the Creative Commons Attribution 4.0 License.
the Creative Commons Attribution 4.0 License.
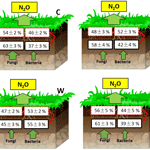
Fungi regulate the response of the N2O production process to warming and grazing in a Tibetan grassland
Lei Zhong
Shiping Wang
Xingliang Xu
Yanfen Wang
Yichao Rui
Xiaoqi Zhou
Qinhua Shen
Jinzhi Wang
Lili Jiang
Caiyun Luo
Tianbao Gu
Wenchao Ma
Guanyi Chen
Lack of understanding of the effects of warming and winter grazing on soil fungal contribution to the nitrous oxide (N2O) production process has limited our ability to predict N2O fluxes under changes in climate and land use management, because soil fungi play an important role in driving terrestrial N cycling. A controlled warming and winter grazing experiment that included control (C), winter grazing (G), warming (W) and warming with winter grazing (WG) was conducted to investigate the effects of warming and winter grazing on soil N2O production potential in an alpine meadow on the Tibetan Plateau. Our results showed that soil bacteria and fungi contributed 46 ± 2 % and 54 ± 2 % to nitrification, and 37 ± 3 % and 63 ± 3 % to denitrification in the control treatment, respectively. We conclude that soil fungi could be the main source of N2O production potential for the Tibetan alpine grasslands. In our results, neither warming nor winter grazing affected the activity of enzymes responsible for overall nitrification and denitrification. However, warming significantly increased the enzyme activity of bacterial nitrification and potential of N2O production from denitrification to 53 ± 2 % and 55 ± 3 %, respectively, but decreased them to 47 ± 2 % and 45 ± 3 %, respectively. Winter grazing had no such effects. Warming and winter grazing may not affect the soil N2O production potential, but climate warming can alter biotic pathways responsible for N2O production process. These findings confirm the importance of soil fungi in the soil N2O production process and how they respond to environmental and land use changes in alpine meadow ecosystems. Therefore, our results provide some new insights into ecological controls on the N2O production process and contribute to the development of an ecosystem nitrogen cycle model.
- Article
(4055 KB) - Full-text XML
- BibTeX
- EndNote
N2O emissions from soil contribute to climate warming as N2O is a potent greenhouse gas (IPCC, 2015); it is mainly produced in soils through microbial nitrification and denitrification (Zumft, 1997). Clarifying nitrification and denitrification processes and their controlling factors will be beneficial for understanding the N cycle in terrestrial ecosystems. Previous studies are mainly focused on bacterial nitrification and denitrification (Hayatsu et al., 2008; Klotz and Stein, 2008) because the conventional N cycle is thought to be controlled primarily by bacteria. However, recent studies using novel molecular techniques have shown that soil fungi are important players in terrestrial N cycling, including N2O production and nitrification–denitrification in drylands or soils with high organic carbon (C) and N (Chen et al., 2015; Huang et al., 2017; Laughlin and Stevens, 2002; Marusenko et al., 2013; Zhong et al., 2018).
The Tibetan grasslands occupy approximately 40 % of the Tibetan Plateau, which represents 0.7–1.0 % of total global N storage (Tian et al., 2006) and is required for sufficient forage production (Zheng et al., 2000). These grasslands represent one of the most vulnerable regions in the world to climate change and anthropogenic perturbation (Thompson et al., 1993; Thompson, 2000; Wang and French, 1994). A much greater than average increase in the surface temperature has been predicted to occur in this region in the future (Giorgi et al., 2001) and have profound impacts on soil N cycling in alpine grasslands. Additionally, the grasslands of the Tibetan Plateau are generally divided into two grazing seasons, i.e., summer grazing from June to September and winter grazing from October to May (Cui et al., 2014), which host about 13.3 million domestic yaks and 50 million sheep, with dramatically increasing numbers in the future (Yao et al., 2006). Grazing strongly affects soil N cycling, as well as plant and microbial diversity (Hillebrand, 2008) and the stability of ecosystems (Klein et al., 2004). Previous studies have demonstrated losses of N caused by warming (Klein et al., 2004, 2007) and that overgrazing (Zhou et al., 2005) leads to degradation in alpine grasslands. The effects of climate warming and grazing on the aboveground vegetation, soil physicochemical properties, litter mass loss, bacterial communities and N2O fluxes of Tibetan alpine grasslands have been extensively investigated (Hu et al., 2010; Li et al., 2016; Luo et al., 2010; Rui et al., 2012; Wang et al., 2012; Zhu et al., 2015); however, most of these studies were focused on the effect of summer grazing; little is shown on the effect of winter grazing on them (Zhu et al., 2015; Che et al., 2018). On the other hand, many studies of Tibetan alpine grasslands are mainly focused on bacterial nitrifiers and denitrifiers or their activities, taking these to be the key factors in N2O emission in alpine grasslands. However, while many studies have explored N mineralization, nitrification and even denitrification as well as bacterial nitrifiers and denitrifiers for better understanding of N2O emission and ecosystem functioning (Yang et al., 2013; Yue et al., 2015), few studies have been conducted to distinguish whether bacteria or fungi dominate in N2O emission and N cycling (Kato et al., 2013), especially under warming and grazing conditions.
Since optimum environments for fungi and bacteria are different, they may respond differently to environmental changes. Fungi prefer a lower temperature (Pietikäinen et al., 2005), higher organic C ∕ N (Chen et al., 2015) and a more arid soil environment (Marusenko et al., 2013) compared to bacteria. Climate warming and grazing can change vegetation cover, soil water and energy balance, alter the quantity and quality of soil organic matter and mineral N content (Saggar et al., 2004), and thus affect N2O production (Shi et al., 2017). However, it remains unknown how bacteria and fungi respond to concurrent warming and grazing and contribute to N2O production in alpine grasslands.
