the Creative Commons Attribution 4.0 License.
the Creative Commons Attribution 4.0 License.
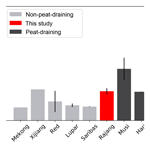
Impact of peatlands on carbon dioxide (CO2) emissions from the Rajang River and Estuary, Malaysia
Denise Müller-Dum
Thorsten Warneke
Tim Rixen
Moritz Müller
Antje Baum
Aliki Christodoulou
Joanne Oakes
Bradley D. Eyre
Justus Notholt
Tropical peat-draining rivers are known as potentially large sources of carbon dioxide (CO2) to the atmosphere due to the high loads of carbon they receive from surrounding soils. However, not many seasonally resolved data are available, limiting our understanding of these systems. We report the first measurements of carbon dioxide partial pressure (pCO2) in the Rajang River and Estuary, the longest river in Malaysia. The Rajang River catchment is characterized by extensive peat deposits found in the delta region, and by human impact such as logging, land use and river damming. pCO2 averaged 2540±189 µatm during the wet season and 2350±301 µatm during the dry season. Using three different parameterizations for the gas transfer velocity, calculated CO2 fluxes to the atmosphere were 1.5 (0.5–2.0) g C m−2 d−1 (mean, minimum – maximum) during the wet season and 1.7 (0.6–2.6) g C m−2 d−1 during the dry season. This is at the low end of reported values for Southeast Asian peat-draining rivers, but similar to values reported for Southeast Asian rivers that do not flow through peat deposits. In the Rajang River, peatlands probably do not contribute much to the CO2 flux due to the proximity of the peatlands to the coast, which limits the opportunity for degradation of organic C during transport. Thus, we suggest that peat coverage is, by itself, insufficient as the sole predictor of CO2 emissions from peat-draining rivers, and that other factors, like the spatial distribution of peat in the catchment and pH, also need to be considered.
- Article
(2422 KB) - Full-text XML
-
Supplement
(4207 KB) - BibTeX
- EndNote
Tropical rivers transport large amounts of terrestrially derived carbon to the ocean (Dai et al., 2012) and the atmosphere (Aufdenkampe et al., 2011; Raymond et al., 2013). It has been estimated that 78 % of riverine carbon dioxide (CO2) emissions occur in the tropics (Lauerwald et al., 2015). Tropical wetlands exert a particularly strong influence on the carbon budget of these rivers. Two regional studies independently showed that the partial pressure of CO2 (pCO2) in rivers increases with increasing wetland coverage in the catchment. Borges et al. (2015) established a relationship between wetland extent and pCO2 for African rivers. Wit et al. (2015) presented an analog synthesis for Southeast Asian rivers, which flow through peatlands. Peatlands are a special type of wetland, where organic matter accumulates at rates that make them the most effective terrestrial carbon store on a millennial timescale (Dommain et al., 2011). Southeast Asian peatlands store 68.5 Gt carbon (Page et al., 2011). The highest riverine dissolved organic carbon (DOC) concentrations reported so far were found in Southeast Asian peat-draining rivers (Alkhatib et al., 2007; Moore et al., 2011; Müller et al., 2015), with an annual average of 68 mg L−1 DOC found in an undisturbed peat-draining river (Moore et al., 2013). Because of these high DOC concentrations, Indonesian rivers may account for 75 % of the DOC flux into the South China Sea (SCS) while accounting for 39 % of the discharge (Huang et al., 2017). Surprisingly, CO2 emissions from these rivers are not exceptionally high (Müller et al., 2015; Wit et al., 2015). This is attributed to a short residence time of the organic matter in the river, allowing little time for decomposition, and the resistance of peat-derived carbon to bacterial degradation. Nevertheless, the CO2 flux from peat-draining rivers to the atmosphere increases with increasing peat coverage in the river basin (Wit et al., 2015), showing that these ecosystems exert an important influence on a river's carbon budget.
Most Southeast Asian peat-draining rivers are disturbed by human activities such as river damming, urbanization, deforestation (Milliman and Farnsworth, 2011) and discharge of untreated wastewater (Park et al., 2018). Anthropogenic change poses a new challenge to understanding carbon fluxes in Asian river systems, and more data are urgently needed to constrain the carbon budget for this important region (Park et al., 2018). In Malaysia, the country holding the second largest share of tropical peat (Page et al., 2011), river CO2 emissions have only been studied in a small undisturbed peat-draining river (Müller et al., 2015), in estuaries (Chen et al., 2013; Müller et al., 2016) and in two river reaches which were not influenced by peat (Müller et al., 2016). In this study, the longest Malaysian river, the Rajang River on the island of Borneo, was investigated. This river flows through largely logged-over tropical rainforest (Gaveau et al., 2014), urban areas and disturbed peat swamps (Gaveau et al., 2016). The aim of this study was to assess the Rajang River and Estuary carbon load and to investigate the impact of peatlands on its CO2 emissions. To this end, we surveyed longitudinal transects extending from river reaches that were not influenced by peat to the peat-covered delta.
