, suggesting a delicate dynamic balance reached between abiotic and biotic entities at play. It is important to remark that with the number of unclassified taxa, many novel resistant or usage mechanisms remain to be characterized.
Observed trends in magnetic susceptibility in Inka-Coya sediment could be mainly attributed to variations in the concentration of ferromagnetic minerals, such as titanomagnetite, and authigenic origin minerals, such as sulfide (greigite), as is broadly explained in Pérez-Portilla et al. (2024) for this sediment core. In this case, the high χ values would result from a high concentration of ferromagnetic minerals of detrital origin, primarily Fe oxides, while elevated χfd% values would be linked to the presence of greigite of authigenic origin (Pérez-Portilla et al., 2024). Thus, greigite formation typically occurs through the dissolution of titanomagnetite or other detrital minerals containing Fe (e.g., Fialová et al., 2006; Versteeg et al., 1995).
Elevated magnetic susceptibility values in the upper layers of sediments may be attributed to a recent deposition of fine Fe oxide grains, which could be originating and transported from industrial and urban sources; this concurs with the Fe top sediment peak, and it could be related to a diverse superficial community, while in Zone II the lowest values of χ are observed, which could be associated with a mixed community. All these processes occur under a high production of Fe minerals of authigenic origin (χfd% > 3 %; Dearing et al., 1996). Moreover, lower levels of χfd% could be involved in the production and assimilation of iron sulfides such as greigite (Bazylinski, 2001; Lins et al., 2007), promoting a microbial specialization and increasing the resistance of the anaerobic community found in the deeper sediments. Microbial metabolic responses could satisfactorily support several processes associated with greigite formation. The presence of greigite in the lake sediments could be associated with reducing or low-oxygen environments (e.g., Benning et al., 2000), where additionally magnetotactic bacteria could contribute to sedimentary greigite formation through the biomineralization of magnetosomes in anoxic aqueous environments (Moskowitz et al., 2008).
There are statistically significant correlations between some particular taxa and the geochemical composition along the sediment gradient in Inca-Coya Lake suggesting that each element directly influences the metabolic capabilities of the microorganisms and shapes the community-selecting taxa that can resist metal and metalloid toxicity (Yao and Gao, 2007; Laplante et al., 2013; Stankevica et al., 2020; Kostka and Leśniak, 2021; Yan et al., 2020). However, this study is restricted to metabolic approximations based on taxonomy from a single sediment core. Therefore, it would be desirable to carry our future studies including metagenomic data to explore functional potential and investigate other waterbodies to identify temporal patterns of microbiological assemblages in the most exploited area of the Atacama Desert, which will enhance our understanding in terms of the mechanisms used to resist and survive in environments with high metal and metalloid concentrations and the use of different electron acceptors for energy production.
Finally, increasing metal and metalloid exploitation in the region during the last 100 years has directly influenced their mobility and the local geochemistry. In this context, microorganisms from these extreme environments are known to be highly adaptive and have developed several resistance mechanisms and the ability to use these compounds to their benefit. Hence, some bacteria (and their genes) can be used as biomarkers for the bioavailability of such metals and contamination of soils (Li and Wong, 2010; Roosa et al., 2014). In Inka-Coya Lake, a selective process could be evidenced along the sediment record, in which the recent period is characterized by a marked increase in chemical elements and microbial composition, which could be associated with the increasing mining activity and other anthropological activities – as water extraction or aridity increase by climatic changes – that also would increase the disturbance of this relevant area in the core of the most arid non-polar desert.
This study is the first to describe a deep gradient of microbial life in a desert lake in the Atacama area, proposing a biological clustering of taxa and predicted functions in three periods that stratified for over 600 years, including the pre-mining period, the mining development, and the most industrialized mega-mining observed nowadays. A great taxonomic novelty exists among the microbial community inhabiting lacustrine sediments of Inka-Coya Lake, and mineralogical enrichment, water, organic matter availability, and magnetic susceptibility are variables that explain the changes in its abundance and composition. There are strong relationships between geochemical composition and microbial diversity, especially in Cu, Fe, Ni, and V. The first zone is less diverse and dominated by Actinobacteria, and the second zone has a high abundance of Chloroflexi, Acidobacteriota, and Actinobacteriota. The third zone shows more rare taxa with lower abundance and clusters in the more recent sediments closer to the surface, including archaea. Overall, chemoheterotrophy is the prevalent energy production mechanism along the sediment core. This unique and fragile ecosystem depends on biogeochemical dynamics that are vulnerable to anthropogenic activities and climate change.
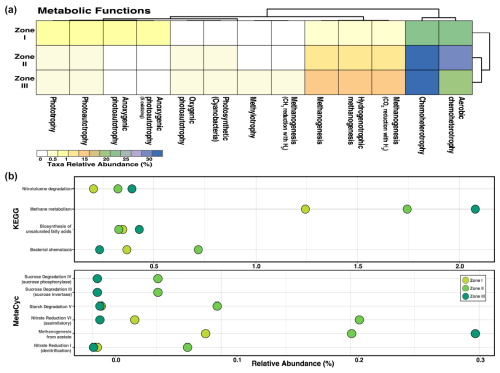
Figure B1Metabolic pathway prediction of the microbial community inhabiting sediments along a depth gradient in Inka-Coya Lake. The predictions are based on the identified taxonomic composition according to the comparison with different databases, such as (a) FAPROTAX, (b) KEGG, and MetaCyc. Categories with significant differences (p<0.05) according to the Kruskal–Wallis test are displayed.