To clarify whether fungi played the main role in the N2O production process and its response to warming and winter grazing in alpine grasslands, we used a warming and grazing experiment over 10 years in an alpine meadow on the Tibetan Plateau. We measured the gene abundance of soil bacterial and fungal communities using quantitative PCR, and the potential of N2O emission from bacterial and fungal nitrification and denitrification through an incubation experiment to assess the contribution of N2O production potential from bacteria and fungi. We aimed to test the following hypotheses: (1) soil fungi were the main contributors to N2O production because of the low soil temperature and high organic C and N in the alpine grasslands, and (2) although N2O emission was not affected by warming and winter grazing at our site (Zhu et al., 2015), the biotic pathways responsible for N2O would be changed due to the distinctly preferred soil environments of bacterial and fungal communities.
2.1 Site and sampling
Details of the experimental site and design of the warming and grazing were described by Wang et al. (2012). The experiment was conducted in an alpine grassland (37∘37′ N, 101∘12′ E; 3250 m elevation) at the Haibei Alpine Meadow Ecosystem Research Station of the Chinese Academy of Sciences. Over the past 25 years, the mean annual temperature was −2 ∘C, and the mean annual precipitation was 500 mm. In the soil sampling year and month of August 2015, mean temperature was 0 and 9.7 ∘C, respectively; total rainfall was 327.2 and 46.6 mm, respectively. Over 80 % of total rainfall falls during the summer monsoon season (Luo et al., 2010; Zhao and Zhou, 1999). The soil was classified as Gelic Cambisols (WRB, 1998). The plant community at the experimental site is dominated by Kobresia humilis, Festuca ovina, Elymus nutans, Poa pratensis, Carex scabrirostris, Gentiana straminea, Gentiana farreri, Blysmus sinocompressus, Potentilla nivea and Dasiphora fruticosa (Luo et al., 2010).
A two-way factorial design (warming and grazing) was used with four replicates of each of four treatments (Wang et al., 2012), beginning in May 2006, namely no warming with no grazing (C), no warming with winter grazing (G), warming with no winter grazing (W) and warming with winter grazing (WG). In total, 16 plots of 3 m diameter were fully randomized throughout the study site.
For warming treatments, the design of the controlled warming (i.e., a free-air temperature enhancement (FATE) system with infrared heaters) with a grazing experiment was described previously by Kimball et al. (2008) and Wang et al. (2012). Free-air temperature enhancement using infrared heating was set up to create a warming treatment since May 2006 (Luo et al., 2010). The differences in canopy temperature at set points between heated plots and the corresponding reference plots were 1.2 ∘C during the daytime and 1.7 ∘C at night in summer. During winter, from October to April, the power output of the heaters was manually set at 1500 W per plot to make sure the increased soil temperature was the same as in summer, as some infrared thermometers were not working.
For grazing treatments, summer grazing treatments were used to explore the effects of warming and grazing on ecosystem during the growing season from 2006 to 2010 (Luo et al., 2010; Hu et al., 2010; Wang et al., 2012). Considering strong disturbance, grazing was stopped during 2011–2015; summer grazing was replaced by cutting and removing about 50 % of the litter biomass in October and the following March each year to simulate winter grazing. Given the importance of winter grazing, winter grazing during the non-growing seasons was further investigated (Zhu et al., 2015; Che et al., 2018). Alpine meadows in the region can be divided into two grazing seasons (i.e., warm-season grazing from June to September and cold-season grazing from October to May) (Cui et al., 2015). Before the experiment was conducted, we had examined how clipping simulated the effects of actual grazing before we established four replicated “actual grazing treatments” compared with the “simulated grazing treatments”. The soils and plants all showed no difference between simulated grazing and actual grazing treatments (Klein et al., 2004, 2007), because the soil is frozen in winter, meaning that the effect of selective feeding and trampling by sheep would be limited, so the effect of cutting in winter was similar to winter grazing (Zhu et al., 2015).
2.2 Soil sampling
Five soil cores (5 cm in diameter) were randomly collected within each plot on 15 August 2015 at a depth of 0–20 cm (including the organic layer) and then mixed to form a composite sample. All soil samples were transported to the laboratory and sieved through a 2 mm mesh before being stored at −20 or 4 ∘C for further molecular analyses.
2.3 Soil properties and gene abundance of bacteria and fungi analysis
Soil moisture content was measured by drying at 105 ∘C for 24 h. For soil mineral N (–N and –N) analyses, 10 g of soil (field-moist) were shaken for 1 h with 50 mL of 1 M KCl and filtered through filter paper, and determined the –N and –N concentrations by a Skalar flow analyzer (Skalar Analytical, Breda, the Netherlands). Total C and N contents were measured by using combustion elemental analyzers (PerkinElmer, EA2400, USA).
Soil DNA was extracted from 0.5 g of frozen soil using a FastDNA™ Kit for Soil (QBIOgene) based on the instructions and stored at −20 ∘C. Total bacteria and fungi copies were quantified by real-time PCR using an iCycler thermal cycler equipped with an optical module (Bio-Rad, USA)
The real-time PCR mixture contained 5 ng of soil DNA, 2 pmol of primers and a 10× iQ SYBR Green super mix (Bio-Rad) in a 20 µL reaction volume. The primers for bacteria were 341F 5′-CCTACGGGAGGCAGCAG-3′ and 534R5′-ATTACCGCGGCTGCTGGCA-3′ (Muyzer et al., 1993). The thermal cycle conditions were 10 min at 95 ∘C; 35 cycles of PCR were then performed in the iCycler iQ Real-Time PCR Detection System (BIORAD) as follows: 20 s at 95 ∘C, 15 s at 55 ∘C and 30 s at 72 ∘C. A final 5 min extension step completed the protocol. The primers for fungi were FU18S1 5′-GGAAACTCACCAGGTCCAGA-3′ derived from Nu-SSU-1196 and Nu-SSU-1536 5′-ATTGCAATGCYCTATCCCCA-3′ (Borneman and Hartin, 2000), and the thermal cycle conditions were one step of 10 min at 95 ∘C. Then 40 cycles of PCR were performed as follows: 20 s at 95 ∘C, 30 s at 62 ∘C and 30 s at 72 ∘C. A final 5 min extension step completed the protocol.