2.1 Study area
The Rajang River is located in the Malaysian state of Sarawak in the northern part of the island of Borneo (Fig. 1a). Sarawak has a tropical climate with high temperatures (average 26.6 ∘C, 1992–2016, in Sibu, DWD, 2018) and high precipitation (average 3578 mm yr−1, 1992–2016 in Sibu, DWD, 2018). The region experiences two monsoonal periods: the northeastern monsoon, with enhanced rainfall and frequent floods, occurs between December and February (“wet season”; see Fig. 2a), while the southwestern monsoon from May until September is associated with relatively drier weather (“dry season”). However, despite the monsoon seasons, rainfall is high throughout the year (Sa'adi et al., 2017).
The Rajang River originates in the Iran mountains, a mountain range at the border between Malaysia and Indonesia (MacKinnon, 1996) with elevations of up to 1800 m (Milliman and Farnsworth, 2011). It drains an area of approximately 52 010 km2 (Lehner et al., 2006; DID, 2017) whose geology is dominated by Cenozoic sedimentary and metamorphic rocks, consisting of siliciclastic rock with minor amounts of carbonates (Staub et al., 2000; Milliman and Farnsworth, 2011). The Rajang River flows approximately 530 km from east to west and discharges into the South China Sea (Milliman and Farnsworth, 2011). The main settlements along the river are the towns of Kapit, Kanowit and the city of Sibu (163 000 inhabitants) (see Fig. 1b). In addition, a large number of longhouses (traditional buildings inhabited by local communities) are located along the river and its tributaries (Ling et al., 2017). Hydroelectric power plants were built on two tributaries in the upper Rajang basin: The Bakun hydroelectric power plant commenced operation in 2011 and the Murum dam in 2015 (Sarawak Energy, 2013; see Fig. 1b). The construction of another hydroelectric power plant on a tributary in the southern Rajang basin is planned for the future (Sarawak Energy, 2013).
The Rajang delta system is comprehensively described in Staub and Gastaldo (2003). It is entirely surrounded by peatlands (Fig. 1b), which extend over an area that corresponds to approximately 11 % of the catchment size (Nachtergaele et al., 2009). Most of these peatlands have been converted to industrial oil palm plantations (Gaveau et al., 2016, Fig. 1b). The main distributary channels forming the delta (from north to south) are the Igan, Hulu Seredeng (which splits up into Lassa and Paloh), Belawai and Rajang, which have a maximum tidal range (spring tide) of 3–6 m (Staub and Gastaldo, 2003). Saltwater intrudes into the estuary approximately as far as the point where the Rajang River splits up into its four southernmost distributaries, a few kilometers downstream of Sibu (Fig. 1b), depending on the season. Tidal influence extends further inland approximately up to the town of Kanowit (Staub and Gastaldo, 2003).
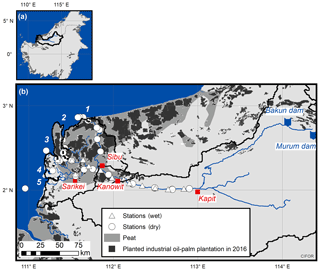
Figure 1Map of the Rajang basin on the island of Borneo (a) and a close-up of the basin (b) including the location of the peatlands (Nachtergaele et al., 2009), industrial oil palm plantations (Gaveau et al., 2016) and the stations during the cruise. The distributaries are marked with numbers: 1: Igan, 2: Lassa, 3: Paloh, 4: Belawai, 5: Rajang.
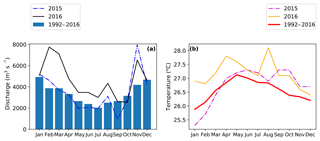
Figure 2Monthly discharge calculated for the Rajang River (a) and average temperatures (b) for the city of Sibu (2∘20′ N, 111∘50′ E) for the years 2015 and 2016 and the long-term average from 1992 to 2016. Data from DWD (2018).
