the Creative Commons Attribution 4.0 License.
the Creative Commons Attribution 4.0 License.
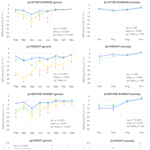
Seasonal carbon fluxes from vegetation and soil in a Mediterranean non-tidal salt marsh
Lorena Carrasco-Barea
Dolors Verdaguer
Maria Gispert
Xavier D. Quintana
Hélène Bourhis
Laura Llorens
Salt marshes are important ecosystems for carbon sequestration. However, while studies of atmospheric carbon exchange fluxes have broadly been performed in tidal salt marshes, they are scarce in non-tidal salt marshes. In this study we measured, throughout 1 year, instantaneous net carbon dioxide (CO2) exchange rates from four halophytes which are dominant species of their corresponding habitat (Sarcocornia fruticosa in a halophilous scrub, Halimione portulacoides and Elytrigia atherica in a salt meadow, and Salicornia patula in a glasswort sward) of a Mediterranean non-tidal salt marsh. Soil CO2 and methane (CH4) fluxes from these habitats were also measured. E. atherica, a perennial herbaceous species, showed the highest photosynthetic rates during the entire year, but S. patula, an annual succulent herb, also had remarkable photosynthetic rates in summer. Interestingly, the woody fraction of the two perennial shrubs, S. fruticosa and H. portulacoides, showed CO2 uptake during most of the daily measurements. Regarding the studied habitats, the halophilous scrub and the salt meadow showed higher soil CO2 emissions than the glasswort sward, and the overall emissions were higher than those reported for tidal salt marshes. Both soil absorption and emission of CH4 were detected. In particular, CH4 emissions were remarkably high, similar to those found in low-salinity marshes and, in general, higher than those reported for salt marshes with a high water table salinity. Soil mineralization quotients of the halophilous scrub and the salt meadow were lower than those measured at the glasswort sward, suggesting a higher soil carbon sequestration potential of the first two habitats.
- Article
(2142 KB) - Full-text XML
-
Supplement
(803 KB) - BibTeX
- EndNote
In the last decades there has been a continuous increase in atmospheric carbon dioxide (CO2) and methane (CH4) concentrations, two significantly active greenhouse gases, which has contributed to global climate change. In 2022, the global averages of atmospheric CO2 and CH4 concentrations were 417.9 ± 0.2 ppm and 1913.9 ± 0.4 ppb, respectively (Lan et al., 2023b, a), which represents an increase of 150 % and 264 % compared to the atmospheric concentration of these gases in 1750 (Blunden and Arndt, 2019). In this context of continuous global warming, ecosystems play an important role in global climate regulation. Therefore, it is essential to determine net emissions of greenhouse gases of ecosystems to estimate their effects on global warming (Ciais et al., 2013).
In particular, salt marshes play an important role in global climate regulation, since they are considered one of the most powerful carbon sinks on Earth (Laffoley and Grimsditch, 2009) due to their high net primary productivity and low rates of organic matter decomposition (McLeod et al., 2011; Mitsch and Gosselink, 2015). Previous studies on the photosynthetic capacity of salt marsh halophytic species have mainly focused on the effect of salinity on photosynthetic rates, and most of these studies were performed under controlled conditions (Davy et al., 2006; Duarte et al., 2014; Kuramoto and Brest, 1979; Nieva et al., 1999; Pearcy and Ustin, 1984; Redondo-Gómez et al., 2007) and less frequently under field conditions (Drake, 1989; Maricle and Maricle, 2018; Warren and Brockelman, 1989). Among the latter, the few studies that have characterized temporal patterns of CO2 exchange from salt marsh plant species have been carried out in tidal salt marshes (Antlfinger and Dunn, 1979; Das Neves et al., 2008; Nieva et al., 2003).
In salt marshes, species with different photosynthetic carbon metabolism (such as C3 and C4 species) and/or belonging to different plant classes (such as monocotyledonous and dicotyledonous species) share the same habitats contributing differently to the photosynthetic removal of atmospheric CO2 (Nieva et al., 1999; Pearcy and Ustin, 1984). Photosynthetic rates also depend on abiotic factors, such as light, temperature, flooding regime, salinity, or nutrient availability (Drake, 1989; Huckle et al., 2000). In general it is assumed that the highest plant photosynthetic activity occurs during the hours of the day with the highest solar radiation (midday) (Antlfinger and Dunn, 1979; Nieva et al., 2003). However, in salt marshes subjected to Mediterranean climate, high temperature and low soil moisture can become noticeable limiting factors for photosynthesis at midday, especially during summer (Das Neves et al., 2008). Other factors, such as tidal regime or soil salinity, can also reduce CO2 uptake and influence the seasonal photosynthetic patterns of salt marsh species (Das Neves et al., 2008; Nieva et al., 2003).
In addition to vegetation, soil carbon fluxes also influence the role that salt marshes play in climate regulation (Bridgham et al., 2006; Chmura et al., 2003). Soil carbon (CO2 and CH4) emissions are related with the organic matter decomposition performed by microorganisms (Chmura, 2011), being usually positively correlated with soil organic carbon content (Li et al., 2019; Wang et al., 2016). In salt marshes, flooding also has a major effect on CO2 and CH4 emissions, since it determines which process, aerobic respiration or anaerobic metabolism, prevails. Under aerobic conditions, organic matter can be oxidized completely to CO2, whereas, during flooding periods, when soil reaches anoxic conditions, aerobic respiration is replaced by fermentation and methanogenesis (Mitsch and Gosselink, 2015). In this sense, ecosystems that usually or periodically have submerged soils, such as salt marshes, are among the major sources of CH4 (Dalal and Allen, 2008). Nevertheless, soil CH4 emissions are generally negatively affected by salinity (Bartlett and Harriss, 1993; Livesley and Andrusiak, 2012; Poffenbarger et al., 2011), since, in saline environments, the presence of the ion sulfate allows sulfate-reducing bacteria to compete with methanogens for energy sources and consequently disfavor and even inhibit methane production (Bartlett et al., 1987; DeLaune et al., 1983). Specifically, aceticlastic and hydrogenotrophic methanogens, with their lower energetic yields, are more susceptible to increasing salinity than methylotrophic methanogens, which explains the predominance of methylotrophic methanogens like Methanohalophilus spp. in hypersaline environments (McGenity and Sorokin, 2018). Temperature also affects soil carbon emissions in salt marshes, with the highest CO2 and CH4 emissions being mostly recorded in the warmest season, since high temperatures enhance the metabolic activity of soil microorganisms (Chen et al., 2018; Hu et al., 2017; Wang et al., 2016). Despite the importance of soil carbon fluxes in climate regulation, few studies have characterized these fluxes in Mediterranean salt marshes (Wang, 2018), and, to our knowledge, not one study has been performed in non-tidal salt marshes (tides range from 0.1 to 1 m, in contrast to 1–10 m of tidal salt marshes) of the Mediterranean Basin. Hence, considering the extensive coverage of non-tidal salt marshes in the Mediterranean Basin, which has been estimated to be approximately 19 million ha (around 2.5 % of the total area of the 27 Mediterranean countries and 1 % to 2 % of wetlands in the world; Geijzendorffer et al., 2018), and recognizing the potential variations in carbon flux patterns due to distinct flooding regimes, temperature fluctuations, and annual rainfall distribution (Ibañez et al., 2000), it is essential to study CO2 and CH4 fluxes in these salt marsh ecosystems.
The aim of this study was to assess the CO2 fluxes from vegetation, along with CO2 and CH4 fluxes from the soil, in the three main habitats of a Mediterranean non-tidal salt marsh. To achieve this objective, we measured seasonally, throughout 1 year, instantaneous net CO2 exchange rates from the dominant plant species of these three habitats at four daily periods (after sunrise, at midday, before sunset, and at night) in green and woody (if present) tissues. Additionally, daily CO2 and CH4 fluxes from soils (or water, in the case of flooded soils) were also recorded.
2.1 Study zone
The study was performed at La Pletera, a coastal Mediterranean non-tidal salt marsh located in the north of the mouth of the Ter river in the municipality of Torroella de Montgrí (Girona, NE of the Iberian Peninsula; N E; Fig. S1 in the Supplement). The largest area of this salt marsh is dominated by three Habitats of Community Interest (HCI), which, in accordance with the European Union's Habitats Directive (Council Directive 92/43/EEC; see Annex 1 of the Directive, European Commission, 1997), are habitats with a high ecological value that are at risk of disappearance. These habitats are the Mediterranean halophilous scrub (HCI code 1420), dominated by Sarcocornia fruticosa (syn. Arthrocnemum fruticosum); the Mediterranean salt meadow (HCI code 1410), dominated by Elytrigia atherica (syn. Elymus pycnanthus) and Halimione portulacoides (syn. Atriplex portulacoides); and the glasswort sward (HCI code 1310), dominated by Salicornia patula, all these species being C3 plants. S. fruticosa and H. portulacoides are perennial halophytic species from the Chenopodiaceae family. S. fruticosa is a shrub with highly reduced leaves and succulent photosynthetic green and woody stems, while H. portulacoides is a creeping subshrub with slightly fleshy leaves and woody stems. S. patula also belongs to the Chenopodiaceae family, being an annual succulent herb with highly reduced leaves and succulent articulated green stems. Finally, E. atherica is a perennial, herbaceous, monocotyledonous species belonging to the Poaceae family.