2.4 Total fungal and bacterial nitrification enzyme activity, and total fungal and bacterial potential of N2O production from denitrification analysis
Fungal (FNEA), bacterial (BNEA) and total nitrification enzyme activity (TNEA) was determined following the protocol described in Dassonville et al. (2011). Briefly, moist field soil equivalent to 12 g of dry soil was weighed into 240 mL specimen bottles (LabServ); 12 mL of NH4–N solution (50 µg N–(NH4)2SO4 g−1 dry soil) and distilled water were added to achieve a 96 mL total liquid volume, and the slurry was incubated at 28 ∘C for 10 h with constant agitation (180 rpm) in an orbital shaker (Lab-Line 3527; Boston, MA, USA) to mix slurry well and provide an aerobic environment. Three treatments were imposed: (i) cycloheximide (C15H23NO4, a fungicide) at 1.5 mg g−1 in solution was used to inhibit the nitrification activity from soil fungi, (ii) streptomycin sulfate (C42H84N14O36S3, a bactericide) at 3.0 mg g−1 in solution was used to inhibit the nitrification activity from soil bacteria (Castaldi and Smith, 1998; Laughlin et al., 2009) and (III) a no-inhibitor control was used to show the total nitrification activity. During incubation, 10 mL of the soil slurry was sampled with a syringe at 2, 4, 6, 8 and 10 h, and then filtered through Whatman No. 42 ashless filter paper. Filtered samples were stored at −20 ∘C until analysis for concentration on a LACHAT Quickchem Automated Ion Analyzer (Foss 5027 Sampler, TECATOR, Hillerød, Denmark). Linear regression between the production rate and time was observed, and the rates of nitrification enzyme activity were determined from the slope of this linear regression. The nitrification enzyme activity of soil fungi was estimated by the difference between rates of nitrification enzyme activity under treatment (III) and treatment (I); the nitrification enzyme activity of soil bacteria was estimated by the difference between rates of nitrification enzyme activity under treatment (III) and treatment (II). The total nitrification enzyme activity was from treatment (III).
Fungal (FDEA), bacterial (BDEA) and total potentials of N2O production (TDEA) from denitrification were measured in fresh soil from each plot following the protocol described in Patra et al. (2006) and Marusenko et al. (2013). Three sub-samples (equivalent to 12 g dry soil) from each soil sample were placed into 240 mL plasma flasks, and 7 mL of a solution containing KNO3 (50 µg N g−1 dry soil), glucose (0.5 mg C g−1 dry soil) and glutamic acid (0.5 mg C g−1 dry soil) were added. Additional distilled water was provided to achieve 100 % water-holding capacity and optimal conditions for denitrification. Three treatments were imposed: (I) cycloheximide (C15H23NO4; a fungicide) at 1.5 mg g−1 in solution was used to inhibit the fungal potential of N2O production from denitrification, (II) streptomycin sulfate (C42H84N14O36S3; a bactericide) at 3.0 mg g−1 in solution was used to inhibit the bacterial potential of N2O production from denitrification (Castaldi and Smith, 1998; Laughlin and Stevens, 2002), and (III) a no-inhibitor control was used to show the total potential of N2O production from denitrification. The headspace air of the specimen bottles was replaced with N2 to provide anaerobic conditions. Specimen bottles were then sealed with a lid containing a rubber septum for gas sample collection. Specimen bottles with the soil slurry were then incubated at 28 ∘C for 48 h with constant agitation (180 rpm) in an orbital shaker (Lab-Line 3527; Boston, MA, USA). During incubation, 12 mL gas samples were taken at 0, 24 and 48 h with syringes and injected into pre-evacuated 6 mL glass vials. The N2O concentration of the gas samples was analyzed via gas chromatography. The potential of N2O production from denitrification was calculated from the slope of the regression using values for 0, 24 and 48 h of incubation. The fungal potential of N2O production from denitrification was estimated by the difference between potential production under treatment (III) and treatment (I). The bacterial potential of N2O production from denitrification was estimated by the difference between rates of denitrification enzyme activity under treatment (III) and treatment (II). Total denitrification enzyme activity was from treatment (III).
The contribution of bacteria and fungi to total nitrification enzyme activity was calculated by the ratio of BNEA or FNEA to BNEA + FNEA; the contribution of bacteria and fungi to the total potential of N2O production from denitrification was calculated by the ratio of BDEA or FDEA to BDEA + FDEA.
2.5 Statistical analysis
For the controlled experiment, the statistical significance of the effects of warming, grazing and their interaction on plant biomass, soil properties, microbial functional genes, and fungal and bacterial nitrification enzyme activity and potential of N2O production from denitrification were tested by two-way ANOVA in the PROC GLM procedure of SAS (version 9, SAS Institute, Cary, NC, USA).
3.1 Plant biomass and soil properties
The average plant standing biomass was 343, 345, 301 and 362 g dry matter m−2 in the control, G, W and WG treatments measured at the day of soil sampling, respectively. Grazing and warming had no effect on plant biomass (Fig. 1a, Table 1).
Soil temperature varied from 11.8 to 14.0 ∘C. Grazing (P= 0.05) and warming (P < 0.01) increased soil temperature (Fig. 1b, Table 1). The average soil moisture varied from 26 % to 34 % (w∕w). Grazing had no effect on soil moisture, which was lower in warming plots (P < 0.01) (Fig. 1c, Table 1). There was an interactive effect between grazing and warming on soil temperature (P < 0.01).