The raw sequencing data presented in this study have been deposited in the DDBJ/ENA/GenBank SRA database (https://www.ncbi.nlm.nih.gov/bioproject/?term=PRJNA1067596, National Library of Medicine, 2024) under the BioProject PRJNA1067596.
Conceptualization: AAA, FR, JCS, CPE. Data curation: CPE, JCS, FR, IHF, AAA. Formal analysis: CPE, JCS, FR, AAA, AM, HP. Funding acquisition: FR, AAA. Methodology: CPE, JCS, AM, HP, FR, AAA. Supervision: AAA, FR. Writing – original draft: CPE, JCS. Writing – review and editing: AAA, AM, HP, JCS, CPE. All authors have read and agreed to the published version of the manuscript.
The contact author has declared that none of the authors has any competing interests.
Publisher’s note: Copernicus Publications remains neutral with regard to jurisdictional claims made in the text, published maps, institutional affiliations, or any other geographical representation in this paper. While Copernicus Publications makes every effort to include appropriate place names, the final responsibility lies with the authors.
We would like the thank the Likan Antay Community of San Francisco de Chiu-Chiu for their collaboration and the MAINI-UCN facilities and team (Kappabridge).
This research was funded by the following grants: ANID 2020 FONDECYT Regular 1200423 (AA-A; FR), UTA-Mayor 9738-24 (AA-A), ANID 2022 FONDECYT Regular 1220902 (FR), ANID 2023 FONDECYT postdoctoral 3230189 (CPE), ANID 2021 FONDECYT postdoctoral 3210156, ANID-Anillo ATE 220007 (JCS), and ANID 2022 MILENIO-NCS2022_009 (AA-A, AM).
OR
This research has been supported by the Fondo Nacional de Desarrollo Científico y Tecnológico (grant nos. 1200423 and 1220902), the Universidad de Tarapacá (grant no. 9738-24), and the Fondo Nacional de Desarrollo Científico y Tecnológico (grant nos. 3230189 and 3210156).
This paper was edited by Tina Treude and reviewed by Gustavo Ramirez and Hongchen Jiang.
Adrian, R., O'Reilly, C. M., Zagarese, H., Baines, S. B., Hessen, D. O., Keller, W., Livingstone, D. M., Sommaruga, R., Straile, D., van Donk, E., Weyhenmeyer, G. A., and Winder, M.: Lakes as sentinels of climate change, Limnol. Oceanogr., 54, 2283–2297, 2009.
Albarracín, V. H., Galván, F. S., and Farías, M. E.: Extreme microbiology at Laguna Socompa: A high-altitude Andean lake (3570 m asl) in Salta, Argentina. Microbial Ecosystems in Central Andes Extreme Environments: Biofilms, Microbial Mats, Microbialites and Endoevaporites, 205–220, https://doi.org/10.1007/978-3-030-36192-1_14, 2020.
Al-Shaibani, M. M., Radin Mohamed, R. M. S., Sidik, N. M., Enshasy, H. A. E., Al-Gheethi, A., Noman, E., and Zin, N. M. Biodiversity of secondary metabolites compounds isolated from phylum actinobacteria and its therapeutic applications, Molecules, 26, 4504, https://doi.org/10.3390/molecules26154504, 2021.
Andersen, K. S., Kirkegaard, R. H., Karst, S. M., and Albertsen, M.: ampvis2: an R package to analyse and visualise 16S rRNA amplicon data, BioRxiv, https://doi.org/10.1101/299537, 2018.
Aránguiz-Acuña, A., Pérez-Portilla, P., De la Fuente, A., and Fontaneto, D.: Life-history strategies in zooplankton promote coexistence of competitors in extreme environments with high metal content, Sci. Rep., 8, 11060, https://doi.org/10.1038/s41598-018-29487-3, 2018.
Aránguiz-Acuña, A., Luque, J. A., Pizarro, H., Cerda, M., Heine-Fuster, I., Valdés, J., and Wennrich, V.: Aquatic community structure as sentinel of recent environmental changes unraveled from lake sedimentary records from the Atacama Desert, Chile, PLoS One, 15, e0229453, https://doi.org/10.1371/journal.pone.0229453, 2020.
Aszalós, J. M., Szabó, A., Felföldi, T., Jurecska, L., Nagy, B., and Borsodi, A. K.: Effects of active volcanism on bacterial communities in the highest-altitude crater lake of Ojos del Salado (Dry Andes, Altiplano-Atacama Region), Astrobiology, 20, 741–753, 2020.
Banda, J. F., Zhang, Q., Ma, L., Pei, L., Du, Z., Hao, C., and Dong, H.: Both pH and salinity shape the microbial communities of the lakes in Badain Jaran Desert, NW China, Sci. Total Environ., 791, 148108, https://doi.org/10.1016/j.scitotenv.2021.148108, 2021.