Monthly discharge (Fig. 2a) was estimated from monthly precipitation (1992–2016; DWD, 2018) and an evapotranspiration rate of 1545 mm yr−1 (Kumagai et al., 2005) or 43.2 %. Annual average discharge from 1992 to 2016 was 3355 m3 s−1, in good agreement with reported discharges of 3490 m3 s−1 (Milliman and Farnsworth, 2011) and 3372 m3 s−1 for the years 1991–2015 (Sa'adi et al., 2017).
2.2 Surveys
We sampled the Rajang River during two surveys, which were designed to get spatial coverage of both peat and nonpeat areas during the wettest and driest period of one year. The first survey took place at the peak of the monsoon season in January 2016 (“wet season”). The second one was performed during the dry season in August 2016 (“dry season”). In January 2016, we entered the Rajang River through the Rajang river mouth (distributary 5 in Fig. 1b), went upstream to the town of Kapit and back downstream to the town of Belawai at the Belawai river mouth (distributary 4 in Fig. 1b). In August 2016, we entered the Rajang River through the Rajang river mouth (5), went upstream to Kapit and back to Sibu. From there, we went out to the coast through the Lassa distributary (2), and back to Sibu through the Igan distributary (1). The last sampling stretch was from Sibu into the Paloh distributary (3) and back to Belawai (4). During this campaign, one stationary measurement was performed overnight in Sarikei in the Rajang distributary in order to assess tidal and diurnal variability.
2.3 CO2 measurements
The setup on the boat was similar to the one described in Müller et al. (2016). Surface water was pumped through a shower-type equilibrator (Johnson, 1999) at a rate of approximately 15 L min−1. In the beginning, the equilibrator headspace was connected to an FTIR analyzer (Griffith et al., 2012), which allows for the simultaneous measurement of CO2, methane (CH4), nitrous oxide (N2O) and carbon monoxide (CO). During the cruise in January 2016, a failure of the FTIR analyzer occurred and measurements were continued (also in August 2016) using an Li-820 non-dispersive infrared (NDIR) analyzer for the measurement of CO2 (Licor, USA). For calibration and inter-calibration of the two instruments, a set of gravimetrically prepared gas mixtures (DEUSTE Steininger) was measured, which were calibrated against the World Meteorological Organization (WMO) standard scale by the Max Planck Institute for Biogeochemistry in Jena, Germany. For the FTIR, spectra were averaged over 5 min and dry air mole fractions were retrieved using the software MALT5 (Griffith, 1996). Li-820 data were stored with a temporal resolution of 1 min. Gas partial pressure was determined using measurements of ambient pressure with a PTB110 barometer (Vaisala, Finland) and correction for removal of water according to Dickson et al. (2007). Water temperature was measured in the equilibrator and in the surface water and correction to water surface temperature was performed according to Dickson et al. (2007).
In August 2016, the internal pressure sensor of the Li-820 failed. Because the instrument performs an internal correction based on the cell pressure, this correction had to be reversed and recalculated with an assumed internal cell pressure. This procedure is described in the Supplement.
CO2 fluxes (FCO2, in g C m−2 d−1) across the water–air interface were computed using the gas transfer equation
where k is the gas transfer velocity (m s−1), K0 is the solubility (mol L−1 atm−1) calculated according to Weiss (1974), pCO is the partial pressure of CO2 in water, pCO is the partial pressure of CO2 in the overlying air (both in µatm), f1 is a conversion factor from L−1 to m−3, and f2 is a conversion factor from µmol s−1 to mg d−1. The atmospheric mole fractions of CO2 during the months of our measurements were derived from the NOAA ESRL Carbon Cycle Cooperative Global Air Sampling Network (Dlugokencky et al., 2018) for the closest station, which was Bukit Kototabang, Indonesia.