La Pletera salt marsh has a coastal Mediterranean climate with the lowest mean temperatures occurring in winter and the highest in summer (around 9 and 24 °C for the previous 10 years, respectively; Fig. S2) and with autumn and spring being the seasons with the highest monthly accumulated rainfall (Fig. S2). Astronomical tides are almost imperceptible (0.2–0.3 m). Meteorological events, such as strong easterly winds and rainfall, are the main causes of flooding, mostly occurring in autumn. The duration of flooding varies among habitats, with the shortest duration in the salt meadow (a few days at most), an intermediate duration in the halophilous scrub (several weeks), and the longest duration in the glasswort sward (ranging from several weeks to several months) (Pascual and Martinoy, 2017). The salinity of the water table is around 0.86 ‰. During summer, sea water intrusion typically happens, which moves the saltwater wedge inland and increases groundwater salinity until it reaches levels similar to those of the sea (approximately 32 ‰) (Menció et al., 2017).
2.2 Seasonal CO2 fluxes from vegetation
Throughout 1 year (2017), instantaneous net CO2 exchange rates (NERs) and stomatal conductance (gs) were seasonally monitored for S. fruticosa, H. portulacoides, E. atherica, and S. patula. Measurements were performed in the field, using attached living green and woody plant tissues (except for E. atherica and S. patula, which had only green tissues), by means of a PLC3 conifer leaf cuvette (80×40 mm) connected to an infrared gas analyzer (IRGA; CIRAS-2, PP Systems USA). Only woody stems of maximum 3 mm in diameter were used, which represented 35 % and 100 % of the total woody live biomass of S. fruticosa and H. portulacoides, respectively (Carrasco-Barea et al., unpublished data), since thicker stems did not allow the PLC3 leaf cuvette to close. Daylight measurements were always carried out on sunny days in sun-exposed vegetal tissues after sunrise, at midday, and before sunset. They were performed every 1.5 months for green tissues and every 3 months for woody tissues. Night measurements were taken 1 h after the complete absence of light once per season. The frequency of samplings per season and the number of plants measured per species in each sampling time are detailed in Table S1 in the Supplement.
After measuring CO2 fluxes in the field, the used plant fractions were collected and stored in a fridge until the sampled area was determined in the laboratory (within the next 24 h). To quantify the photosynthetic area, it was taken into account that only half of the stem and one side of the leaves received direct sunlight inside the leaf cuvette during daytime measurements, in accordance with Redondo-Gómez et al. (2007, 2010). However, for nighttime measurements, the entire stem and both sides of the leaves were taken into consideration. The instantaneous NER was expressed as , where m−2 refers to tissue area. Intrinsic water-use efficiency (iWUE) of green tissues was calculated for midday measurements as the ratio between photosynthetic rates and gs.
2.3 Seasonal carbon (CO2 and CH4) fluxes from soil
2.3.1 Soil CO2 measurements
Measurements of soil CO2 fluxes were performed during 2017, every 1.5 months, using the soda lime method (Edwards, 1982), which is based on the capacity of the soda lime to absorb CO2. This method gives a reliable and integrative measurement of soil CO2 fluxes throughout the whole day (Keith and Wong, 2006). In the field, five static opaque chambers per habitat (PVC cylinders of 11 cm in diameter and 13 cm in height) were inserted 5 cm into the soil (Fig. S3). At midday of each sampling day, an open glass vessel containing soda lime, previously oven-dried at 105 °C and weighed, was placed inside each chamber, and then chambers were immediately closed. After approximately 24 h, the glass vessel was hermetically sealed, collected, oven-dried at 105 °C, and weighed. The CO2 absorbed by the soda lime was calculated by multiplying the weight gain by 1.69 as a water correction factor (Emran et al., 2012; Grogan, 1998). Daily soil respiration rates (SR; ) were calculated as follows:
where SLf is the soda lime dry weight (in g) after 24 h in the field, SLi is the initial soda lime dry weight (in g) before being placed in the field, A is the soil surface area within the chamber (m2), and t is the time (in days) that soda lime remained in the field.
When the soil was flooded (i.e., covered with water), taller opaque chambers were used (Fig. S3), and soil CO2 fluxes were measured by collecting air samples from inside the chamber after 24 h of being hermetically closed. The CO2 concentration of these samples was analyzed at the Laboratory of Chemical and Environmental Engineering of the Research Technical Services of the University of Girona (UdG), using a gas chromatograph (Agilent 7890A, Agilent Technologies USA) equipped with a thermal conductivity detector. Control air samples were also taken just before closing the chambers. Daily soil respiration rates measured by gas chromatography (SR; ) were calculated as follows:
where Wf is the amount of CO2 (grams) in the air inside the chamber after 24 h of being closed, Wi is the initial amount of CO2 (grams) in the air inside the chamber before being closed, A is the soil surface area within the chamber (m2), and t is the time (in days) that the chamber remained closed. Wf and Wi were estimated from volumetric concentration (%) considering the air volume inside the chamber on each sampling date.
Gas chromatography analyses were not used to estimate soil respiration when the soil was not flooded because temperature and humidity variations throughout the day and night could affect the concentration of gas components in the sample (Rochette and Hutchinson, 2005). This is not a problem when using the soda lime method, which therefore can integrate soil CO2 fluxes over long periods, such as 24 h (Keith and Wong, 2006). The number of flooded and non-flooded plots and the method used on every sampling day are detailed in Table S2.
2.3.2 Soil CH4 measurements
Methane fluxes between the atmosphere and the soil surface (or the water surface when the soil was flooded) were estimated using the same opaque chambers used to measure soil CO2 fluxes. After 24 h of being hermetically closed, air samples from inside the chambers were collected and CH4 concentration was analyzed by gas chromatography at the Laboratory of Chemical and Environmental Engineering of the UdG Research Technical Services, with the same gas chromatograph used to determine SR when soil was flooded. Control air samples were also taken just before closing the chambers.
Daily soil methane fluxes (SMFs; ) were calculated as follows:
where Wmf is the milligrams of CH4 in the air inside the chamber after 24 h of being closed, Wmi is the initial milligrams of CH4 in the air inside the chamber before being closed, A is the soil surface area within the chamber (m2), and t is the time (in days) that the chamber remained closed. Wmf and Wmi were estimated from volumetric concentration (%) considering the air volume inside the chamber on each sampling date.
Soil CH4 and CO2 fluxes (with soda lime or gas chromatography) were measured on the same days. When soil was flooded, the same air samples were used to estimate both CO2 and CH4 fluxes.
2.3.3 Carbon mineralization quotient
The carbon mineralization quotient (Qmin) represents the fraction of soil organic carbon (SOC) mineralized in a given period of time (Pinzari et al., 1999), thus being considered a carbon sequestration index. Specifically, it represents the carbon that is emitted to the atmosphere under inorganic forms (CO2 and CH4) in relation to the carbon that is stored in the soil (SOC) at a certain depth. The soil depth considered was the first 20 cm, since it was previously determined that most SOC is stored there (Carrasco-Barea et al., 2023).
Daily carbon mineralization quotients (Qmin) were calculated for each sampling date following this equation:
where C_CO2 represents the carbon emitted as CO2 per gram of soil (), C_CH4 represents the carbon emitted as CH4 (), and SOC is the soil organic carbon of the first 20 cm of depth (mg SOC g soil−1). C_CO2 and C_CH4 were calculated multiplying the amount of CO2 and CH4 emitted by 12/44 and 12/16, respectively, with 12 being the molecular weight of carbon, 44 being the molecular weight of CO2, and 16 being the molecular weight of CH4. To convert emissions per unit area to emissions per gram of soil, we estimated the volume of soil beneath the chamber by multiplying the chamber area by the considered soil depth (20 cm) and then multiplying this volume by soil bulk density (g soil cm−3). SOC values were taken from previous measurements performed in July 2015 and 2016 in the same experiment (Table S3) after observing that these values exhibited stability and remained constant over the studied years (Carrasco-Barea et al., 2023).
2.4 Environmental measurements
For each sampling date and measurement location, the following soil environmental parameters were monitored: soil temperature (Ts) for the first 12 cm of depth (Digital Portable Thermometer AI-368, Acez, Singapore), soil volumetric water content (VWC) by means of a 20 cm rod (Field Scout TDR 300 soil moisture meter, Spectrum Technologies Inc, USA), and soil electrical conductivity (EC) (conductivity meter 254, Crison Instruments, Spain). Since the high EC values of these soils could affect VWC measurements, VWC values were corrected by obtaining calibration curves between TDR readings and the soil water content measured directly by determining soil weight loss over time (and considering the soil bulk density to convert data into volumetric content) in undisturbed soil samples.
Climatic data (maximum and minimum air temperature, air relative humidity, and vapor pressure deficit) were obtained from l'Estartit meteorological station (Estacions meteorològiques de l'Estartit i Torroella de Montgrí) located 2.5 km from La Pletera salt marsh.
2.5 Data analyses and statistics
To evaluate whether the studied plant species differed in their instantaneous net CO2 exchange rates (NERs), stomatal conductance, and intrinsic water-use efficiency, two-way ANOVAs were performed for each sampled time of the day, using species and sampling day as fixed factors. When the interaction between factors was significant, differences among species (for each sampling day) and sampling days (within each species) were evaluated by means of one-way ANOVAs.