Bandowe, F. L., Grosjean, M., Tylmann, W., Mosquera, P., Hampel, H., and Schneider, T.: A 150-year record of polycyclic aromatic compound (PAC) deposition from high Andean Cajas National Park, southern Ecuador, STOTEN, 621, 1652–1663, 2018.
Bazylinski, D. A.: Bacterial mineralization, Encyclopedia of materials: Science and technology, 441–447, 2001.
Benito, X.: Benthic Foraminifera and diatoms as ecological indicators. Modern Trends in Diatom Identification: Fundamentals and Applications, 257–280, 2020.
Benning, L. G., Wilkin, R. T., and Barnes, H. L.: Reaction pathways in the Fe–S system below 100 C, Chem Geol., 167, 25–51, 2000.
Borsdorf, A. and Stadel, C.: The Andes: A geographical portrait, Springer, 25–51 pp., https://doi.org/10.1016/j.scitotenv.2021.148108, 2015.
Borsodi, A. K., Aszalós, J. M., Megyes, M., and Nagy, B.: Benthic Bacterial Diversity of High-Altitude Athalassohaline Lakes of the Puna de Atacama (Central Andes), Geomicrobiol. J., 39, 28–38, 2022.
Callahan, B. J., McMurdie, P. J., Rosen, M. J., Han, A. W., Johnson, A., and Holmes, S. P.: DADA2: high-resolution sample inference from Illumina amplicon data, Nat. Methods, 13, 581–583, https://doi.org/10.1038/nmeth.3869, 2016.
Casamayor, E. O., Triadó-Margarit, X., and Castañeda, C.: Microbial biodiversity in saline shallow lakes of the Monegros Desert, Spain, FEMS microbiology ecology, 85, 503–518, 2013.
Caspi, R., Billington, R., Fulcher, C. A., Keseler, I. M., Kothari, A., Krummenacker, M., and Karp, P. D.: The MetaCyc database of metabolic pathways and enzymes, Nuc. acids Res., 46, D633–D639, 2018.
Castro-Severyn, J., Pardo-Esté, C., Sulbaran, Y., Cabezas, C., Gariazzo, V., Briones, A., and Saavedra, C. P.: Arsenic response of three altiplanic Exiguobacterium strains with different tolerance levels against the metalloid species: a proteomics study, Front. Microbiol., 10, 2161, https://doi.org/10.3389/fmicb.2019.02161, eCollection, 2019.
Castro-Severyn, J., Pardo-Esté, C., Mendez, K. N., Fortt, J., Marquez, S., Molina, F., and Saavedra, C. P.: Living to the high extreme: unraveling the composition, structure, and functional insights of bacterial communities thriving in the arsenic-rich Salar de Huasco altiplanic ecosystem, mSpectrum, 9, 10–1128, 2021.
Cavicchioli, R.: Cold-adapted archaea, Nat. Rev. Microbiol., 4, 331–343, 2006.
Cerda, M., Evangelista, H., Valdés, J., Siffedine, A., Boucher, H., Nogueira, J., and Ortlieb, L.: A new 20th century lake sedimentary record from the Atacama Desert/Chile reveals persistent PDO (Pacific Decadal Oscillation) impact, J. South Am. Earth Sci., 95, 102302, https://doi.org/10.1016/j.jsames.2019.102302, 2019.
Cohen, A. S., Manobianco, J., Dettman, D. L., Black, B. A., Beck, C., Feibel, C. S., and Vonhof, H.: Seasonality and lake water temperature inferred from the geochemistry and sclerochronology of quaternary freshwater bivalves from the Turkana Basin, Ethiopia and Kenya, Quaternary Sci. Rev., 317, 108284, https://doi.org/10.1016/j.quascirev.2023.108284, 2023.
Collado, L., Levican, A., Perez, J., and Figueras, M. J.: Arcobacter defluvii sp. nov., isolated from sewage samples, IJSE, 61, 2155–2161, 2011.
Cron, B., Henri, P., Chan, C. S., Macalady, J. L., and Cosmidis, J.: Elemental sulfur formation by Sulfuricurvum kujiense is mediated by extracellular organic compounds, Front. Microbiol., 10, 2710, https://doi.org/10.3389/fmicb.2019.02710, 2019.
Csavina, J., Field, J., Taylor, M. P., Gao, S., Landázuri, A., Betterton, E. A., and Sáez, A. E.: A review on the importance of metals and metalloids in atmospheric dust and aerosol from mining operations, STOTEN, 433, 58–73, 2012.
Da Costa, C., Colin, Y., Debret, M., Copard, Y., Gardes, T., Jacq, K., and Berthe, T. Shifts in sediment bacterial communities reflect changes in depositional environments in a fluviatile context, STOTEN, 885, 163890, https://doi.org/10.1016/j.scitotenv.2023.163890, 2023.
Dearing, J. A., Hay, K. S., Baban, A. S., Huddleston, E. M., Wellington H., and Loveland P. J.: Magnetic susceptibility of topsoils: a test of conflicting theories using a national database, Geophys. J. Int., 127, 728–734, 1996.