The gas transfer velocity (k) is a critical yet poorly constrained parameter. Many studies have attempted to relate k to the main drivers of turbulence, such as wind speed (e.g., Wanninkhof, 1992; Nightingale, 2000; Raymond and Cole, 2001) or, especially for rivers, catchment parameters like slope, water flow velocity and discharge (Raymond et al., 2012). We sampled mainly the downstream reaches of the Rajang River, which range in width from 271 m to several kilometers in the delta (Allen and Pavelsky, 2018). Therefore, k parameterizations that were developed for estuaries or big rivers were considered the most appropriate. We compared three parameterizations to constrain the CO2 fluxes. The first parameterization is the one by Borges et al. (2004) for estuaries, which is driven both by wind speed and water flow velocity; the one by Alin et al. (2011), which was developed for rivers wider than 100 m and is driven by wind speed; and the one by Raymond and Cole (2001), which is driven by wind speed and was developed for big rivers and estuaries. Those parameterizations read as follows:
where w is the water flow velocity (cm s−1), h is the depth (m) and u10 is the wind speed at 10 m (m s−1). The depth h was taken from the bottom sounder recordings of our boat, and w in the lower river reaches was measured by Staub and Esterle (1993) to be 0.7 m s−1. A more recent study by Ling et al. (2017) reports w= 1.1 m s−1 for the Rajang River upstream from Kapit. For the calculation of the gas exchange velocity k, we used the average of w=0.9 m s−1. For u10, on-site wind speed data were unfortunately not available. In such cases, other authors (e.g., Bouillon et al., 2012; some estuaries in Chen et al., 2013) have resorted to gridded wind data from the NOAA NCEP–NCAR reanalysis product (Kalnay et al., 1996). While we acknowledge the uncertainty introduced by using gridded data instead of in situ wind speed, we used this product as well, as it is the best one available for our study area. We retrieved daily wind speed at 10 m for the grid centered at 2.85∘ N, 112.5∘ E for the time of our measurements. In situ k is dependent on in situ salinity and temperature and was calculated from k600, exploiting its relationship with the Schmidt number (Wanninkhof, 1992).
The calculation of fluxes using k parameterizations is associated with a large uncertainty, and it is difficult to determine the most suitable parameterization if none of them was developed in the study region. In addition, using input data from the literature (as for the water flow velocity) or gridded instead of measured wind data adds to this uncertainty (see Supplement). However, using three different parameterizations we are able to constrain the magnitude of CO2 emissions from the Rajang River. We will report the average CO2 fluxes from the three different parameterizations as well as minimum and maximum fluxes.
2.4 Ancillary measurements
In January 2016, individual water samples were taken at 15 stations between the river mouth and Kapit, including the distributary channels Rajang and Belawai. In August 2016, water samples were taken at 34 stations, with a higher sampling frequency and coverage in the delta (Rajang, Igan, Lassa, Paloh and Belawai, Fig. 1b). Water samples were taken from approximately 1 m below the surface using a Van Dorn water sampler. Particulate material was sampled on pre-weighed and pre-combusted glass fiber filters. From the net sample weight and the volume of filtered water, the amount of suspended particulate matter (SPM) was determined. For POC, 1 molar hydrochloric acid was added in order to remove inorganic carbon from the sample. For the determination of carbon, samples were catalytically combusted at 1050 ∘C and combustion products were measured by thermal conductivity using a Euro EA3000 Elemental Analyzer. Repeatability for C content was 0.04 % (standard deviation).
In August 2016, water samples were also taken for the determination of dissolved inorganic carbon (DIC) and the isotopic composition (δ13C) of DIC, because the isotopic composition of DIC can help in identifying its sources (Das et al., 2005; Campeau et al., 2017, 2018). Samples were poisoned with 200 µL concentrated HgCl2 and filtered through Whatman glass fiber filters (GF/F, pore size 0.7 µm). Samples were filled into 40 mL vials leaving no headspace, checked for the existence of bubbles, and stored refrigerated until analysis. Concentrations and δ13C of DIC were measured via continuous-flow wet-oxidation isotope ratio mass spectrometry (CF-WO-IRMS) using an Aurora 1030W TOC analyzer coupled to a Thermo Delta V Plus IRMS (Oakes et al., 2010). Sodium bicarbonate (DIC) of known isotope composition dissolved in helium-purged milli-Q was used for drift correction and to verify concentrations and δ13C values. Reproducibility for DIC was ±10 µmol L−1 for concentrations and ±0.10 ‰ for δ13C (standard deviations).
During both surveys, dissolved oxygen and water temperature were continuously measured with a temporal resolution of 5 min using an FDO 925 oxygen sensor and a WTW 3430 data logger (Xylem Inc., USA). The oxygen sensor was calibrated by the manufacturer, and a routine function check was performed before the start of measurements using the check and calibration vessel (FDO© Check) provided by the company. The reported accuracy of a dissolved oxygen measurement at 20 ∘C in air-saturated water is 1.5 %, and the precision of the accompanying temperature measurement is 0.2 ∘C (WTW, 2012). pH, salinity and temperature were measured at the stations, using a SenTix 940 pH sensor and a multiprobe (Aquaread AP-2000). The pH sensor was calibrated before the start of the measurements using NIST (National Institute of Standards and Technology) traceable buffers. Since salinity was only measured at the stations, we spatially interpolated salinity for the interpretation of pCO2 data. This procedure is described in the Supplement.