To evaluate differences among habitats in soil respiration (SR), methane fluxes (SMF), carbon mineralization quotients (Qmin), soil temperature (Ts), electrical conductivity (EC), and volumetric water content (VWC), mixed models were performed using habitat as a fixed factor, sampling day as a repeated factor (repeated measures), and plots as a random factor. The interaction between habitat and sampling date was also included. When this interaction was significant, differences among habitats were tested for each sampling day by means of one-way ANOVAs, except for March and December, since, in these months, the glasswort sward had only two non-flooded plots; therefore, non-parametric Kruskal–Wallis tests were used.
Correlations were performed between (a) midday NERs of the perennial species (S. fruticosa, H. portulacoides, and E. atherica) and edaphic (VWC and EC) and climatic (maximum air temperature, air relative humidity, and vapor pressure deficit) parameters, (b) night NERs and minimum air temperature, (c) soil carbon fluxes (SR, SMF) and edaphic parameters (VWC, EC, and Ts) measured during the entire year, and (d) soil carbon fluxes in July (SR, SMF) and soil organic carbon (SOC) and total nitrogen content (TN) measured previously in July 2015 and 2016 in the same experiment (Carrasco-Barea et al., 2023). Pearson's correlation tests were usually applied, although the Spearman's rank correlation coefficient was used when data did not follow a normal distribution.
The Shapiro–Wilk test was used to check the normality of data, while Levene's test was applied to evaluate the homogeneity of variances. When factors (species, sampling day, and/or habitat) were significant, Tukey's HDS post hoc tests or pairwise comparisons (when data did not accomplish the assumptions of normality and/or homoscedasticity) were performed. For all the statistical tests, the significance level considered was p<0.05. Statistical analyses were performed using SPSS software (IBM SPSS statistics, Corporation, Chicago, USA).
3.1 Seasonal CO2 fluxes from vegetation
Differences among species in instantaneous NERs from green tissues depended on the time of the day and the sampling day. Specifically, E. atherica had the highest photosynthetic rates (negative NERs) in March and April after sunrise with no significant differences to S. fruticosa in April (Fig. 1a) and throughout the year at midday, except in June and July, when S. patula showed the highest values (Fig. 1c), corresponding to its growth period. Before sunset, the highest photosynthetic rates were recorded for E. atherica and S. fruticosa in February and March, for S. patula in June, and again for E. atherica during the rest of the year (Fig. 1e). At night, the highest respiration values (positive NERs) were found in August and November for E. atherica and S. fruticosa (Fig. 1g).
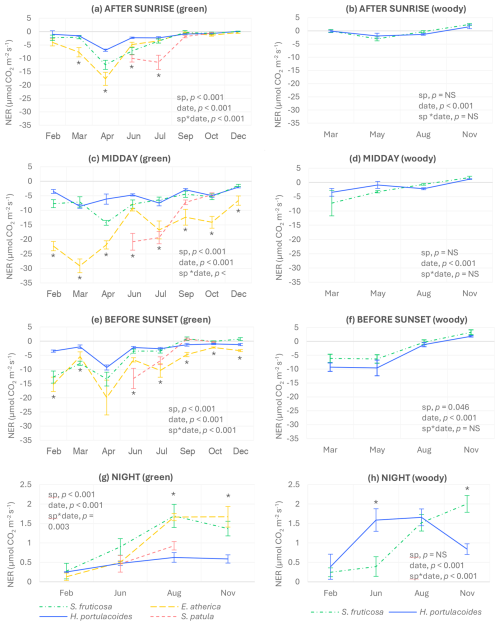
Figure 1Instantaneous net CO2 exchange rates (NERs) of the four studied species after sunrise (a, b), at midday (c, d), before sunset (e, f), and at night (g, h) for green and woody tissues. Negative values indicate net photosynthetic activity, while positive values indicate net respiration. Bars represent standard errors (n=4 after sunrise, before sunset, and at night; n=6 at midday). Significant p-values for the species and sampling date factors and their interactions (according to the two-way-ANOVA results) are also shown. Asterisks indicate significant differences among species in each sampling date (p<0.05), and they are depicted only when the interaction between species and sampling date was significant. NS: not significant.
As expected, the maximum photosynthetic activities of the green tissues of the three perennial species (S. fruticosa, E. atherica, and H. portulacoides) were recorded in spring (March or April; Fig. S4). Moreover, the four species studied showed, in general, the highest photosynthetic rates at midday, although differences with the other times of the day were not always significant (Figs. 1 and S4). In fact, seasonal patterns of photosynthetic activity were similar at the three times of day sampled for S. fruticosa and S. patula, while, in the case of E. atherica and H. portulacoides, midday patterns differed from those measured after sunrise and before sunset (Fig. S4).
Interestingly, thin woody stems of S. fruticosa and H. portulacoides had net CO2 uptake during all the light sampling times and days measured except in November, with both species showing similar values throughout the year after sunrise and at midday (Fig. 1b and d). Before sunset, H. portulacoides generally had lower values than S. fruticosa (Fig. 1f), while, at night, H. portulacoides showed lower respiration values than S. fruticosa in November but higher in June (Fig. 1h).
No significant correlations were found between midday NERs and soil VWC, soil EC, maximum air temperature, air relative humidity, or air vapor pressure deficit and between night NERs and minimum air temperature for any of the three perennial species studied (results not shown).
Seasonal stomatal conductance and intrinsic water-use efficiency of green tissues at midday
During most of the year, E. atherica showed the highest values of gs at midday, while H. portulacoides had the lowest (Fig. 2a). In July and October, no significant differences in gs were found among the four species studied. With regard to intrinsic water-use efficiency, differences among species were significant only in September, when S. fruticosa showed lower values than the other three species (Fig. 2b).
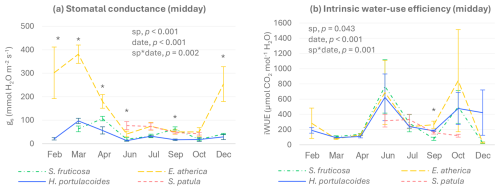
Figure 2Stomatal conductance (a) and intrinsic water-use efficiency (photosynthesis/stomatal conductance) (b) of green tissues of S. fruticosa, E. atherica, H. portulacoides, and S. patula at midday (mean ± SE, n=6). February values of S. fruticosa are missing because gs values were too low to be properly determined. Significant p-values for the species and sampling date factors and their interactions (according to the two-way-ANOVA results) are also shown. Asterisks indicate significant differences among species within each sampling day (p<0.05).
Concerning seasonal patterns, the three perennial species presented the highest gs values in spring (March or April), while no significant differences were observed among sampling dates for S. patula. iWUE values were generally higher in June and October for the three perennial species, although, due to the high variability found, differences to most of the other dates were not significant. In the case of S. patula, the highest iWUE values were observed in June and July.
3.2 Seasonal carbon fluxes from soil
3.2.1 Soil environmental parameters
The highest Ts values were registered during late spring and summer (Fig. 3a), in agreement with the highest air temperatures recorded during these months (Estacions meteorològiques de l'Estartit i Torroella de Montgrí). From July to December, soil temperatures were higher in the glasswort sward than in the two other habitats. Significant differences in VWC of the soil were only detected for the halophilous scrub, with the highest values being found in October and the lowest in March and June (Fig. 3b). No significant differences in VWC were found among habitats. Nevertheless, EC was significantly higher in the glasswort sward, followed by the halophilous scrub and the salt meadow, which was the least saline (Fig. 3c). Overall, the highest values of EC were recorded in June, although these values did not differ significantly from those obtained in July and September.
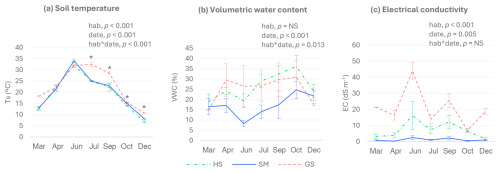
Figure 3Soil temperature (a), volumetric water content (b), and electrical conductivity (c) of the plots where soil carbon fluxes were measured for each sampling date and habitat. Only non-flooded plots are considered. HS: halophilous scrub; SM: salt meadow; GS: glasswort sward. Bars represent ± standard errors (n=5, except in the glasswort sward in March and December in which n=2). Significant p-values for the habitat and date factors and their interactions (according to the two-way-ANOVA results) are also shown. Asterisks indicate significant differences among habitats within a sampling date (p<0.05), being depicted only when the interaction between habitat and sampling date was significant. Despite the interaction between habitat and sampling date being significant for VWC, the analyses of the differences among habitats within each sampling date did not report significant results. NS: not significant.
3.2.2 Soil CO2 and CH4 fluxes and soil carbon mineralization quotient
SR for non-flooded soils of the three salt marsh habitats ranged from 4.0 ± 0.03 to 19.4 ± 0.9 , with the highest values being recorded in July and the lowest in October and December (Fig. 4a). CO2 emissions were remarkably lower when soils were flooded. Regarding the differences among habitats, the halophilous scrub and the salt meadow showed significantly higher SR values than the glasswort sward.