Demergasso, C., Escudero, L., Casamayor, E. O., Chong, G., Balagué, V., and Pedrós-Alió, C.: Novelty and spatio–temporal heterogeneity in the bacterial diversity of hypersaline Lake Tebenquiche (Salar de Atacama), Extremophiles, 12, 491–504, 2008.
Deng, Y., Mou, T., Wang, J., Su, J., Yan, Y., and Zhang, Y. Q.: Characterization of three rapidly growing novel Mycobacterium species with significant polycyclic aromatic hydrocarbon bioremediation potential, Front. Microbiol., 14, 1–15 pp., https://doi.org/10.3389/fmicb.2023.1225746, 2023.
Dittmar, T.: Hydrochemical process controlling arsenic and heavy metal contamination in the Elqui river system (Chile), STOTEN, 325, 193–207, 2004.
Donati, E. R., Sani, R. K., Goh, K. M., and Chan, K. G.: Recent advances in bioremediation/biodegradation by extreme microorganisms, Front. Microbiol., 10, 1851, https://doi.org/10.3389/fmicb.2019.01851, 2019.
Dong, H., Huang, L., Zhao, L., Zeng, Q., Liu, X., Sheng, Y., and Chen, H.: A critical review of mineral–microbe interaction and co-evolution: mechanisms and applications, Nat. Sci. Rev., 9, nwac128, https://doi.org/10.1093/nsr/nwac128, 2022.
Dorador, C., Fink, P., Hengst, M., Icaza, G., Villalobos, A. S., Vejar, D., and Harrod, C.: Microbial community composition and trophic role along a marked salinity gradient in Laguna Puilar, Salar de Atacama, Chile, Antonie Van Leeuwenhoek, 111, 1361–1374, 2018.
Douglas, G. M., Maffei, V. J., Zaneveld, J. R., Yurgel, S. N., Brown, J. R., Taylor, C. M., and Langille, M. G.: PICRUSt2 for prediction of metagenome functions, Nat. Biotechnol., 38, 685–688, 2020.
Falkinham III, J. O.: The biology of environmental mycobacteria, Env. Microbiol. Rep., 1, 477–487, 2009.
Farias, M. E., Contreras, M., Rasuk, M. C., Kurth, D., Flores, M. R., Poire, D. G., and Visscher, P. T.: Characterization of bacterial diversity associated with microbial mats, gypsum evaporites and carbonate microbialites in thalassic wetlands: Tebenquiche and La Brava, Salar de Atacama, Chile, Extremophiles 18, 311–329, 2014.
Fernandez, A. B., Rasuk, M. C., Visscher, P. T., Contreras, M., Novoa, F., Poire, D. G., and Farias, M. E.: Microbial diversity in sediment ecosystems (evaporites domes, microbial mats, and crusts) of hypersaline Laguna Tebenquiche, Salar de Atacama, Chile, Front. Microbiol., 7, 1284, https://doi.org/10.3389/fmicb.2016.01284, 2016.
Fialová, H., Maier, G., Petrovský, E., Kapička, A., Boyko, T., Scholger, R., and MAGPROX Team.: Magnetic properties of soils from sites with different geological and environmental settings, J. Appl. Geophys., 59, 273–283, 2006.
Garreaud, R., Vuille, M., and Clement, A. C.: The climate of the Altiplano: observed current conditions and mechanisms of past changes, Palaeogeogr., 194, 5–22, 2003.
Grosjean, M. and Veit, H. Water resources in the arid mountains of the Atacama Desert (northern Chile): past climate changes and modern conflicts, Global change and mountain regions: an overview of current knowledge, Springer, Dordrecht, 23, 93–104, https://doi.org/10.1007/1-4020-3508-X_10, 2005.
Hamilton, P. B., Rolshausen, G., Uren Webster, T. M., and Tyler, C. R.: Adaptive capabilities and fitness consequences associated with pollution exposure in fish, Proc. R. Soc. B, 372, 20160042, https://doi.org/10.1098/rstb.2016.0042, 2017.
Han, Y. and Perner, M.: The globally widespread genus Sulfurimonas: versatile energy metabolisms and adaptations to redox clines, Front Microbiol., 6, 989, https://doi.org/10.3389/fmicb.2015.00989, 2015.
Haslett, J. and Parnell, A.: A simple monotone process with application to radiocarbon-dated depth chronologies, J. R. Stats., 57, 399–418, 2008.
Heiri, O., Lotter, A. F., and Lemcke, G.: Loss on ignition as a method for estimating organic and carbonate content in sediments: reproducibility and comparability of results, J. Paleolimnol., 25, 101–110, 2001.
Herlemann, D. P., Labrenz, M., Jürgens, K., Bertilsson, S., Waniek, J. J., and Andersson, A. F.: Transitions in bacterial communities along the 2000 km salinity gradient of the Baltic Sea, The ISME journal, 5, 1571–1579, 2011.
Hogg, A. G., Heaton, T. J., Hua, Q., Palmer, J. G., Turney, C. S. M., Southon, J., Bayliss, A., Blackwell, P. G., Boswijk, G., Bronk Ramsey, C., Pearson, C., Petchey, F., Reimer, P., Reimer, R. and Wacker, L.: SHCal20 southern hemisphere calibration, 0–55,000 years cal bp, Radiocarbon. Cambridge University Press, 1–20 pp., http://intcal.org/curves/shcal20.14c (last acces: October 2024), 2020.