Soil total C (TC) was not affected by grazing (P= 0.13) or warming (P= 0.12) alone, but there was a marginal interaction between grazing and warming on TC (P= 0.07) (Fig. 2a, Table 1). Similar to TC, soil total N (TN) also showed no response to grazing or warming (Fig. 2b, Table 1). Soil –N content was lower in warming treatments than in no-warming treatments (P= 0.05) (Fig. 2c, Table 1). Greater soil NO3–N content occurred under the warming treatments (P= 0.05) than under the no-warming treatments (Fig. 2d, Table 1).
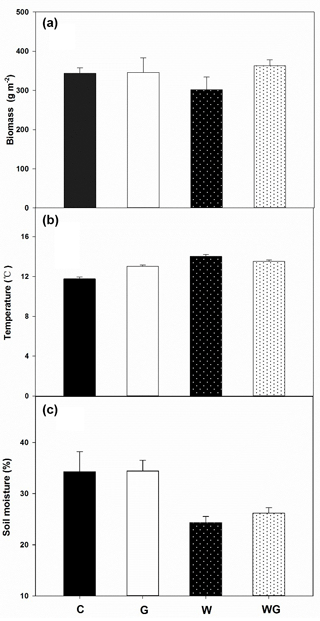
Figure 1Plant biomass (a) soil temperature (b) and soil moisture content (c) in an alpine meadow. C (black bar), control treatment; G (white bar), winter grazing treatment; W (white-dotted bar), warming treatment; WG (black-dotted bar), warming combined with the winter grazing treatment. Values are means ±1 s.e.m. (n= 4).
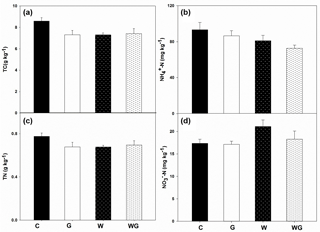
Figure 2Soil total carbon (TC) (a), soil total nitrogen (TN) (b), soil –N (c) and –N (d) content in an alpine meadow. C (black bar), control treatment; G (white bar), winter grazing treatment; W (white-dotted bar), warming treatment; WG (black-dotted bar), warming combined with the winter grazing treatment. Values are means ±1 s.e.m. (n= 4).
3.2 Microbial functional genes
Bacterial gene abundance varied from 4.71×109 to 5.93×109 copies g−1 dry soil, which was much higher than fungal gene abundance (Fig. 3). Warming and grazing both increased the bacterial gene abundance in soil (P < 0.01), but there was no interaction effect between them on bacterial gene abundance (Table 1). By comparison, fungal gene abundance showed no difference across all treatments.
3.3 Nitrification enzyme activity and potential of N2O production from denitrification of bacteria and fungi
TNEA varied from 1.07 to 1.64 µg N g−1 h−1 in all treatments. BNEA ranged from 0.43 to 0.64 µg N g−1 h−1, which was lower than the FNEA in soil (0.59–0.66 µg N g−1 h−1) (P= 0.01) (Fig. 4a–c). FNEA was lower under warming treatments than under the no-warming treatments (P= 0.05) (Table 1).
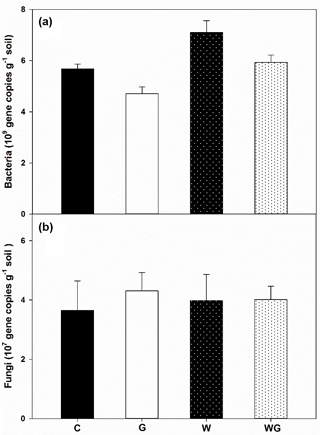
Figure 3Abundance of bacteria (a) and fungi (b) in an alpine meadow; C (black bar), control treatment; G (white bar), winter grazing treatment; W (white-dotted bar), warming treatment; WG (black-dotted bar), warming combined with the winter grazing treatment. Values are means ±1 s.e.m. (n= 4).
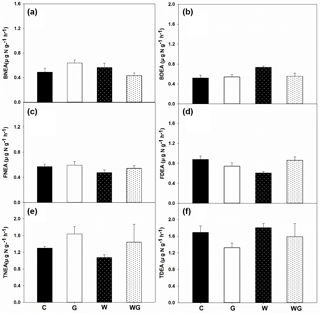
Figure 4Bacterial nitrification enzyme activity (BNEA) (a), fungal nitrification enzyme activity (FNEA) (b), total nitrification enzyme activity (TNEA) (c); bacterial potential of N2O production from denitrification (BDEA) (d), fungal potential of N2O production from denitrification (FDEA) (e) and total potential of N2O production from denitrification (TDEA) (f) in an alpine meadow. C (black bar), control treatment; G (white bar), winter grazing treatment; W (white-dotted bar), warming treatment; WG (black-dotted bar), warming combined with the winter grazing treatment. Values are means ±1 s.e.m. (n= 4).
TDEA was between 1.32 and 1.80 µg N g−1 h−1. FDEA was clearly the dominant process for TDEA (Fig. 4d–f), because it was higher than BDEA for all treatments except warming. Warming increased BDEA (P= 0.04). Warming and grazing had a significant interaction effect on FDEA (P < 0.01) (Table 1).
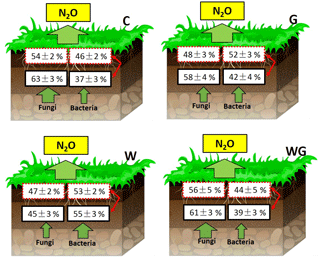
Figure 5Contribution of bacteria and fungi to total nitrification enzyme activity (box with the red and dashed line) and total potential of N2O production from denitrification (box with the black and solid line) in an alpine meadow. C (black bar), control treatment; G (white bar), winter grazing treatment; W (white-dotted bar), warming treatment; WG (black-dotted bar), warming combined with the winter grazing treatment. Values are means ±1 s.e.m. (n= 4).