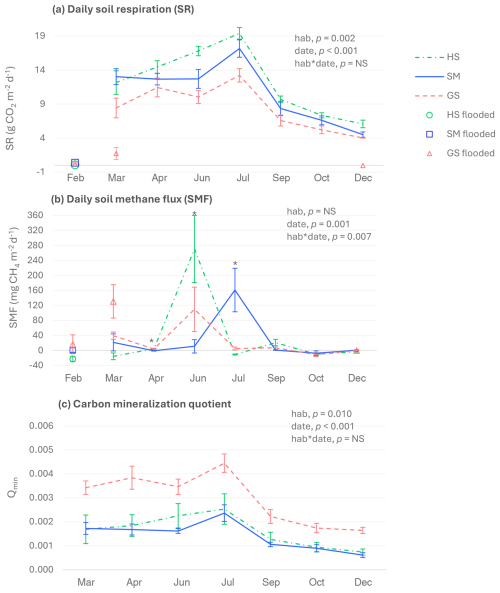
Figure 4Daily soil CO2 (a) and CH4 (b) fluxes and soil carbon mineralization quotient (c). HS: halophilous scrub; SM: salt meadow; GS: glasswort sward. Bars represent ± standard errors (n=5). In February, plots from the three habitats were flooded. In March and December, the glasswort sward had flooded (n=3) and non-flooded (n=2) plots. Daily soil CO2 fluxes were measured with the soda lime method in non-flooded soils and by gas chromatography when soils were flooded. Significant p-values for the habitat and sampling date factors and their interactions (according to the two-way ANOVA results) are also shown. Asterisks indicate significant differences among habitats within a sampling date (p<0.05). NS: not significant.
Remarkably high peaks of soil CH4 emissions were recorded in the three habitats, but negative values indicating net CH4 consumption were also observed (Fig. 4b). In the halophilous scrub, soil CH4 emissions were detected in April, June, and September, and the highest CH4 absorption was observed in February, when the soil was flooded. In the salt meadow and the glasswort sward, soil CH4 absorption was only detected in October. Maximum soil CH4 emissions for the salt meadow were recorded in July and for the glasswort sward in March and June in flooded and non-flooded soils, respectively.
For the three habitats, the highest soil carbon mineralization quotient (Qmin) was found in July, and the lowest was found in December (Fig. 4c). The glasswort sward presented the highest Qmin, while the halophilous scrub and the salt meadow showed similar values.
SR and SMF were positively correlated with soil temperature (Pearson's correlation coefficients (r) 0.568 and 0.557, respectively; p<0.01), while SR of July was positively correlated with SOC (r=0.997, p=0.045) and TN (r=0.999, p=0.026).
4.1 Carbon fluxes from vegetation
Elytrigia atherica, a herbaceous monocotyledonous species which is the dominant species of the salt meadow (Carrasco-Barea et al., 2023), presented the highest photosynthetic rates during most of the year, reaching 29 in March at midday, only being surpassed by the annual species Salicornia patula in June/July. E. atherica, as a Poaceae species, has dumbbell-shaped stomata, while the other two perennial species studied, Sarcocornia fruticosa and Halimione portulacoides, have kidney-shaped stomata. Dumbbell-shaped guard cells are surrounded by subsidiary cells that participate in the pore movements through physical interaction with guard cells, allowing a faster response and a wide pore aperture (Franks and Farquhar, 2007; Grantz and Zeiger, 1986). These conditions usually result in higher photosynthetic rates, especially in fluctuating environments such as salt marshes, when a fast stomatal response is an advantage for the photosynthetic process (Chen et al., 2017; Franks and Farquhar, 2007). In accordance with its higher photosynthetic rates, E. atherica also showed greater stomatal conductance values during almost the entire study period and higher leaf carbon concentration (Carrasco-Barea et al., 2023) compared to the other two perennial species. Previous studies in salt marshes have also reported higher net photosynthetic rates in monocotyledonous grasses compared to succulent Chenopodiaceae species (Kuramoto and Brest, 1979; Nieva et al., 1999; Pearcy and Ustin, 1984).
In the case of S. fruticosa, the maximum mean photosynthetic rate recorded at La Pletera salt marsh was 14.3 ± 0.8 , which was higher than that obtained in other studies, where maximum values were between 3–6 (Abdulrahman and Williams, 1981; Nieva et al., 1999; Redondo-Gómez et al., 2006; Redondo-Gómez and Mateos-Naranjo, 2010). However, these previous studies were performed with potted plants, collected from field sites and then cultivated under controlled conditions of light, temperature, and soil salinity. The same was true for E. atherica and S. patula, since their maximum photosynthetic rates at La Pletera (29.1 ± 2.4 and 20.8 ± 2.9 , respectively) were higher than those previously reported for E. atherica (18 ; Rozema and Diggelen, 1991) and for the annual species Salicornia ramosissima (14 ; Pérez-Romero et al., 2018) grown under controlled conditions. On the contrary, the maximum mean photosynthetic rate obtained for H. portulacoides at La Pletera salt marsh (9.3 ± 0.8 ) was slightly lower than the values previously obtained for the same species (15 to 18 ) growing under controlled (Redondo-Gómez et al., 2007) or field (Das Neves et al., 2008) conditions. Studies reporting photosynthetic rates of dominant salt marsh plant species under field conditions are scarce, and the values obtained often diverge substantially from those recorded under controlled conditions. This disparity can be a serious shortcoming for the development of accurate predictive models regarding the carbon balance of these ecosystems.
The elevated photosynthetic rates found in the most common plant species of La Pletera would explain their high mean maximum water use efficiency values (843 ± 671, 767 ± 351, 624 ± 307, and 331 ± 70 µmol CO2 mol−1 H2O for E. atherica, S. fruticosa, H. portulacoides, and S. patula, respectively), which exceed those reported under natural conditions for other salt marsh species, such as Spartina densiflora (around 100 µmol CO2 mol−1 H2O; Nieva et al., 2003), Halimione portulacoides, and Limoniastrum monopetalum (around 400 µmol CO2 mol−1 H2O; Das Neves et al., 2008), and for Salicornia ramosissima under controlled conditions (around 100 µmol CO2 mol−1 H2O; Pérez-Romero et al., 2018). In general, the perennial halophytic species studied (S. fruticosa, E. atherica, and H. portulacoides) presented their highest photosynthetic activity in March and/or April, which coincides with their growing season and with favorable environmental conditions (maximum temperatures around 18 °C; Estacions meteorològiques de l'Estartit i Torroella de Montgrí), as was also found for a non-tidal population of Spartina alterniflora located in the upper part of a tidal salt marsh in Portugal (Nieva et al., 2003). Interestingly, photosynthetic rates of the studied species at La Pletera were much lower in autumn than in spring, despite the environmental parameters, such as temperature and soil moisture, also being favorable for photosynthesis in autumn, especially in October, where the maximum temperature was 21 °C and the soil VWC was even higher than in March and April (Estacions meteorològiques de l'Estartit i Torroella de Montgrí). A possible explanation might be related to the high accumulation of ions and soluble carbohydrates in these species after a salt stress period, which occurs in the Mediterranean salt marshes during summer (Gil et al., 2011, 2014; Redondo-Gómez et al., 2007). In dicotyledonous species, high-salinity conditions can induce the accumulation of Na+ and Cl− in the cytoplasm (Munns, 1993), affecting photosynthesis (Almeida et al., 2017; Chaves et al., 2009). In addition, the accumulation of high intracellular levels of soluble carbohydrates as a salt-tolerant mechanism in salt marsh monocotyledonous species (Gil et al., 2013) can promote a feedback inhibition of photosynthesis (Munns, 1993).
Thin woody tissues (stem diameter <3 mm) of S. fruticosa and H. portulacoides also showed photosynthetic activity, especially in March and May and before sunset, with values of photosynthesis reaching 12 . These values are in agreement with data reported for Californian evergreen species (Saveyn et al., 2010) or for savannah shrubs and trees (Cernusak et al., 2006; Levy and Jarvis, 1998). As occurs with the woody stems of these other species, the thin woody stems of S. fruticosa and H. portulacoides plants growing at La Pletera salt marsh presented a layer of green cells under the periderm (Fig. S5). This layer of cells would likely be responsible for the recorded photosynthesis by using the light that passes through the thin bark and the CO2 that penetrates through lenticels. Taking into account that woody stems are typically overlooked in studies focused on characterizing CO2 exchange in vegetation, the present results highlight the importance of measuring and incorporating the photosynthetic activity of thin stems into such investigations. This is particularly crucial for ecosystems like the Mediterranean salt marshes, dominated by succulent Chenopodiaceae species in the form of the genus Sarcocornia, in which the woody fraction represents a significant fraction of the plant aerial biomass (Carrasco-Barea et al., 2023).
Regarding night respiration rates, the highest values for the four species were recorded in summer (August) and/or autumn (November), with those found for the green tissues of S. fruticosa and E. atherica during these months being especially elevated. Night respiration of these species seems not to be directly affected by air temperature, since correlations between night NER and minimum air temperature were not significant for any of the three perennial species. In E. atherica and S. fruticosa, the high values of night respiration registered in August coincide with their flowering period and thus with a high energetic demand (Bustan and Goldschmidt, 1998; Lambers et al., 2008). In the case of S. fruticosa, flowers, which are very abundant and small, remained inside the chamber during the measurements, and this could have contributed to increased respiration rates in August (Bustan and Goldschmidt, 1998). In November, respiration rates were also very high despite the minimum temperature being much colder (4.6 °C) than in August (22.2 °C) and similar to February (5.9 °C) (Estacions meteorològiques de l'Estartit i Torroella de Montgrí). High night respiration values in autumn might be explained, at least partially, by the accumulation of soluble carbohydrates and/or by the increase in the chloroplast redox status (Atkin et al., 2005; Koch, 1996) due to low temperatures, although more research is needed to clarify this.