Imachi, H., Sakai, S., Sekiguchi, Y., Hanada, S., Kamagata, Y., Ohashi, A., and Harada, H.: Methanolinea tarda gen. nov., sp. nov., a methane-producing archaeon isolated from a methanogenic digester sludge, IJSEM, 58, 294–301, 2008.
Imachi, H., Sakai, S., Lipp, J. S., Miyazaki, M., Saito, Y., Yamanaka, Y., and Takai, K.: Pelolinea submarina gen. nov., sp. nov., an anaerobic, filamentous bacterium of the phylum Chloroflexi isolated from subseafloor sediment, Int. J. Syst. Evol. Micr., 64, 812–818, 2014.
Jiao, J. Y., Liu, L., Hua, Z. S., Fang, B. Z., Zhou, E. M., Salam, N., and Li, W. J.: Microbial dark matter coming to light: challenges and opportunities, Nat. Sci. Rev., 8, nwaa280, https://doi.org/10.1093/nsr/nwaa280, 2021.
Kadnikov, V. V., Mardanov, A. V., Beletsky, A. V., Karnachuk, O. V., and Ravin, N. V.: Genome of the candidate phylum Aminicenantes bacterium from a deep subsurface thermal aquifer revealed its fermentative saccharolytic lifestyle, Extremophiles, 23, 189–200, 2019.
Kanehisa, M., Goto, S., Sato, Y., Furumichi, M., and Tanabe, M.: KEGG for integration and interpretation of large-scale molecular data sets, Nucleic acids Res., 40, D109–D114, 2012.
Kassambara, A.: R Graphics Essentials for Great Data Visualization: 200 Practical Examples You Want to Know for Data Science, STHDA, 1–142 pp., https://www.sthda.com/french/ (last access: April 2024), 2017.
Keller, W., Yan, N. D., Holtze, K., and Pitblado, J. R.: Chemical response of acid lakes in the Sudbury, Ontario area to reduced smelter emissions, 1981–1989, Can. J. Fish Aquat. Sci., 49, 25–32, 1992.
Kereszturi, Á., Aszalós, J. M., Heiling, Z., Ignéczi, Á., Kapui, Z., Király, C., and Szalai, Z.: Cold, dry, windy, and UV irradiated: surveying Mars-relevant conditions in Ojos del Salado Volcano (Andes Mountains, Chile), Astrobiology, 20, 677–683, 2020.
Korosi, J. B., Thienpont, J. R., Smol, J. P., and Blais, J. M.: Paleo-ecotoxicology: what can lake sediments tell us about ecosystem responses to environmental pollutants?, Environ. Sci. Technol., 51, 9446–9457, 2017.
Kostka, A. and Leśniak, A.: Natural and anthropogenic origin of metals in lacustrine sediments; assessment and consequences – A case study of Wigry lake (Poland), Minerals, 11, 158, https://doi.org/10.3390/min11020158, 2021.
Kurth, D., Elias, D., Rasuk, M. C., Contreras, M., and Farias, M. E.: Carbon fixation and rhodopsin systems in microbial mats from hypersaline lakes Brava and Tebenquiche, Salar de Atacama, Chile, PLoS One, 16, e0246656, https://doi.org/10.1093/nsr/nwaa280, 2021.
Lahti, L., Shetty, S., Blake, T., and Salojarvi, J.: Tools for microbiome analysis in R Version 2.1. 26, Bioc Package, https://github.com/microbiome/microbiome (last acces: October 2024), 2017.
Laplante, K., Sébastien, B., and Derome, N.: Parallel changes of taxonomic interaction networks in lacustrine bacterial communities induced by a polymetallic perturbation, Evol. Appl., 6, 643–659, 2013.
Li, W. C. and Wong, M. H.: Effects of bacteria on metal bioavailability, speciation, and mobility in different metal mine soils: a column study, J. Soils Sediments, 10, 313–325, 2010.
Lins, U., Keim, C. N., Evans, F. F., Farina, M., and Buseck, P. R.: Magnetite (Fe3O4) and greigite (Fe3S4) crystals in multicellular magnetotactic prokaryotes, Geomicrobiology J., 24, 43–50, 2007.
Louca, S., Parfrey, L. W., and Doebeli, M.: Decoupling function and taxonomy in the global ocean microbiome, Science, 353, 1272–1277, 2016.
Love, M. I., Huber, W., and Anders, S.: Moderated estimation of fold change and dispersion for RNA-seq data with DESeq2, Genome Biol., 15, 1–21, 2014.
Madsen, E. L.: Microorganisms and their roles in fundamental biogeochemical cycles, Curr. Opinion Biotech., 22, 456–464, 2011.
Mathivanan, K., Chandirika, J. U., Vinothkanna, A., Yin, H., Liu, X., and Meng, D.: Bacterial adaptive strategies to cope with metal toxicity in the contaminated environment – A review, Ecotox Env. Safety, 226, 112863, https://doi.org/10.1016/j.ecoenv.2021.112863, 2021.