Different geographical extents of the river were covered during the two campaigns, and the salt intrusion limits were different during the two seasons. In order to keep the results from the two surveys comparable, we report results for three categories: nonpeat (Kapit–Kanowit), peat (Kanowit–Sibu) and delta (downstream of Sibu). Their definition and properties are specified in Table 1. The nonpeat and peat areas are directly comparable between seasons, because the same spatial extent was covered during both surveys and they were non-saline during both seasons.
2.5 Data analysis and export calculations
Data analysis was performed with Python 2.7.15 and ArcMap 10.5. Averages of measured parameters are reported ±1 standard error unless stated otherwise. Errors for calculated parameters (e.g., river loads; see below) were determined with error propagation. For fluxes and derived quantities, we report the mean, minimum and maximum from the three k parameterizations. Seasonal differences were tested for significance using the Mann–Whitney U test from the Python Scipy Statistical Functions module. Data from the delta were excluded from the statistical tests due to the different geographical coverage achieved during the two surveys.
In order to calculate the total carbon export from the Rajang River for the months of our measurements, we derived DOC load, POC load, DIC load for the peat and nonpeat area, and CO2 outgassing as follows:
The river loads of DOC, POC and DIC were calculated for the peat and nonpeat area combined, using
where C is the average concentration of DOC, POC and DIC (mg L−1), Q is monthly discharge (m3 s−1) and f3 is a conversion factor from s−1 to month−1.
For DOC, we used the DOC concentrations reported by Martin et al. (2018). These data were acquired during 2017 downstream of Kanowit. Only freshwater values were considered (average for the wet and dry season: 2.0 and 2.1 mg L−1). For POC, we used the area-weighted average concentration of peat and nonpeat river reaches determined during our surveys.
For DIC, we used an area-weighted average concentration as well, which was determined from our measurements during the dry season. For the wet season survey, DIC was calculated from pH and pCO2 using the program CO2sys (Lewis and Wallace, 1998). Note that pH measurements were only available at the stations, and sometimes we did not have parallel pCO2 measurements. Therefore, the number of calculated DIC values for the peat and nonpeat area is 6.
For the calculation of total CO2 emissions from FCO2, the river surface area was required. River surface area was calculated from the GRWL (Global River Widths from Landsat) database (Allen and Pavelsky, 2018) using Esri's ArcMap 10.5. Missing segments in the delta were manually delineated using a Landsat satellite image and their surface area was determined. This procedure is described in the Supplement. With the surface area of individual river segments at hand, CO2 emissions were calculated for the nonpeat area, peat area and the delta separately.
A Keeling plot (Keeling, 1958) was used to explore possible sources of DIC in the Rajang River. The Keeling plot method has been used to determine the isotopic signature in CO2 from ecosystem respiration (Pataki et al., 2003; van Asperen et al., 2017) by plotting δ13C of CO2 in an air sample vs. the inverse CO2 concentration. From the y intercept of a linear regression, the isotopic signature of the source can be determined (Keeling, 1958; Pataki et al., 2003). The Keeling plot method assumes mixing of only two components: One background component (e.g., atmospheric background) and one additional source (e.g., respiration). While originally designed for atmospheric research, it has also been used in studies exploring possible sources of DIC in stream water (Campeau et al., 2017, 2018). However, it has to be interpreted with caution, because rivers are open systems where the basic assumption of two-component mixing is easily violated. When we interpret the Keeling plot in this study, we look at mixing between river DIC and added DIC from groundwater and peat-draining rivers. We thus have to assume that DIC in the main river does not change over the time of our measurements. Since the water is continuously replenished from upstream, this assumption is valid as long as the upstream (background) source's isotopic signature remains the same. This can be assumed during a relatively short sampling period (7 days). Nevertheless, we can only consider freshwater data, as mixing with seawater already constitutes a third component. Therefore, we show only freshwater data in our Keeling plot and caution that its interpretation is based on the relatively strong assumption that we can view the river's main stem δ13C of DIC as constant over the sampling period.
3.1 General characterization of the Rajang River
Measured salinity ranged between 0 and 18.6 during the wet season and 0 and 32.1 during the dry season. Saltwater was detected further upstream during the dry season than during the wet season (Fig. 3a and b). Saltwater penetrated further inland in the Rajang and Belawai distributaries than in the Igan distributary (Fig. 3b), suggesting that most freshwater is discharged via the Igan distributary.