4.2 Carbon fluxes from soil
Soil respiration (SR) values recorded at the halophilous scrub and the salt meadow were higher than those found at the glasswort sward. These differences might be related to the soil C and/or N content, which can affect the microorganism-mediated soil organic matter decomposition (Gougoulias et al., 2014; Oertel et al., 2016). In fact, positive correlations between CO2 emissions and soil organic carbon (SOC) or total nitrogen (TN) have been found for coastal saline soils (Li et al., 2019; Wang et al., 2016) and for soils of other terrestrial ecosystems (Merbold et al., 2011; Shi et al., 2014). In our study, a positive correlation was found between July SR and SOC or TN content, reinforcing the idea that differences in SR among habitats would be related with the higher SOC and TN content found in the halophilous scrub and the salt meadow compared to the glasswort sward (Table S3). Previous studies have shown that most of the CO2 produced during decomposition is derived from organic material recently incorporated to the soil, with only a small fraction (approx. 10 %) of soil respiration being derived from the decomposition of older, more recalcitrant carbon compounds (Giardina et al., 2004; Trumbore, 2000). Considering this, the lower soil CO2 emissions recorded in the glasswort sward would be in accordance with its more humified and stable soil organic matter (Gispert, unpublished data) and the scarce amount of litter found in this habitat (Carrasco-Barea et al., 2023). In addition, root respiration could have contributed to the high soil respiration found in the halophilous scrub and the salt meadow habitats, since S. fruticosa, H. portulacoides, and E. atherica have superficial root systems. On the contrary, the sparse vegetation (which is only alive during a few months) of the glasswort sward and the poorly developed root system of its dominant species, S. patula, would make the contribution of roots to soil respiration in this habitat negligible. Despite the halophilous scrub and the salt meadow having higher soil CO2 emissions than the glasswort sward, they showed lower mineralization quotients due to a much greater amount of SOC (Table S3), which was in accordance with a much higher aboveground, belowground, and litter biomass (Carrasco-Barea et al., 2023). Hence, our results would indicate that soils of the halophilous scrub and the salt meadow would have a higher carbon sequestration potential, despite their higher soil carbon emissions.
Table 1Published data on in situ daily soil CO2 and CH4 emissions from salt marshes at different locations. Values without parentheses indicate maximum values of CO2 and CH4 emissions, while values in parentheses indicate annual averages. A rank of mean maximum values is given when emissions have been measured in more than one area or habitat within the same salt marsh. When C emissions were measured in zones experiencing different degrees of tidal influence at the same tidal salt marsh, the term non-tidal is employed to indicate areas where flooding occurs only at certain times of year (instead of being flooded daily by tides). ND: no data; SD: soda lime; GC: gas chromatography; IRGA: infrared gas analyzer.
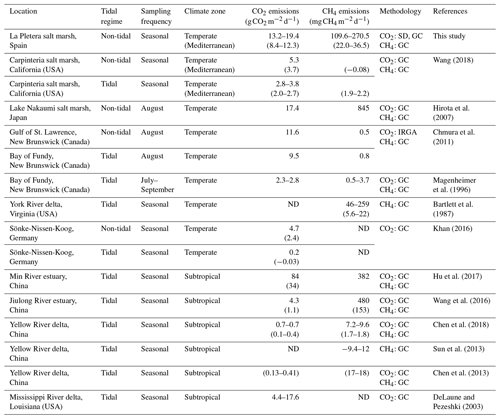
Daily soil respiration presented a similar seasonal pattern in the three habitats, with the highest values being recorded in summer (July), which agrees with the positive correlation found between SR and soil temperature. These findings are consistent with numerous previous studies in which the highest soil CO2 emissions are produced in the warmest season, since high temperatures enhance metabolic activity of soil microbes (Chen et al., 2018; Hu et al., 2017; Wang et al., 2016). In general, our estimations of daily soil CO2 emissions were in the upper part of the range previously published for salt marshes (Table 1), except for the study of Hu et al. (2017). One relevant difference between La Pletera salt marsh and all the salt marshes considered in Table 1, except the one studied by Hirota et al. (2007), is the daily tidal flood. In tidal salt marshes, flooding occurs once or twice every day, while it is occasional at La Pletera, only 1–2 times per year, leaving the soil flooded for some days in the salt meadow and several weeks or even months in the glasswort sward (Pascual and Martinoy, 2017). This allows an airier soil at La Pletera, which favors microbial respiration. The study of Hirota et al. (2007), performed in a non-tidal salt marsh, reported daily soil CO2 emissions similar to those found at La Pletera. Nevertheless, it should be noted that the methodology used to determine soil CO2 and CH4 fluxes in this study differs from the one generally employed in the studies listed in Table 1, since most of them used gas chromatography for both CH4 and CO2 measurements. Thus, an effect caused by these methodological differences cannot be excluded.
Previous studies under field (Kathilankal et al., 2008; Moffett et al., 2010) or laboratory (Jones et al., 2018; Wang et al., 2019) conditions support a negative effect of flooding on soil CO2 emissions, as has been found at La Pletera. At La Pletera, the reduction in soil CO2 emission during flooding conditions can be explained by the fact that CO2 molecules diffuse 10 000 times slower in water than in air (Kathilankal et al., 2008). However, since different methods were used to measure soil respiration in flooded and non-flooded soils, this comparison should be interpreted with caution.
Regarding soil methane fluxes, the highest emissions were detected in the warmest months, being recorded in June for the halophilous scrub and the glasswort sward and in July for the salt meadow. The same seasonal trend has broadly been found in other salt marsh studies, and it would be explained by the positive effect of higher temperatures on microbial activity (Bartlett et al., 1987; Chen et al., 2018; Hu et al., 2017; Wang et al., 2016; Yuan et al., 2015). Despite the fact that no soil anaerobic conditions, necessary for the growth of methanogens, would be expected during summer because of the low soil VWC at La Pletera salt marsh, anaerobic conditions could be found at the water table level, which is around 30–50 cm deep (Menció et al., 2023). At this depth, there is still organic matter susceptible to being decomposed (Amorós, 2018), which could promote CH4 production. The CH4 produced in the anaerobic zone can easily diffuse through air-filled macropores, especially during summer, when high temperatures at the soil surface would promote soil water evaporation to the atmosphere (Denier Van Der Gon et al., 1996).
CH4 emissions are, in general, negatively affected by salinity, usually being higher in freshwater wetlands than in salt marshes (Bartlett and Harriss, 1993; Hu et al., 2017; Poffenbarger et al., 2011). However, according to the Poffenbarger et al. (2011) revision, the soil salinity threshold that would reduce CH4 emissions would be around 18 ‰. Indeed, Poffenbarger et al. (2011) found significantly lower CH4 emission values (3 ± 5 ) in polyhaline salt marshes (salinity >18 ‰) compared to mesohaline (salinity 5 ‰–18 ‰), oligohaline (salinity 0.5 ‰–5 ‰), and freshwater (salinity 0 ‰–0.5 ‰) (44 ± 30, 411 ± 578, and 115 ± 208 , respectively) marshes. In the present study, we detected relatively high soil CH4 emissions in all three habitats, similar to those reported for freshwater marshes by Poffenbarger et al. (2011), which could be explained by the low salinity (0.86 ‰) of the water table of La Pletera (Menció et al., 2017). As summer progresses (from July onwards), the absence of important rainfall episodes at La Pletera salt marsh, together with the sea water intrusion, moves the saltwater wedge inland, increasing groundwater salinity until levels similar to those of the sea are reached (approximately 32 ‰) (Menció et al., 2017). Therefore, the sharp decrease in CH4 emissions recorded in July in the halophilous scrub and the glasswort sward (the closest habitats to the sea) might be the consequence of more saline conditions in the water table. This would not be the case in the salt meadow, the most distant habitat from the sea, where maximum CH4 emissions were recorded in July. Some previous studies in salt marshes with a low-salinity water table, such as those of Hirota et al. (2007) (2 ‰), Bartlett et al. (1987) (0 ‰–12 ‰), Hu et al. (2017) (4 ‰), and Wang et al. (2016) (13 ‰–21 ‰), have also found high CH4 emissions (Table 1), although it is worth mentioning that only Hirota et al. (2007) took samples after 24 h of chamber closure, as was done in the present study.
This study emphasizes the remarkable atmospheric CO2 removal capacity through photosynthesis exhibited by the four dominant non-tidal Mediterranean salt marsh species studied, especially by Elytrigia atherica. The green parts of these species had net CO2 uptake along the day during most of the year, with the recorded values being generally higher compared with previous data for the same or similar species, which would indicate a significant potential for carbon sequestration. Besides, the thin woody stems of Sarcocornia fruticosa and Halimione portulacoides had net CO2 uptake in most measurements, highlighting the importance of considering this fraction when characterizing daily and seasonal CO2 fluxes from these ecosystems.