McMurdie, P. and Holmes, S.: phyloseq: an R package for reproducible interactive analysis and graphics of microbiome census data, PLoS ONE, 8, e61217, https://doi.org/10.1371/journal.pone.0061217, 2013.
Moskowitz, B. M., Bazylinski, D. A., Egli, R., Frankel, R. B., and Edwards, K. J.: Magnetic properties of marine magnetotactic bacteria in a seasonally stratified coastal pond (Salt Pond, MA, USA), GJI, 174, 75–92, 2008.
National Library of Medicine – National Center for Biotechnology Information: Raw sequencing data (BioProject: PRJNA1067596), https://www.ncbi.nlm.nih.gov/bioproject/?term=PRJNA1067596 (last access: January 2024), 2024.
Niu, Z. S., Yang, Y., Tou, F. Y., Guo, X. P., Huang, R., Xu, J., and Hochella, M. F. Sulfate-reducing bacteria (SRB) can enhance the uptake of silver-containing nanoparticles by a wetland plant, Env. Sci. Nano, 7, 912–925, 2020.
Olilo, C. O., Odoli, C. O., Obiero, M. O., Malala, J. O., and Bironga, C. H. Microbial ecology of desert Lakes Baringo and Turkana, Kenya, East Africa, in: Lakes of Africa, 247–268 pp., Elsevier, https://doi.org/10.1016/B978-0-323-95527-0.00016-6, 2023.
Ordoñez, O. F., Flores, M. R., Dib, J. R., Paz, A., and Farías, M. E.: Extremophile culture collection from Andean lakes: extreme pristine environments that host a wide diversity of microorganisms with tolerance to UV radiation, Microb. Ecol., 58, 461–473, 2009.
Orellana, R., Macaya, C., Bravo, G., Dorochesi, F., Cumsille, A., Valencia, R., and Seeger, M.: Living at the frontiers of life: extremophiles in Chile and their potential for bioremediation, Front. Microbiol., 9, 2309, https://doi.org/10.3389/fmicb.2018.02309, 2018.
Oren, A. and Garrity, G. M.: Valid publication of the names of forty-two phyla of prokaryotes, Int. J. Syst. Evolut. Microbiol., 71, 005056, https://doi.org/10.1099/ijsem.0.005056, 2021.
Pérez-Cataluña, A., Salas-Massó, N., Diéguez, A. L., Balboa, S., Lema, A., Romalde, J. L., and Figueras, M. J. Revisiting the taxonomy of the genus Arcobacter: getting order from the chaos, Front. Microbiol., 9, 2077, https://doi.org/10.3389/fmicb.2018.02077, 2018.
Pérez-Portilla, P., Aránguiz-Acuña, A., Pizarro, H., and Herrera, J.: Assessing the Effects of Long-Term Mining Exploitation on a Lacustrine System from the Arid Region of the Atacama Desert, Chile, STOTEN, 949, https://doi.org/10.1016/j.scitotenv.2024.174771, 2024.
Picard, M., Wood, S. A., Pochon, X., Vandergoes, M. J., Reyes, L., Howarth, J. D., and Puddick, J.: Molecular and pigment analyses provide comparative results when reconstructing historic cyanobacterial abundances from lake sediment cores, Microorganisms, 10, 279, https://doi.org/10.3390/microorganisms10020279, 2022.
Pigati, J. S., Miller, D. M., Bright, J., Mahan, S. A., Nekola, J. C., and Paces, J. B.: Chronology, sedimentology, and micro-fauna of ground-water discharge deposits in the central Mojave Desert, Valley Wells, California, Geol Soc Am Bull., 123, 2224–2239, 2011.
Placzek, C., Quade, J., Betancourt, J. L., Patchett, P. J., Rech, J. A., Latorre, C., and English, N. B.: Climate in the dry central Andes over Geologic, millennial, and interannual timescales, Ann. Mo. Bot. Gard., 96, 386–397, 2009.
Pollard, H. G., Colbourne, J. K., and Keller, W.: Reconstruction of Centuries-old Daphnia Communities in a Lake Recovering from Acidification and Metal Contamination, Ambio., 32, 214–218, 2003.
Price, M. N., Dehal, P. S., and Arkin, A. P.: FastTree: computing large minimum evolution trees with profiles instead of a distance matrix, Mol. Biol. Evol., 26, 1641–1650, 2009.
Pueyo, J. J., Sáez, A., Giralt, S., Valero-Garcés, B. L., Moreno, A., Bao, R., Schwalb, A., Herrera, C., Klosowska, B., and Taberner, C.: Carbonate and organic matter sedimentation and isotopic signatures in Lake Chungará, Chilean Altiplano, during the last 12.3 kyr, Palaeogeogr. Palaeocl., 307, 339–355, 2011.
Quast, C., Pruesse, E., Yilmaz, P., Gerken, J., Schweer, T., Yarza, P., Peplies, J., and Glockner, F.: The SILVA ribosomal RNA gene database project: improved data processing and web-based tools, Nucl. Acids Res., 41, D590–D596, 2012.
Raab, A. and Feldmann, J.: Microbial transformation of metals and metalloids, Sci. Prog., 86, 179–202, 2003.