3.4 The contribution of bacteria and fungi to potential N2O emissions
The contribution of FNEA to TNEA varied from 47 ± 2 % to 56±5 %, and the contribution of FDEA to TDEA varied from 45 ± 3 % to 63 ± 3 % (Fig. 5). Warming significantly decreased the contribution of FNEA and FDEA to TNEA and TDEA in soils (FNEA: P= 0.02; FDEA: P= 0.04).
N2O is mainly produced from microbial nitrification and denitrification processes, but the contribution of bacteria and fungi to nitrification and denitrification processes is still unclear. In our results, fungi contributed 54 % and 63 % of the TNEA and TDEA, respectively, in control treatments of the alpine grassland studied. Our result of the fungal contribution to potential of N2O production is much lower than Laughlin and Stevens (2002) and Zhong et al. (2018), who reported 89 % and 86 % fungal contributions from temperate grasslands, but is higher than the 40–51 % fungal contribution observed across different ecosystems by Chen et al. (2014). Kato et al. (2013) also showed that N2O emissions from FDEA were higher than from BDEA in alpine meadows, reinforcing the important role fungi play in the denitrification process. Our findings support our first hypothesis and further proved that both nitrification and denitrification were largely driven by fungal communities in alpine meadow grasslands. A possible explanation is that fungi prefer the arid, high organic substrate and low-temperature environment (Pietikäinen et al., 2005; Chen et al., 2015; Marusenko et al., 2013). In alpine grasslands, the mean annual temperature is 0 ∘C; even during the sampling day, the mean temperature was only 11 ∘C. The cold environment could cause higher activity in fungi than in bacteria. Moreover, the cold environment decreases the rate of mineralization, leading to greater organic C and N accumulation (Ineson et al., 1998; Schmidt et al., 2004). In our study, soil TC and TN concentrations were 72–86 and 6–7 g kg−1, respectively (Fig. 2a and b), much higher than in temperate grasslands and farmland, providing a favorable environment for fungi (Bai et al., 2010). These are the main reasons why soil fungi played the main role in the N2O production process in the Tibetan alpine grasslands.
Our methodology did not exclude a role for archaea in nitrification and denitrification. Previous studies on grasslands only focused on fungal and bacterial process because archaeal-specific inhibitors have not yet been identified for N cycling processes. However, archaea are widespread in soil, and are involved in nitrification denitrification (Cabello et al., 2004); e.g., archaeal ammonia oxidizers are global (Leininger et al., 2006). In our study, we also found that the TNEA was higher than the sum of NEA from bacteria and fungi, while TDEA was higher than DEA from bacteria and fungi (Fig. 4), which showed that archaea also played a role in the N2O production process in our site. The development of inhibitor-based approaches may help to show how archaea respond to environmental change (Marusenko et al., 2013).
Our results supported the second hypothesis that although warming did not change the total N2O production potential on the Qinghai–Tibetan Plateau, the biotic pathways responsible for N2O had been changed, as bacterial contributions to TNEA and TDEA were all higher than fungal, which suggested higher bacterial N2O production potential under warming treatment (Fig. 4, Table 1). The increase in bacterial N2O production potential, coupled with a decrease in fungal N2O production potential, could be the main reasons why the total N2O production potential showed no difference between control and warming treatments. The field data of N2O emission in our site measured in the years of 2011–2012 also showed no effect of warming on N2O emission (Zhu et al., 2015). Our results reinforced this and suggested that bacterial nitrification and the denitrification process alone are unable to accurately describe the response of N2O to warming.
It is these two reasons that lead to the changes in fungal and bacterial pathways for the N2O production process by warming. Firstly, warming significantly increased the soil temperature (Fig. 1b, Table 1); the increased soil temperature directly reduced fungal activity but increased bacterial activity, because fungi prefer the cold environment compared with bacteria (Pietikäinen et al., 2005). Secondly, fungi prefer a higher organic C ∕ N environment, while bacteria prefer a higher inorganic C ∕ N environment (Chen et al., 2015). In our site, although the soil –N concentration did not change with warming, soil –N concentration that was significantly increased showed that the soil inorganic N was increased (Fig. 2a and b, Table 1); on the other hand, the soil dissolved organic nitrogen was significantly decreased from 48 to 41 mg kg−1 (P < 0.04), and the soil labile C and N were also found to be significantly decreased by warming (Rui et al., 2012); this showed that the soil organic C and N were decreased in our site. Therefore, warming indirectly reduced fungal activity but increased bacterial activity through increased soil inorganic N and decreased soil organic N in our site. In our site, the FNEA and FDEA were reduced by 16 % and 30 %, respectively, but the BNEA and BDEA were increased by 15 % and 41 %, respectively, by warming. All these changes resulted in fungi contributing less to nitrification and denitrification than bacteria (Fig. 5). Although the gene abundance of fungi was not changed by warming, which showed inconsistencies with the changes in FNEA and FDEA, these inconsistencies might be explained by the fungal gene abundance not likely providing information on real-time process rates since such rates are dependent on environmental conditions: fluctuations in environmental conditions can cause rapid changes in real-time process rates but not necessarily affect gene abundance (Zhong et al., 2014). In summary, it indicates that the soil microbial process was altered by warming, even though the total potential of N2O production did not change, with a shift in dominance from fungi to bacteria in the N2O production process after 10 years of warming.