The halophilous scrub and the salt meadow had higher soil CO2 emissions than the glasswort sward, and, in general, these values were higher than those reported for temperate and subtropical tidal salt marshes. Both soil CH4 absorption and emission were detected, with soil CH4 emissions being remarkably high. In general, CH4 emissions from La Pletera soils were higher than those reported for other salt marshes with high water table salinity but similar to those found in low-salinity salt marshes. Remarkably, soils from the halophilous scrub and the salt meadow presented lower mineralization quotients than those of the glasswort sward, suggesting a higher potential for carbon sequestration.
The high variability among species and habitats observed in the present study, along with the differences between La Pletera and other salt marshes, concerning the carbon cycle highlights the importance of increasing the availability of data on carbon fluxes from salt marshes. This is essential to be able to make more accurate predictions regarding carbon emissions from these ecosystems, emphasizing the importance of further field research on this subject.
Data obtained for carbon fluxes can be downloaded at https://doi.org/10.34810/data1836 (Carrasco-Barea et al., 2024), while climatic data are available at http://www.meteolestartit.cat (Pascual and Martinoy, 2018a, b).
The supplement related to this article is available online at: https://doi.org/10.5194/bg-22-289-2025-supplement.
LCB: conceptualization, data curation, methodology, formal analysis, investigation, and writing (original draft and review and editing). DV: conceptualization, writing (review and editing), and supervision. MG: conceptualization, writing (review and editing), and supervision. XDQ: conceptualization and funding acquisition. HB: methodology. LL: conceptualization, writing (review and editing), and supervision.
The contact author has declared that none of the authors has any competing interests.
Publisher's note: Copernicus Publications remains neutral with regard to jurisdictional claims made in the text, published maps, institutional affiliations, or any other geographical representation in this paper. While Copernicus Publications makes every effort to include appropriate place names, the final responsibility lies with the authors.
We are grateful to the Parc Natural del Montgrí, les Illes Medes i el Baix Ter, for the support received in performing this study.
This research has been supported by the Life+ Program of the European Commission (Life Pletera; grant no. LIFE13NAT/ES/001001). Lorena Carrasco-Barea held a PhD grant (grant no. IFUdG2015) from the University of Girona.
This paper was edited by Tyler Cyronak and reviewed by two anonymous referees.
Abdulrahman, F. S. and Williams, G. J.: Temperature and salinity regulation of growth and gas exchange of Salicornia fruticosa (L.) L, Oecologia, 48, 346–352, 1981.
Almeida, D. M., Margarida Oliveira, M., and Saibo, N. J. M.: Regulation of Na+ and K+ homeostasis in plants: Towards improved salt stress tolerance in crop plants, Genet. Mol. Biol., 40, 326–345, https://doi.org/10.1590/1678-4685-gmb-2016-0106, 2017.
Amorós, M.: Avaluació de la capacitat de segrest de carboni del sòl en diferents hàbitats de la zona de la Pletera (L'Estartit), Universitat de Girona, 2018.
Antlfinger, A. E. and Dunn, E. L.: Seasonal patterns of CO2 and water vapor exchange of three salt marsh succulents, Oecologia, 43, 249–260, https://doi.org/10.1007/BF00344952, 1979.
Atkin, O. K., Bruhn, D., Hurry, V. M., and Tjoelker, M. G.: The hot and the cold: unravelling the variable response of plant respiration to temperature, Funct. Plant Biol., 32, 87–105, https://doi.org/10.1071/FP03176, 2005.
Bartlett, K., Bartlett, D., Harriss, R., and Sebacher, D.: Methane emissions along a salt marsh salinity gradient, Biogeochemistry, 4, 183–202, 1987.
Bartlett, K. B. and Harriss, R. C.: Review and assessment of methane emissions from wetlands, Chemosphere, 26, 261–320, https://doi.org/10.1016/0045-6535(93)90427-7, 1993.
Blunden, J. and Arndt, D. S.: State of the Climate in 2018, B. Am. Meteorol. Soc., 100, 1–305, 2019.
Bridgham, S. D., Megonigal, J. P., Keller, J. K., Bliss, N. B., and Trettin, C.: The carbon balance of North American wetlands, Wetlands, 26, 889–916, https://doi.org/10.1672/0277-5212(2006)26[889:TCBONA]2.0.CO;2, 2006.
Bustan, A. and Goldschmidt, E. E.: Estimating the cost of flowering in a grapefruit tree, Plant Cell Environ., 21, 217–224, https://doi.org/10.1046/j.1365-3040.1998.00267.x, 1998.
Carrasco-Barea, L., Verdaguer, D., Gispert, M., Font, J., Compte, J., and Llorens, L.: Carbon stocks in vegetation and soil and their relationship with plant community traits in a Mediterranean non-tidal salt marsh, Estuar. Coast., 46, 376–387, https://doi.org/10.1007/s12237-022-01155-w, 2023.
Carrasco-Barea, L., Verdaguer, D., Gispert Negrell, M. A., Quintana, X., Bourhis, H., and Llorens, L.: Replication Data for: “Seasonal carbon fluxes from vegetation and soil in a Mediterranean non-tidal salt marsh”, V2, CORA, Repositori de Dades de Recerca [data set], https://doi.org/10.34810/data1836, 2024.
Cernusak, L. A., Hutley, L. B., Beringer, J., and Tapper, N. J.: Stem and leaf gas exchange and their responses to fire in a north Australian tropical savanna, Plant Cell Environ., 29, 632–646, https://doi.org/10.1111/j.1365-3040.2005.01442.x, 2006.
Chaves, M. M., Flexas, J., and Pinheiro, C.: Photosynthesis under drought and salt stress: Regulation mechanisms from whole plant to cell, Ann. Bot., 103, 551–560, https://doi.org/10.1093/aob/mcn125, 2009.
Chen, Q., Guo, B., Zhao, C., and Xing, B.: Characteristics of CH4 and CO2 emissions and influence of water and salinity in the Yellow River delta wetland, China, Environ. Pollut., 239, 289–299, https://doi.org/10.1016/j.envpol.2018.04.043, 2018.
Chen, Q.-F., Ma, J.-J., Liu, J.-H., Zhao, C.-S., and Liu, W.: Characteristics of greenhouse gas emission in the Yellow River Delta wetland, Int. Biodeter. Biodegr., 85, 646–651, https://doi.org/10.1016/j.ibiod.2013.04.009, 2013.
Chen, Z. H., Chen, G., Dai, F., Wang, Y., Hills, A., Ruan, Y. L., Zhang, G., Franks, P. J., Nevo, E., and Blatt, M. R.: Molecular Evolution of Grass Stomata, Trends Plant Sci., 22, 124–139, https://doi.org/10.1016/j.tplants.2016.09.005, 2017.
Chmura, G. L.: What do we need to assess the sustainability of the tidal salt marsh carbon sink?, Ocean Coast. Manage., 83, 25–31, https://doi.org/10.1016/j.ocecoaman.2011.09.006, 2011.
Chmura, G. L., Anisfeld, S. C., Cahoon, D. R., and Lynch, J. C.: Global carbon sequestration in tidal, saline wetland soils, Global Biogeochem. Cy., 17, 1111, https://doi.org/10.1029/2002GB001917, 2003.
Chmura, G. L., Kellman, L., and Guntenspergen, G. R.: The greenhouse gas flux and potential global warming feedbacks of a northern macrotidal and microtidal salt marsh, Environ. Res. Lett., 6, 1–6, https://doi.org/10.1088/1748-9326/6/4/044016, 2011.
Ciais, P., Sabine, C., Bala, G., Bopp, L., Brovkin, V., Canadell, J., Chhabra, A., DeFries, R., Galloway, J., Heimann, M., Jones, C., Le Quéré, C., Myneni, R. B., Piao, S., and Thornton, P.: Carbon and other biogeochemical cycles, in: Climate Change 2013: The Physical Science Basis. Contribution of Working Group I to the Fifth Assessment Report of the Intergovernmental Panel on Climate Change, edited by: Stocker, T. F., Qin, D., Plattner, G. K., Tignor, M., Allen, S. K., Boschung, J., Nauels, A., Xia, Y., Bex, V., and Midgley, P. M., Cambridge University Press, Cambridge, United Kingdom and New York, USA, https://doi.org/10.1017/CBO9781107415324.015, 2013.
Dalal, R. C. and Allen, D. E.: Greenhouse gas fluxes from natural ecosystems, Aust. J. Bot., 56, 369–407, https://doi.org/10.1071/BT07128, 2008.
Das Neves, J. P. C., Ferreira, L. F. P., Vaz, M. M., and Gazarini, L. C.: Gas exchange in the salt marsh species Atriplex portulacoides L. and Limoniastrum monopetalum L. in Southern Portugal, Acta Physiol. Plant., 30, 91–97, https://doi.org/10.1007/s11738-007-0094-6, 2008.
Davy, A. J., Bishop, G. F., Mossman, H., Redondo-Gómez, S., Castillo, J. M., Castellanos, E. M., Luque, T., and Figueroa, M. E.: Biological Flora of the British Isles: Sarcocornia perennis (Miller) A. J. Scott, J. Ecol., 94, 1035–1048, https://doi.org/10.1111/j.1365-2745.2006.01156.x, 2006.
DeLaune, R. D. and Pezeshki, S. R.: The role of soil organic carbon in maintaining surface elevation in rapidly subsiding U.S. Gulf of Mexico coastal marshes, Water Air Soil Poll., 3, 167–179, https://doi.org/10.1023/A:1022136328105, 2003.