Rahman, Z.: An overview on heavy metal resistant microorganisms for simultaneous treatment of multiple chemical pollutants at co-contaminated sites, and their multipurpose application, J. Haz. Mat., 396, 122682, https://doi.org/10.1016/j.jhazmat.2020.122682, 2020.
Rainey, F., Kämpfer, P., Trujillo, M., Chun, J., DeVos, P., Hedlund, B., and Dedysh, S. Bergey's manual of systematics of Archaea and Bacteria, Vol. 410, edited by: Whitman, W. B., Hoboken, NJ: Wiley, https://doi.org/10.1002/9781118960608.gbm00186, 2015.
Ramírez, M., Massolo, S., Frache, R., and Correa, J. A.: Metal speciation and environmental impact on sandy beaches due to El Salvador copper mine, Chile. Mar Pollut Bull., 50, 62–72, 2005.
Ramos-Tapia, I., Salinas, P., Núñez, R., Cortez, D., Soto, J., and Paneque, M.: Compositional Changes in Sediment Microbiota Are Associated with Seasonal Variation of the Water Column in High-Altitude Hyperarid Andean Lake Systems, Microbiol Spectr., 15, e0520022, https://doi.org/10.1038/s41598-019-41743-8, 2023.
Rasuk, M. C., Kurth, D., Flores, M. R., Contreras, M., Novoa, F., Poire, D., and Farias, M. E.: Microbial characterization of microbial ecosystems associated to evaporites domes of gypsum in Salar de Llamara in Atacama Desert, Microb. Ecol. 71, 44–56, 2014.
Ritter, B., Wennrich, V., Medialdea, A., Brill, D., King, G., Schneiderwind, S., and Dunai, T. J.: Climatic fluctuations in the hyperarid core of the Atacama Desert during the past 215 ka, Sci. Rep., 9, 5270, https://doi.org/10.3390/land11122278, 2019.
Rodríguez-Luna, D., Encina-Montoya, F., Alcalá, F. J., and Vela, N.: An Overview of the Environmental Impact Assessment of Mining Projects in Chile, Land, 11, 2278, https://doi.org/10.1089/env.2011.0017, 2022.
Romero, L., Alonso, H., Campano, P., Fanfani, L., Cidu, R., Dadea, C., and Farago, M.: Arsenic enrichment in waters and sediments of the Rio Loa (Second Region, Chile), App. Geochem., 18, 1399–1416, 2003.
Roosa, S., Wattiez, R., Prygiel, E., Lesven, L., Billon, G., and Gillan, D. C.: Bacterial metal resistance genes and metal bioavailability in contaminated sediments, Env. Pollut., 189, 143–151, 2014.
Salvarredy-Aranguren, M. M., Probst, A., Roulet, M., and Isaure M.-P.: Contamination of surface waters by mining wastes in the Milluni Valley (Cordillera Real, Bolivia): Mineralogical and hydrological influences, Appl. Geochem., 23, 1299–1324, 2008.
Santini, T. C., Gramenz, L., Southam, G., and Zammit, C.: Microbial community structure is most strongly associated with geographical distance and PH in salt lake sediments, Front. Microbiol., 13, 920056, https://doi.org/10.1093/femsec/fiab056, 2022.
Staicu, L. C. and Stolz, J. F.: Microbes vs. metals: Harvest and recycle, FEMS Microbiol. Ecol., 97, fiab056, https://doi.org/10.5200/baltica.201, 2021.
Stankevica, K., Vincevica-Gaile, Z., Klavins, M., Kalnina, L., Stivrins, N., Grudzinska, I., and Kaup, E.: Accumulation of metals and changes in composition of freshwater lake organic sediments during the Holocene, Chem. Geol., 539, 119502, https://doi.org/10.1371/journal.pone.0089531, 2020.
Stoeva, M. K., Aris-Brosou, S., Chételat, J., Hintelmann, H., Pelletier, P., and Poulain, A. J.: Microbial community structure in lake and wetland sediments from a high Arctic polar desert revealed by targeted transcriptomics, PLoS One, 9, e89531, https://doi.org/10.1007/s40203-024-00209-0, 2014.
Taj, Z. and Chattopadhyay, I.: Identification of bio-active secondary metabolites from Actinobacteria as potential drug targets against Porphyromonas gingivalis in oral squamous cell carcinoma using molecular docking and dynamics study, in: Silico Pharmacology, 12, 1, https://doi.org/10.1016/j.envpol.2022.120151, 2024.
Tapia, J., Davenport, J., Townley, B., Dorador, C., Schneider, B., Tolorza, V., and von Tümpling, W.: Sources, enrichment, and redistribution of As, Cd, Cu, Li, Mo, and Sb in the Northern Atacama Region, Chile: implications for arid watersheds affected by mining, J. Geochem. Exploration, 185, 33–51, 2018.
Tapia, J., Murray, J., Ormachea, M., Tirado, N., and Nordstrom, D. K.: Origin, distribution, and geochemistry of arsenic in the Altiplano-Puna plateau of Argentina, Bolivia, Chile, and Perú, STOTEN, 678, 309–325, 2019.