Numerous studies have demonstrated that grazing can impact microbial processes and induce the loss of N by (1) altering the substrate concentration for N2O production and reduction in soil through the deposition of dung and urine (Saggar et al., 2004); (2) reducing vegetation cover due to changes in soil water content and energy balance (Leriche et al., 2001); and (3) increasing soil compaction and reducing soil aeration through animal tramping (Houlbrooke et al., 2008). However, most of these were focused on grazing in the growing season, and few were focused on the effect of winter grazing on the N cycle process. In this study fungal and bacterial potential of N2O production from nitrification and denitrification all showed little response to winter grazing (Fig. 4, Table 1). A possible explanation is that neither soil moisture, nor plant biomass nor organic/inorganic C ∕ N content was affected by winter grazing (Figs. 1–2, Table 1). Additionally, the soil was frozen in winter, so that the effect of selective feeding and trampling could be limited by grazing sheep (Zhu et al., 2015; Krümmelbein et al., 2009; Steffens et al., 2008). As a result, the same soil environmental conditions for both winter grazing and control had no effect on soil fungi and bacteria, and thus on fungal and bacterial nitrification and denitrification. Moreover, the field data of N2O emission in the years of 2011–2012 also support the results and suggest that replacing summer grazing by winter grazing could cause the soil N cycle process to become stable (Zhu et al., 2015).
Overall, we conclude that fungi played the dominant role in the N2O production process in alpine meadows. Previous studies had proved that the climate warming did not affect the N2O production in our site (Zhu et al., 2015), but we found that warming could alter biotic pathways responsible for the N2O production process on the Tibetan Plateau. Our study exhibited the effects of a decade of the simulation experiment; however, a thorough understanding of the long-term impact of warming and grazing on soil fungal nitrification and denitrification from alpine meadow grasslands requires further investigation for a multi-decade period.
From this study, due to the different adaptation strategies of fungi and bacteria, and their different nutrition requirements, future changes in climate and soil resources are likely to affect biogeochemistry in a way not currently accounted for in ecosystem models that assume N transformations are controlled only by bacteria. Accurate predictions for N2O production and N loss due to environmental change and land use will benefit from the inclusion of fungi as key mediators of ecological processes in grasslands.
Data are available from the corresponding author upon request.
GYC, WCM, and LZ conceived the idea; YFW, SPW, and XLX designed the experiment; QHS, JZW, and LLJ performed soil sampling, plant biomass, and measurements; LZ, RYC, and XQZ analyzed data and prepared the manuscript, and all authors contributed to the writing.
The authors declare that they have no conflict of interest.
This work was supported by the National Key R&D Program of China
(no. 2016YFC0501802), the National Natural Science Foundation of China
(nos. 41601245 and 31672474), the Foundation of Committee on Science and Technology of Tianjin
(no. 16YFXTSF00500), and Strategic Priority Research Program B of the Chinese
Academy of Sciences (no. XDB15010201). We also thank Ri Weal for her
assistance in improving the use of English in the manuscript.
Edited by: Yakov Kuzyakov
Reviewed by:
Xiaojuan Feng and five anonymous referees
Bai, Y., Jianguo, W. U., Clark, C. M., Naeem, S., Pan, Q., Huang, J., Zhang, L., and Han, X.: Tradeoffs and thresholds in the effects of nitrogen addition on biodiversity and ecosystem functioning: evidence from inner Mongolia Grasslands, Glob. Change Biol., 16, 358–372, https://doi.org/10.1111/j.1365-2486.2009.01950.x, 2010.
Borneman, J. and Hartin, R. J.: PCR primers that amplify fungal rRNA genes from environmental samples, Appl. Environ. Microb., 66, 4356–4360, https://doi.org/10.1128/AEM.66.10.4356-4360, 2000.
Cabello, P., Roldán, M. D., and Moreno-Vivián, C.: Nitrate reduction and the nitrogen cycle in archaea, Microbiology, 150, 3527–3546, 2004.
Castaldi, S. and Smith, K. A.: Effect of cycloheximide on N2O and NO production in a forest and an agricultural soil, Biol. Fert. Soils, 27, 27–34, 1998.
Che, R., Deng, Y., Wang, W., Rui, Y., Zhang, J., Tahmasbian, I., Tang, L., Wang, S., Wang Y., Xu, Z., and Cui, X.: Long-term warming rather than grazing significantly changed total and active soil procaryotic community structures, Geoderma, 316, 1–10, 2018.
Chen, H., Mothapo, N. V., and Shi, W.: The significant contribution of fungi to soil N2O production across diverse ecosystems, Appl. Soil Ecol., 73, 70–77, 2014.
Chen, H., Mothapo, N. V., and Shi, W.: Fungal and bacterial N2O production regulated by soil amendments of simple and complex substrates, Soil Biol. Biochem., 84, 116–126, https://doi.org/10.1016/j.soilbio.2015.02.018, 2015.
Cui, S., Zhu, X., Wang, S., Zhang, Z., Xu, B., Luo, C., Zhao, L., and Zhao, X.: Effects of seasonal grazing on soil respiration in alpine meadow on the Tibetan plateau, Soil Use Manage., 30, 435–443, 2015.
Dassonville, N., Guillaumaud, N., Piola, F., Meerts, P., and Poly, F.: Niche construction by the invasive Asian knotweeds (species complex Fallopia): Impact on activity, abundance and community structure of denitrifiers and nitrifiers, Biol. Invasions, 13, 1115–1133, 2011.
Giorgi, F., Whetton, P. H., Jones, R. G., Christensen, J. H., Mearns, L. O., Hewitson, B., Vonstorch, H., Francisco, R., and Jack, C.: Emerging patterns of simulated regional climatic changes for the 21st century due to anthropogenic forcings, Geophys. Res. Lett., 28, 3317–3320, 2001.