DeLaune, R. D., Smith, C. J., and Patrick, W. H.: Methane release from Gulf coast wetlands, Tellus B, 35, 8–15, https://doi.org/10.1111/j.1600-0889.1983.tb00002.x, 1983.
Denier Van Der Gon, H. A. C., Breemen, N. Van, Neue, H. U., Lantin, R. S., Aduna, J. B., Alberto, M. C. K., and Wassmalln, R.: Release of entrapped methane from wetland rice fields upon soil drying, Global Biogeochem. Cy., 10, 1–7, https://doi.org/10.1029/95GB03460, 1996.
Drake, B. G.: Photosynthesis of salt marsh species, Aquat. Bot., 34, 167–180, 1989.
Duarte, B., Santos, D., Silva, H., Marques, J. C., Caçador, I., and Sleimi, N.: Light-dark O2 dynamics in submerged leaves of C3 and C4 halophytes under increased dissolved CO2: Clues for saltmarsh response to climate change, AoB Plants, 6, 1–15, https://doi.org/10.1093/aobpla/plu067, 2014.
Edwards, N. T.: The use of soda-lime for measuring respiration rates in terrestrial systems, Pedobiologia, 23, 321–330, 1982.
Emran, M., Gispert, M., and Pardini, G.: Comparing measurements methods of carbon dioxide fluxes in a soil sequence under land use and cover change in North Eastern Spain, Geoderma, 170, 176–185, https://doi.org/10.1016/j.geoderma.2011.11.013, 2012.
Franks, P. J. and Farquhar, G. D.: The mechanical diversity of stomata and its significance in gas-exchange control, Plant Physiol., 143, 78–87, https://doi.org/10.1007/s12205-009-0039-2, 2007.
Geijzendorffer, I., Chazée, L., Gaget, E., Galewski, T., Guelmami, A., and Perennou, C.: Mediterranean Wetlands Outlook 2: solutions for sustainable Mediterranean wetlands, J. Mater. Process. Tech., 1, 1–82, 2018.
Giardina, C. P., Binkley, D., Senock, R. S., Fownes, J. H., and Ryan, M. G.: Belowground carbon cycling in a humid tropical forest decreases with fertilization, Oecologia, 139, 545–550, https://doi.org/10.1007/s00442-004-1552-0, 2004.
Gil, R., Lull, C., Boscaiu, M., Bautista, I., Lidón, A., and Vicente, O.: Soluble carbohydrates as osmolytes in several halophytes from a mediterranean salt marsh, Not. Bot. Horti Agrobo., 39, 9–17, https://doi.org/10.15835/NBHA3927176, 2011.
Gil, R., Boscaiu, M., Lull, C., Bautista, I., Lidón, A., and Vicente, O.: Are soluble carbohydrates ecologically relevant for salt tolerance in halophytes?, Funct. Plant Biol., 40, 805–818, https://doi.org/10.1071/FP12359, 2013.
Gil, R., Bautista, I., Boscaiu, M., Lidón, A., Wankhade, S., Sánchez, H., Llinares, J., and Vicente, O.: Responses of five Mediterranean halophytes to seasonal changes in environmental conditions, AoB Plants, 6, 1–18, https://doi.org/10.1093/aobpla/plu049, 2014.
Gougoulias, C., Clark, J. M., and Shaw, L. J.: The role of soil microbes in the global carbon cycle: Tracking the below-ground microbial processing of plant-derived carbon for manipulating carbon dynamics in agricultural systems, J. Sci. Food Agr., 94, 2362–2371, https://doi.org/10.1002/jsfa.6577, 2014.
Grantz, D. A. and Zeiger, E.: Stomatal responses to light and leaf-air water vapor pressure difference show similar kinetics in sugarcane and soybean, Plant Physiol., 81, 865–868, https://doi.org/10.1104/pp.81.3.865, 1986.
Grogan, P.: CO2 flux measurement using soda lime: correction for water formed during CO2 adsorption, Ecology, 79, 1467–1468, 1998.
Hirota, M., Senga, Y., Seike, Y., Nohara, S., and Kunii, H.: Fluxes of carbon dioxide, methane and nitrous oxide in two contrastive fringing zones of coastal lagoon, Lake Nakaumi, Japan, Chemosphere, 68, 597–603, https://doi.org/10.1016/j.chemosphere.2007.01.002, 2007.
Hu, M., Ren, H., Ren, P., Li, J., Wilson, B. J., and Tong, C.: Response of gaseous carbon emissions to low-level salinity increase in tidal marsh ecosystem of the Min River estuary, southeastern China, J. Environ. Sci., 52, 210–222, https://doi.org/10.1016/j.jes.2016.05.009, 2017.
Huckle, J. M., Potter, J. A., and Marrs, R. H.: Influence of environmental factors on the growth and interactions between salt marsh plants: effects of salinity, sediment and waterlogging, J. Ecol., 88, 492–505, https://doi.org/10.1046/j.1365-2745.2000.00464.x, 2000.
Ibañez, C., Curco, A., Day Jr., J. W., and Prat, N.: Structure and productivity of microtidal Mediterranean coastal marshes., in: Concepts and Controversies in Tidal Marsh Ecology, edited by: Weinstein, M. P. and Kreeger, D. A., Springer Netherlands, 107–136, https://doi.org/10.1007/0-306-47534-0, 2000.
Jones, S. F., Stagg, C. L., Krauss, K. W., and Hester, M. W.: Flooding alters plant-mediated carbon cycling independently of elevated atmospheric CO2 concentrations, J. Geophys. Res.-Biogeo., 123, 1976–1987, https://doi.org/10.1029/2017JG004369, 2018.
Kathilankal, J. C., Mozdzer, T. J., Fuentes, J. D., D'Odorico, P., McGlathery, K. J., and Zieman, J. C.: Tidal influences on carbon assimilation by a salt marsh, Environ. Res. Lett., 3, 1–6, https://doi.org/10.1088/1748-9326/3/4/044010, 2008.
Keith, H. and Wong, S. C.: Measurement of soil CO2 efflux using soda lime absorption: both quantitative and reliable, Soil Biol. Biochem., 38, 1121–1131, https://doi.org/10.1016/j.soilbio.2005.09.012, 2006.
Khan, H. R.: Influence of salt marsh ecosystem on the concentration and emission of CO2 from the wadden sea coast soil of Northern Germany, Bangladesh Journal of Scientific Research, 29, 101–109, 2016.
Koch, K. E.: Carbohydrate-modulated gene expression in plants, Annu. Rev. Plant Phys., 47, 509–540, https://doi.org/10.1146/annurev.arplant.47.1.509, 1996.
Kuramoto, R. T. and Brest, D. E.: Physiological response to salinity by four salt marsh plants, Botanical Gazette, 140, 295–298, 1979.
Laffoley, D. d'A. and Grimsditch, G.: The management of natural coastal carbon sinks, Gland, Switzerland, ISBN 978-2-8317-1205-5, 2009.
Lambers, H., Chapin III, F. S., and Pons, T. L.: Plant physiological ecology, Springer, New York, ISBN 978-1-4939-3705-9, 2008.
Lan, X., Thoning, K. W., and Dlugokencky, E. J.: Trends in globally-averaged CH4, N2O, and SF6 determined from NOAA Global Monitoring Laboratory measurements, https://doi.org/10.15138/P8XG-AA10, 2023a.
Lan, X., Trans, P., and Thoning, K. W.: Trends in globally-averaged CO2 determined from NOAA Global Monitoring Laboratory measurements, https://doi.org/10.15138/9N0H-ZH07, 2023b.
Levy, P. E. and Jarvis, P. G.: Stem CO2 fluxes in two Sahelian shrub species (Guiera senegalensis and Combretum micranthum), Funct. Ecol., 12, 107–116, https://doi.org/10.1046/j.1365-2435.1998.00156.x, 1998.
Li, Y., Wu, H., Wang, J., Cui, L., Tian, D., Wang, J., Zhang, X., Yan, L., Yan, Z., Zhang, K., Kang, X., and Song, B.: Plant biomass and soil organic carbon are main factors influencing dry-season ecosystem carbon rates in the coastal zone of the Yellow River Delta, PLoS One, 14, 1–16, https://doi.org/10.1371/journal.pone.0210768, 2019.
Livesley, S. J. and Andrusiak, S. M.: Temperate mangrove and salt marsh sediments are a small methane and nitrous oxide source but important carbon store, Estuar. Coast. Shelf S., 97, 19–27, https://doi.org/10.1016/j.ecss.2011.11.002, 2012.
Magenheimer, J. F., Moore, T. R., Chmura, G. L., and Daoust, R. J.: Methane and carbon dioxide flux from a macrotidal salt marsh, Bay of Fundy, New Brunswick, Estuaries, 19, 139, https://doi.org/10.2307/1352658, 1996.
Maricle, B. R. and Maricle, K. L.: Photosynthesis, stomatal responses, and water potential in three species in an inland salt marsh in Kansas, USA, Flora, 244–245, 1–7, https://doi.org/10.1016/j.flora.2018.05.001, 2018.
McGenity, T. J. and Sorokin, D. Y.: Methanogens and Methanogenesis in Hypersaline Environments, in: Biogenesis of Hydrocarbons, edited by: Stams, A. J. M. and Sousa, D. Z., Springer International Publishing, https://doi.org/10.1007/978-3-319-53114-4_12-1, 2018.