Tapia, J., Mukherjee, A., Rodríguez, M. P., Murray, J., and Bhattacharya, P.: Role of tectonics and climate on elevated arsenic in fluvial systems: Insights from surface water and sediments along regional transects of Chile, Env. Pollut., 314, 120151, https://doi.org/10.1016/j.envpol.2022.120151, 2022.
Teunisse, G. M.: Fantaxtic – Nested Bar Plots for Phyloseq Data (Version 2.0.1) [Computer software], https://github.com/gmteunisse/Fantaxtic (last access: September 2024), 2022.
Thukral, A. K.: A review on measurement of Alpha diversity in biology, Agri. Res. J., 54, 1–10 pp., https://doi.org/10.5958/2395-146X.2017.00001.1, 2017.
Trolle, D., Hamilton, D. P., and Pilditch, C. A.: Evaluating the influence of lake morphology, trophic status and diagenesis on geochemical profiles in lake sediments, Appl. Geochem., 25, 621–632, 2010.
Usenko, S., Landers, D. H., Appleby, P. G., and Simonich, S. L.: Current and historical deposition of PBDEs, pesticides, PCBs, and PAHs to Rocky Mountain National Park, Environ. Sci. Technol., 41, 7235–7241, 2007.
Valero-Garcés, B. L., Delgado-Huertas, A., Navas, A., Edwards, L., Schwalb, A., and Ratto, N.: Patterns of regional hydrological variability in central-southern Altiplano (18–26?S) lakes during the last 500 years, Palaeogeogr. Palaeocl., 194, 319–338, 2003.
Vargas-Machuca, B. D., Zanetta-Colombo, N., De Pol-Holz, R., and Latorre, C.: Variations in local heavy metal concentrations over the last 16,000 years in the central Atacama Desert (22° S) measured in rodent middens, STOTEN, 775, 145849, https://doi.org/10.1016/j.scitotenv.2021.145849, 2021.
Verosub, K. L. and Roberts, A. P.: Environmental magnetism: Past, present, and future, J. Geophys., 100, 2175–2192, 1995.
Versteeg, J. K., Morris, W. A., and Rukavina, N. A.: The utility of magnetic properties as a proxy for mapping contamination in Hamilton Harbour sediment, J. Great Lakes Res., 21, 71–83, 1995.
Vignale, F. A., Lencina, A. I., Stepanenko, T. M., Soria, M. N., Saona, L. A., Kurth, D., and Farías, M. E.: Lithifying and non-lithifying microbial ecosystems in the wetlands and salt flats of the Central Andes, Microb Ecol., 775, 1–17, 2021.
Wasmund, K., Schreiber, L., Lloyd, K. G., Petersen, D. G., Schramm, A., Stepanauskas, R., and Adrian, L.: Genome sequencing of a single cell of the widely distributed marine subsurface Dehalococcoidia, phylum Chloroflexi, ISME J., 8, 383–397, https://doi.org/10.1038/ismej.2013.143, 2014.
Wickham, H.: ggplot2: Elegant Graphics for Data Analysis, Springer-Verlag New York, ISBN 978-3-319-24277-4, https://ggplot2.tidyverse.org (last access: September 2024), 2016.
Yan, C., Wang, F., Liu, H., Liu, H., Pu, S., Lin, F., and Yuan, R.: Deciphering the toxic effects of metals in gold mining area: microbial community tolerance mechanism and change of antibiotic resistance genes, Env. Res., 189, 109869, https://doi.org/10.1016/j.envres.2020.109869, 2020.
Yan, Y., He, A., Dilek, Y., Zhu, Z., and Zhao, Q.: Landscape inversion episodes in SE China during the Mesozoic–early Cenozoic: Constrained by trace-element contents, Nd isotope geochemistry, and detrital zircon U-Pb geochronology of sedimentary basins, GSA Bulletin., 136, 2978–2998, 2024.
Yang, C., Mai, J., Cao, X., Burberry, A., Cominelli, F., and Zhang, L.: ggpicrust2: an R package for PICRUSt2 predicted functional profile analysis and visualization, Bioinformatics, 39, btad470, https://doi.org/10.1093/bioinformatics/btad470, 2023.
Yao, Z. and Gao, P.: Heavy metal research in lacustrine sediment: a review, Chin. J. Ocean. Limnol., 25, 444–454, 2007.
Zanetta-Colombo, N. C., Fleming, Z. L., Gayo, E. M., Manzano, C. A., Panagi, M., Valdés, J., and Siegmund, A.: Impact of mining on the metal content of dust in indigenous villages of northern Chile, Env. Intl., 169, 107490, https://doi.org/10.1016/j.envint.2022.107490, 2022.
Zanetta-Colombo, N. C., Scharnweber, T., Christie, D. A., Manzano, C. A., Blersch, M., Gayo, E. M., and Nüsser, M.: When another one bites the dust: Environmental impact of global copper demand on local communities in the Atacama mining hotspot as registered by tree rings, STOTEN, 920, 170954, https://doi.org/10.1016/j.scitotenv.2024.170954, 2024.
Zha, Y., Chong, H., Yang, P., and Ning, K.: Microbial dark matter: from discovery to applications, GPB, 20, 867–881, 2022.