The Rajang River was slightly acidic (wet: 6.7; dry: 6.8), area-weighted mean for the peat and nonpeat area; see Table 2) and highly turbid, with area-weighted average SPM concentrations of 187.2±75.7 mg L−1 (wet season) and 51.5±12.1 mg L−1 (dry season; see Table 2). With higher SPM during the wet season (p<0.001), the organic carbon content of SPM was significantly decreased (1.5±0.4 % on average, p=0.01) compared to the dry season (2.1±0.6 % on average; see Table 2). POC ranged from 0.7 to 9.1 mg L−1 during the wet season (average 2.6±0.6 mg L−1) and from 0.3 to 1.9 mg L−1 during the dry season (average 1.1±0.4 mg L−1; see Table 2). The seasonal difference was significant (p=0.002).
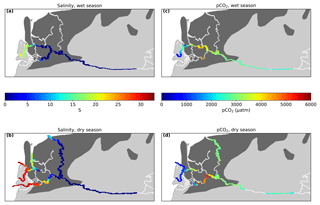
Figure 3Salinity (interpolated) and pCO2 distribution in the Rajang River and delta during the wet season survey (a, c) and dry season survey (b, d).
Table 2Average values of measured parameters for different river reaches (mean ± 1 SE) for all parameters except FCO2, which is reported as mean (minimum − maximum).
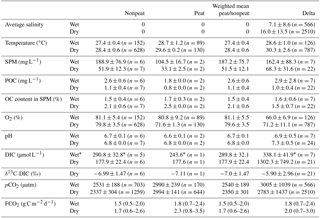
* Calculated, not measured values.
The river water was consistently undersaturated with dissolved oxygen (DO) with respect to the atmosphere. DO oversaturation was not observed. The area-weighted average DO was similar during the wet and dry seasons (wet: 81.1±5.5 %; dry: 79.6±3.5 %), with slightly lower DO in the delta (wet: 66.0±6.9 %; dry: 71.2±11.1 %).
Measured DIC in the dry season ranged from 153.7 µmol L−1 in the nonpeat area to 2399.2 µmol L−1 in the estuary and increased linearly with salinity (Fig. 4a, r=0.98, p<0.001). Concentrations averaged 177.9±22.4 µmol L−1 in the peat and nonpeat area and 1302.3±749.2 µmol L−1 in the delta (Table 2). Calculated DIC for the wet season averaged 289.8±32.1 µmol L−1 (area-weighted mean for the nonpeat and peat area). δ13C-DIC ranged between −11.9 ‰ and −1.4 ‰ and averaged ‰ in the peat and nonpeat area and −5.9 ‰ in the delta (Table 2). δ13C-DIC was positively correlated with DIC for the delta (r=0.81, p<0.001) and negatively correlated with DIC for the freshwater part (peat and nonpeat combined, , p=0.004, Fig. 4b). The Keeling plot for freshwater samples revealed a linear relationship (Fig. 4c) with a y intercept (±SE) of ‰.
3.2 Carbon dioxide
The Rajang River was oversaturated with CO2 with respect to the atmosphere, with an average pCO2 of 2531±188 µatm (wet season) and 2337±304 µatm (dry season) in the nonpeat area. pCO2 was significantly higher in the peat area (p<0.001, both seasons), with 2990±239 µatm (wet season) and 2994±141 µatm (dry season). The area-weighted means for the peat and nonpeat areas were 2540±189 µatm (wet season) and 2340±301 µatm (dry season). In the delta, pCO2 was more variable, and the average values of 3005±1039 µatm (wet season) and 2783±1437 µatm (dry season; see Table 2) were also significantly higher than in the nonpeat area (p<0.001, both seasons). pCO2 values were strikingly similar between wet and dry seasons, and so were the spatial patterns in pCO2 (Fig. 3c and d). Tidal variability of pCO2 was observed at an overnight station in Sarikei in August 2016. During this time, pCO2 increased from approximately 3000 to almost 6000 µatm during rising tide (see Supplement).
pCO2 decreased with increasing salinity in the delta (Fig. 3). However, a big spread of data in both the high-salinity region (during the tidal measurement described above) and the freshwater region was observed. pCO2 was correlated with DO (Fig. 5). An interesting pattern is consistently visible in both the wet and dry season data, by which main stem data can clearly be distinguished from those collected in the Belawai and Paloh distributaries. Unfortunately, our data did not allow identification of a diurnal signal for either pCO2 or DO. In the tidal part of the river, we had only one stationary measurement overnight, when a diurnal signal could not be identified due to the strong tidal signal. In the non-tidal part of the river, we had insufficient nighttime data to make a statement about a day–night difference for pCO2 and DO.