McLeod, E., Chmura, G. L., Bouillon, S., Salm, R., Björk, M., Duarte, C. M., Lovelock, C. E., Schlesinger, W. H., and Silliman, B. R.: A blueprint for blue carbon: Toward an improved understanding of the role of vegetated coastal habitats in sequestering CO2, Front. Ecol. Environ., 9, 552–560, https://doi.org/10.1890/110004, 2011.
Menció, A., Casamitjana, X., Mas-Pla, J., Coll, N., Compte, J., Martinoy, M., Pascual, J., and Quintana, X. D.: Groundwater dependence of coastal lagoons: The case of La Pletera salt marshes (NE Catalonia), J. Hydrol., 552, 793–806, https://doi.org/10.1016/j.jhydrol.2017.07.034, 2017.
Menció, A., Madaula, E., Meredith, W., Casamitjana, X., and Quintana, X. D.: Nitrogen in surface aquifer – Coastal lagoons systems: Analyzing the origin of eutrophication processes, Sci. Total Environ., 871, 161947, https://doi.org/10.1016/j.scitotenv.2023.161947, 2023.
Merbold, L., Ziegler, W., Mukelabai, M. M., and Kutsch, W. L.: Spatial and temporal variation of CO2 efflux along a disturbance gradient in a miombo woodland in Western Zambia, Biogeosciences, 8, 147–164, https://doi.org/10.5194/bg-8-147-2011, 2011.
Mitsch, W. J. and Gosselink, K. M.: Wetlands, 5th edn., Wiley, New York, ISBN 978-1-1186-7682-0, 2015.
Moffett, K. B., Wolf, A., Berry, J. A., and Gorelick, S. M.: Salt marsh-atmosphere exchange of energy, water vapor, and carbon dioxide: Effects of tidal flooding and biophysical controls, Water Resour. Res., 46, 1–18, https://doi.org/10.1029/2009WR009041, 2010.
Munns, R.: Physiological processes limiting plant growth in saline soils: some dogmas and hypotheses, Plant Cell Environ., 16, 15–24, https://doi.org/10.1111/j.1365-3040.1993.tb00840.x, 1993.
Nieva, F. J. J., Castellanos, E. M., Figueroa, M. E., and Gil, F.: Gas exchange and chlorophyll fluorescence of C3 and C4 saltmarsh species, Photosynthetica, 36, 397–406, https://doi.org/10.1023/A, 1999.
Nieva, F. J. J., Castillo, J. M., Luque, C. J., and Figueroa, M. E.: Ecophysiology of tidal and non-tidal populations of the invading cordgrass Spartina densiflora: Seasonal and diurnal patterns in a Mediterranean climate, Estuar. Coast. Shelf S., 57, 919–928, https://doi.org/10.1016/S0272-7714(02)00422-5, 2003.
Oertel, C., Matschullat, J., Zurba, K., Zimmermann, F., and Erasmi, S.: Greenhouse gas emissions from soils – A review, Chem. Erde-Geochem., 76, 327–352, https://doi.org/10.1016/j.chemer.2016.04.002, 2016.
Pascual, J. and Martinoy, M.: La pletera informe 2014–2017: Niveles de agua y salinidad, Torroella de Montgrí, Spain, 2017.
Pascual, J. and Martinoy, M.: Estacions meteorològiques de l'Estartit i Torroella de Montgrí, Resums climatològics – l'Estartit, https://meteolestartit.cat/resums/, last access: 6 October 2018a.
Pascual, J. and Martinoy, M.: Estacions meteorològiques de l'Estartit i Torroella de Montgrí, Dades recents, https://meteolestartit.cat/category/dades-recents/, last access: 6 October 2018b.
Pearcy, R. W. and Ustin, S. L.: Effects of salinity on growth and photosynthesis of three California tidal marsh species, Oecologia, 62, 68–73, https://doi.org/10.1007/BF00377375, 1984.
Pérez-Romero, J. A., Idaszkin, Y. L., Barcia-Piedras, J. M., Duarte, B., Redondo-Gómez, S., Caçador, I., and Mateos-Naranjo, E.: Disentangling the effect of atmospheric CO2 enrichment on the halophyte Salicornia ramosissima J. Woods physiological performance under optimal and suboptimal saline conditions, Plant Physiol. Bioch., 127, 617–629, https://doi.org/10.1016/j.plaphy.2018.04.041, 2018.
Pinzari, F., Trinchera, A., Benedetti, A., and Sequi, P.: Use of biochemical indices in the mediterranean environment: comparison among soils under different forest vegetation, J. Microbiol. Meth., 36, 21–28, https://doi.org/10.1016/S0167-7012(99)00007-X, 1999.
Poffenbarger, H. J., Needelman, B. A., and Megonigal, J. P.: Salinity influence on methane emissions from tidal marshes, Wetlands, 31, 831–842, https://doi.org/10.1007/s13157-011-0197-0, 2011.
Redondo-Gómez, S. and Mateos-Naranjo, E.: Photosynthetic responses to light intensity of Sarcocornia taxa (Chenopodiaceae), Russ. J. Plant Physl.+, 57, 887–891, https://doi.org/10.1134/S1021443710060191, 2010.
Redondo-Gómez, S., Wharmby, C., Castillo, J. M., Mateos-Naranjo, E., Luque, C. J., De Cires, A., Luque, T., Davy, A. J., and Enrique Figueroa, M.: Growth and photosynthetic responses to salinity in an extreme halophyte, Sarcocornia fruticosa, Physiol. Plantarum, 128, 116–124, https://doi.org/10.1111/j.1399-3054.2006.00719.x, 2006.
Redondo-Gómez, S., Mateos-Naranjo, E., Davy, A. J., Fernández-Muñoz, F., Castellanos, E. M., Luque, T., and Figueroa, M. E.: Growth and photosynthetic responses to salinity of the salt-marsh shrub Atriplex portulacoides, Ann. Bot.-London, 100, 555–563, https://doi.org/10.1093/aob/mcm119, 2007.
Redondo-Gómez, S., Mateos-Naranjo, E., Figueroa, M. E., and Davy, A. J.: Salt stimulation of growth and photosynthesis in an extreme halophyte, Arthrocnemum macrostachyum, Plant Biol., 12, 79–87, https://doi.org/10.1111/j.1438-8677.2009.00207.x, 2010.
Rochette, P. and Hutchinson, G. L.: Measurement of Soil Respiration in situ: Chamber Techniques, in: Micrometeorology in Agricultural Systems, edited by: Hatfield, J. L. and Baker, J. M., Madison, USA, 247–286, https://doi.org/10.1007/978-3-662-05171-9_3, 2005.
Rozema, J. and Diggelen, J. V.: A comparative study of growth and photosynthesis of four halophytes in response to salinity, Acta Oecol., 12, 673–681, 1991.
Saveyn, A., Steppe, K., Ubierna, N., and Dawson, T. E.: Woody tissue photosynthesis and its contribution to trunk growth and bud development in young plants, Plant Cell Environ., 33, 1949–1958, https://doi.org/10.1111/j.1365-3040.2010.02197.x, 2010.
Shi, W. Y., Yan, M. J., Zhang, J. G., Guan, J. H., and Du, S.: Soil CO2 emissions from five different types of land use on the semiarid Loess Plateau of China, with emphasis on the contribution of winter soil respiration, Atmos. Environ., 88, 74–82, https://doi.org/10.1016/j.atmosenv.2014.01.066, 2014.
Sun, Z., Jiang, H., Wang, L., Mou, X., and Sun, W.: Seasonal and spatial variations of methane emissions from coastal marshes in the northern Yellow River estuary, China, Plant Soil, 369, 317–333, https://doi.org/10.1007/s11104-012-1564-1, 2013.
Trumbore, S.: Age of soil organic matter and soil respiration: Radiocarbon constraints on belowground C dynamics, Ecol. Appl., 10, 399–411, https://doi.org/10.2307/2641102, 2000.
Wang, F., Kroeger, K. D., Gonneea, M. E., Pohlman, J. W., and Tang, J.: Water salinity and inundation control soil carbon decomposition during salt marsh restoration: An incubation experiment, Ecol. Evol., 9, 1911–1921, https://doi.org/10.1002/ece3.4884, 2019.
Wang, H., Liao, G., D'Souza, M., Yu, X., Yang, J., Yang, X., and Zheng, T.: Temporal and spatial variations of greenhouse gas fluxes from a tidal mangrove wetland in Southeast China, Environ. Sci. Pollut. R., 23, 1873–1885, https://doi.org/10.1007/s11356-015-5440-4, 2016.
Wang, J.: Carbon dioxide and methane emissions from a California salt marsh, University of California, Santa Barbara, 2018.
Warren, R. S. and Brockelman, P. M.: Photosynthesis, respiration, and salt gland activity of Distichlis spicata in relation to soil salinity, Bot. Gaz., 150, 346–350, 1989.
Yuan, J., Ding, W., Liu, D., Kang, H., Freeman, C., Xiang, J., and Lin, Y.: Exotic Spartina alterniflora invasion alters ecosystem-atmosphere exchange of CH4 and N2O and carbon sequestration in a coastal salt marsh in China, Global Change Biol., 21, 1567–1580, https://doi.org/10.1111/gcb.12797, 2015.