Hayatsu, M., Tago, K., and Saito, M.: Various players in the nitrogen cycle: Diversity and functions of the microorganisms involved in nitrification and denitrification, J. Soil Sci. Plant Nut., 54, 33–45, https://doi.org/10.1111/j.1747-0765.2007.00195.x, 2008.
Hillebrand, H.: Grazing regulates the spatial variability of periphyton biomass, Ecology, 89, 165–173, 2008.
Houlbrooke, D. J., Littlejohn, R. P., Morton, J. D., and Paton, R. J.: Effect of irrigation and grazing animals on soil quality measurements in the North Otago Rolling Downlands of New Zealand, Soil Use Manage., 24, 416–423, 2008.
Hu, Y., Chang, X., Lin, X., Wang, Y., Wang, S., Duan, J., Zhang, Z., Yang, X., Luo, C., and Xu, G.: Effects of warming and grazing on N2O fluxes in an alpine meadow ecosystem on the Tibetan plateau, Soil Biol. Biochem., 42, 944–952, 2010.
Huang, Y., Xiao, X., and Long, X.: Fungal denitrification contributes significantly to N2O production in a highly acidic tea soil, J. Soil Sediment., 17, 1599–1606, https://doi.org/10.1007/s11368-017-1655-y, 2017.
Intergovernmental Panel on Climate Change (IPCC): Climate change 2014: Mitigation of climate change, Cambridge University Press, 2015.
Ineson, P., Benham, D. G., Poskitt, J., Harrison, A. F., Taylor, K., and Woods, C.: Effects of climate change on nitrogen dynamics in upland soils. 2. A soil warming study, Glob. Change Biol., 4, 143–152, 1998.
Kato, T., Toyoda, S., Yoshida, N., Tang, Y., and Wada, E.: Isotopomer and isotopologue signatures of N2O produced in alpine ecosystems on the Qinghai-Tibetan Plateau, Rapid Commun. Mass Sp., 27, 1517–1526, 2013.
Kimball, B. A., Conley, M. M., Wang, S. P., Lin, X. W., Luo, C. Y., Morgan, J., and Smith, D.: Infrared heater arrays for warming ecosystem field plots, Glob. Change Biol., 14, 309–320, 2008.
Klein, J. A., Harte, J., and Zhao, X. Q.: Experimental warming causes large and rapid species loss, dampened by simulated grazing, on the Tibetan Plateau, Ecol. Lett., 7, 1170–1179, 2004.
Klein, J. A., Harte, J., and Zhao, X. Q.: Experimental warming, not grazing, decreases rangeland quality on the Tibetan Plateau, Ecol. Appl., 17, 541, https://doi.org/10.1890/05-0685, 2007.
Klotz, M. G. and Stein, L. Y.: Nitrifier genomics and evolution of the nitrogen cycle, Fems Microbiol. Lett., 278, 146–156, https://doi.org/10.1111/j.1574-6968.2007.00970.x, 2008.
Krümmelbein, J., Peth, S., Zhao, Y., and Horn, R.: Grazing-induced alterations of soil hydraulic properties and functions in Inner Mongolia, PR China, J. Plant Nutr. Soil Sc., 172, 769–776, 2009.
Laughlin, R. J. and Stevens, R. J.: Evidence for fungal dominance of denitrification and codenitrification in a grassland soil, Soil Sci. Soc. Am. J., 66, 1540–1548, 2002.
Laughlin, R. J., Rutting, T., Mueller, C., Watson, C. J., and Stevens, R. J.: Effect of acetate on soil respiration, N2O emissions and gross N transformations related to fungi and bacteria in a grassland soil, Appl. Soil Ecol., 42, 25–30, https://doi.org/10.1016/j.apsoil.2009.01.004, 2009.
Leininger, S., Urich, T., Schloter, M., Schwark, L., Qi, J., Nicol, G. W., Prosser, J. I., Schuster, S. C., and Schleper, C.: Archaea predominate among ammoniaoxidizing prokaryotes in soils, Nature, 442, 806–809, 2006.
Leriche, H., Leroux, X., Gignoux, J., Tuzet, A., Fritz, H., Abbadie, L., and Loreau, M.: Which functional processes control the short-term effect of grazing on net primary production in grasslands?, Oecologia, 129, 114–124, 2001.
Li, Y., Lin, Q., Wang, S., Li, X., Liu, W., Luo, C., Zhang, Z., Zhu, X., Jiang, L., and Li, X.: Soil bacterial community responses to warming and grazing in a Tibetan alpine meadow, Fems Microbiol. Ecol., 92, fiv152, https://doi.org/10.1093/femsec/fiv152, 2016.
Luo, C., Xu, G., Chao, Z., Wang, S., Lin, X., Hu, Y., Zhang, Z., Duan, J., Chang, X., and Su, A.: Effect of warming and grazing on litter mass loss and temperature sensitivity of litter and dung mass loss on the Tibetan plateau, Glob. Change Biol., 16, 1606–1617, 2010.
Marusenko, Y., Huber, D. P., and Hall, S. J.: Fungi mediate nitrous oxide production but not ammonia oxidation in aridland soils of the southwestern US, Soil Biol. Biochem., 63, 24–36, 2013.
Muyzer, G., Waal, E. C. D., and Uitterlinden, A. G.: Profiling of complex microbial populations by denaturing gradient gel electrophoresis analysis of polymerase chain reaction-amplified genes coding for 16S rRNA, Appl. Environmen. Microbiol., 59, 695, 1993.
Patra, A. K., Abbadie, L., Clays-Josserand, A., Degrange, V., Grayston, S. J., Guillaumaud, N., Loiseau, P., Louault, F., Mahmood, S., and Nazaret, S.: Effects of management regime and plant species on the enzyme activity and genetic structure of N-fixing, denitrifying and nitrifying bacterial communities in grassland soils, Environ. Microbiol., 8, 1005–1016, 2006.