Wind speed in the grid centered at 2.85∘ N, 112.5∘ E averaged 0.57 m s−1 during our campaign in January 2016 and 1.09 m s−1 during our campaign in August 2016. The calculated gas exchange velocities for a Schmidt number of 600 using the B04 model (k600,B04) were 8.2 and 9.6 cm h−1, respectively. This compares to the A11 model with 8.5 and 12.2 cm h−1 and to the R01 model with 2.3 and 2.8 cm h−1 for the wet and dry season, respectively. The resultant CO2 fluxes (FCO2) to the atmosphere ranged between 0.5 and 2.4 g C m−2 d−1 in the wet season and between 0.6 and 3.5 g C m−2 d−1 in the dry season (per water surface unit area; see Table 2).
3.3 Carbon river load and CO2 emissions
Discharge was above average during 2016 (Fig. 2a). Discharge during January 2016 (wet season) was in accordance with the long-term average, but discharge during August 2016 (dry season) was higher than usual. The Rajang River loads for the peat and nonpeat area were 104 Gg C (82–123 Gg C) in January 2016 and 65 Gg C (45–83 Gg C) in August 2016; another 31 Gg C (12–41 Gg C) (wet) and 34 Gg (12–51 Gg C) were emitted as CO2 from the delta (Table 3). Of the river loads of carbon, 91 % (86–97 %) (wet) and 82 % (70–94 %) (dry) were exported laterally by discharge. Approximately half of the laterally transported carbon was in the organic form (wet: 57±12 %; dry: 60±18 %). River loads were similar during both seasons, except that POC export was 3-fold higher in the wet season. CO2 emissions to the atmosphere accounted for 9 % (3–14 %) and 18 % (6–30 %) (wet and dry) of the total carbon load of the river.
4.1 Sediment yield and organic carbon load
The Asia-Pacific region is known for its high sediment yields, especially where rivers drain Cenozoic sedimentary and volcanic rock (Milliman and Farnsworth, 2011). Therefore, the high suspended sediment load in the Rajang River is not surprising. However, SPM concentrations during our expeditions were substantially lower (187.2±75.7 and 51.5±12.1 mg L−1) than in July 1992 (613 mg L−1; Staub and Esterle, 1993). This could be an effect of upstream dams (operational since 2011 and 2015), which trap sediment in their reservoirs, thereby reducing downstream sediment loads (Vörösmarty et al., 2003; Snoussi et al., 2002). In support of this, SPM concentrations were intermediate in the upper Rajang River in 2014–2015 (218.3 mg L−1; Ling et al., 2017). These measurements were taken before, and the measurements in the current study were taken after, the Murum dam began full operation in the second quarter of 2015. Furthermore, SPM and POC concentrations (wet: 2.6; dry: 1.1 mg L−1) in the Rajang River were similar to those in the Pearl River, China, (SPM: 70–247 mg L−1, POC: 1.0–3.8 mg L−1; Ni et al., 2008) and the Red River, Vietnam, (SPM: 294±569 mg L−1 (wet) and 113±428 mg L−1 (dry), POC: 3.7±2.0 mg L−1 (wet) and 1.1±1.1 mg L−1 (dry); Le et al., 2017), both of which are also affected by damming.
SPM was higher during the wet season than during the dry season, in agreement with observations at the Kinabatangan River, Malaysia (Harun et al., 2014). This can be attributed to enhanced erosion during the wet season. In logged-over forest, such as that found in most of the Rajang River basin, the energy impact of rain drops on the soil is higher than in densely vegetated areas, where rain is intercepted by the canopy before falling on the ground (Ling Lee et al., 2004). In agreement with this line of reasoning, Ling et al. (2016) showed that the amount of suspended solids in Malaysian streams draining areas with logging activities increased significantly after rain events. The decreased organic carbon content observed during the wet season further supports this, as it indicates a higher contribution of eroded mineral soil to SPM. This pattern is observed in many rivers in this region (Huang et al., 2017). Despite the changing carbon content, most POC was still exported during the wet season, as in other Southeast Asian rivers (Ni et al., 2008; Moore et al., 2011). The proportion of laterally transported carbon in the Rajang River that is in organic form (wet: 57±12 %; dry: 60±18 %) is similar to that reported for the carbon flux to the South China Sea (50±14 %, Huang et al., 2017).
4.2 Inorganic carbon load
4.2.1 DIC concentrations and sources
DIC concentrations measured during our dry season survey were comparable to those determined by Huang et al. (2017) for the Rajang River (201 and 487 µmol L−1). Their values are based on seven measurements taken between 2005 and 2009 downstream of Sibu (Ting-Hsuan Huang, personal communication, 2018). DIC concentrations in the Rajang River thus appear to be substantially lower than in the Mekong River (1173–2027 µmol L−1; Li et al., 2013) and the Pearl River (1740 µmol L−1; Huang et al., 2017), but similar to those in the Musi River, Indonesia (250 µmol L−1, Huang et al., 2017). This suggests that the Rajang River compares better to the equatorial Indonesian rivers than to rivers draining mainland Southeast Asia, probably because of the scarcity of carbonate rock, which has the highest weathering rate and is thus responsible for high DIC in rivers (Huang et al., 2012).