Pietikäinen, J., Pettersson, M., and Bååth, E.: Comparison of temperature effects on soil respiration and bacterial and fungal growth rates, Fems Microbiol. Ecol., 52, 49, https://doi.org/10.1016/j.femsec.2004.10.002, 2005.
Rui, Y., Wang, Y., Chen, C., Zhou, X., Wang, S., Xu, Z., Duan, J., Kang, X., Lu, S., and Luo, C.: Warming and grazing increase mineralization of organic P in an alpine meadow ecosystem of Qinghai-Tibet Plateau, China, Plant Soil, 357, 73–87, 2012.
Saggar, S., Bolan, N. S., Bhandral, R., Hedley, C. B., and Luo, J.: A review of emissions of methane, ammonia, and nitrous oxide from animal excreta deposition and farm effluent application in grazed pastures, New Zeal. J. Agr. Res., 47, 513–544, 2004.
Schmidt, I. K., Tietema, A., Williams, D., Gundersen, P., Beier, C., Emmett, B. A., and Estiarte, M.: Soil Solution Chemistry and Element Fluxes in Three European Heathlands and Their Responses to Warming and Drought, Ecosystems, 7, 638–649, 2004.
Shi, H., Hou, L., Yang, L., Wu, D., Zhang, L., and Li, L.: Effects of grazing on CO2, CH4, and N2O fluxes in three temperate steppe ecosystems, Ecosphere, 8, e01760, https://doi.org/10.1002/ecs2.1760, 2017.
Steffens, M., Kölbl, A., Totsche, K. U., and Kögel-Knabner, I.: Grazing effects on soil chemical and physical properties in a semiarid steppe of Inner Mongolia (PR China), Geoderma, 143, 63–72, 2008.
Thompson, L. G.: Ice core evidence for climate change in the Tropics: implications for our future, Quaternary Sci. Rev., 19, 19–35, 2000.
Thompson, L. G., Mosley-Thompson, E., Davis, M., Lin, P. N., Yao, T., Dyurgerov, M., and Dai, J.: “Recent warming”: ice core evidence from tropical ice cores with emphasis on Central Asia, Global Planet. Change, 7, 145–156, 1993.
Tian, H., Wang, S., Liu, J., Pan, S., Chen, H., Zhang, C., and Shi, X.: Patterns of soil nitrogen storage in China, Global Biogeochem. Cy., 20, 247–247, 2006.
Wang, B. and French, H. M.: Climate controls and high-altitude permafrost, qinghai-xizang (tibet) Plateau, China, Permafrost Periglac., 5, 87–100, 1994.
Wang, S., Duan, J., Xu, G., Wang, Y., Zhang, Z., Rui, Y., Luo, C., Xu, B., Zhu, X., and Chang, X.: Effects of warming and grazing on soil N availability, species composition, and ANPP in an alpine meadow, Ecology, 93, 2365–2376, 2012.
WRB: World Reference Base for Soil Resources, FAO/ISRIC/ISSS, Italy, 1998.
Yang, Y., Wu, L., Lin, Q., Yuan, M., Xu, D., Yu, H., Hu, Y., Duan, J., Li, X., and He, Z.: Responses of the functional structure of soil microbial community to livestock grazing in the Tibetan alpine grassland, Glob. Change Biol., 19, 637–648, 2013.
Yao, J., Yang, B., and Yan, P.: Analysis on habitat variance and behaviour of Bos gruiens in China, Acta Prataculturae Sinica, 15, 124–128, 2006 (in Chinese).
Yue, H., Wang, M., Wang, S., Gilbert, J. A., Sun, X., Wu, L., Lin, Q., Hu, Y., Li, X., and He, Z.: The microbe-mediated mechanisms affecting topsoil carbon stock in Tibetan grasslands, ISME J., 9, https://doi.org/10.1038/ismej.2015.19, 2015.
Zhao, X. Q. and Zhou, X. M.: Ecological Basis of Alpine Meadow Ecosystem Management in Tibet: Haibei Alpine Meadow Ecosystem Research Station, 28, 642–647, 1999.
Zheng, D., Zhang, Q., and Wu, S.: Mountain Geoecology and Sustainable Development of the Tibetan Plateau, Geoj. Lib., 57, available at: https://link.springer.com/book/10.1007/978-94-010-0965-2, 2000.
Zhong, L., Du, R., Ding, K., Kang, X., Li, F., Bowatte, S., Hoogendoorn, C., Wang, Y., Rui, Y., Jiang, L., and Wang S.: Effects of grazing on N2O production potential and abundance of nitrifying and denitrifying microbial communities in meadow-steppe grassland in northern China, Soil Biol. Biochem., 69, 1–10, https://doi.org/10.1016/j.soilbio.2013.10.028, 2014.
Zhong, L., Bowatte, S., Newton, P. C., Hoogendoorn, C. J., and Luo, D.: An increased ratio of fungi to bacteria indicates greater potential for N2O production in a grazed grassland exposed to elevated CO2, Agr. Ecol. Environ., 254, 111–116, 2018.
Zhou, H., Zhao, X., Tang, Y., Gu, S., and Zhou, L.: Alpine grassland degradation and its control in the source region of the Yangtze and Yellow Rivers, China, Grassl. Sci., 51, 191–203, 2005.
Zhu, X., Luo, C., Wang, S., Zhang, Z., Cui, S., Bao, X., Jiang, L., Li, Y., Li, X., and Wang, Q.: Effects of warming, grazing/cutting and nitrogen fertilization on greenhouse gas fluxes during growing seasons in an alpine meadow on the Tibetan Plateau, Agr. Forest Meteorol., 214, 506–514, 2015.
Zumft, W. G.: Cell biology and molecular basis of denitrification, Microbiol. Mol. Biol. R., 61, 533–616, 1997.