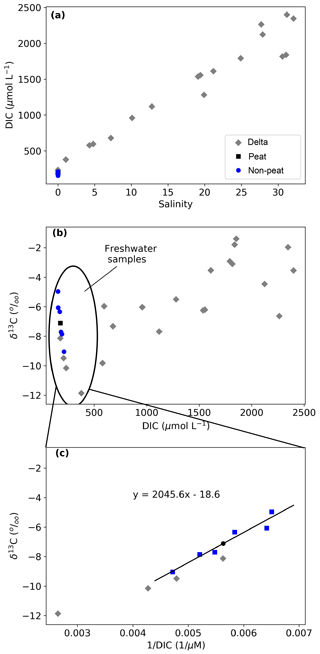
Figure 4DIC vs. salinity (a), δ13C vs. DIC (b) and a Keeling plot of δ13C vs. 1∕DIC for nonpeat, peat and delta samples, respectively. Freshwater samples, including those from the delta region, are circled in panel (b), and only those are shown in the Keeling plot in panel (c). All data refer to dry-season samples.
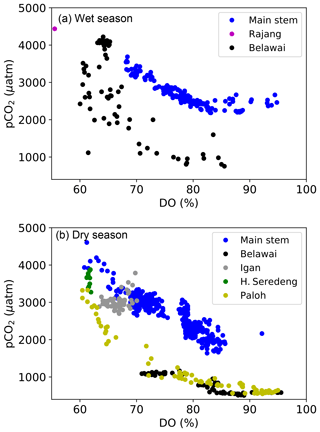
Figure 5Correlation of pCO2 vs. dissolved oxygen (DO) in the wet and dry season for individual distributaries.
The source of DIC varied along the length of the Rajang River. In the estuarine part, the positive linear relationship between DIC and salinity (Fig. 4a) suggests that the main source of DIC in the estuary is marine. This is also supported by the relatively high δ13C-DIC of estuarine samples, as ocean DIC is more enriched, with δ13C-DIC between 0 ‰ and 2.5 ‰ (Rózanski et al., 2003). The positive correlation between DIC and δ13C in the delta thus implies an increasing contribution of marine DIC.
In the freshwater part of the Rajang River, δ13C values were more depleted ( ‰, Table 2) than downstream, but were more enriched than δ13C-DIC values reported for other rivers in the region (Lupar and Saribas Rivers in Sarawak, −15.7 ‰ to −11.4 ‰, Müller et al., 2016; Musi, Indragiri and Siak Rivers in Indonesia, −22.5 ‰ to −9.0 ‰, Wit, 2017).
Multiple sources and processes are likely to influence δ13C-DIC in the Rajang River. To start with, the y intercept of the Keeling plot for freshwater samples suggests that the DIC added to the main river has a δ13C of −18.6 ‰, which is consistent with δ13C values of bicarbonate from silicate weathering with soil CO2 from C3 plants (−22.1 ‰ to −16.1 ‰, Das et al., 2005), i.e., groundwater input.
Another relevant source is rainwater DIC with a typical δ13C of −9.3 ‰ (Das et al., 2005). In a river with relatively low DIC (177.9 µmol L−1) and large surface runoff due to heavy rain, this source term is presumably non-negligible, and a significant contribution would partially explain the relatively high δ13C-DIC values.
With regard to in-stream processes, photosynthesis increases and respiration decreases δ13C-DIC (Campeau et al., 2017). Due to the high turbidity, it can be assumed that photosynthesis in the Rajang River is negligible. In contrast, the correlation of DO and pCO2 (Fig. 5) suggests that respiration is important. This assumption is supported by the negative correlation of δ13C and DIC for freshwater samples, because with increasing DIC, δ13C values get more depleted, suggesting that organic carbon (with a δ13C of around −26 ‰ for C3 plants; Rózanski et al., 2003) is respired to CO2 within the river. However, we observed relatively high δ13C values overall. Two processes are likely to be responsible for the downstream increase in δ13C: (1) methanogenesis and (2) evasion of CO2. (1) Campeau et al. (2018) observed a strong relationship between CH4 concentration and δ13C-DIC in a boreal stream draining a nutrient-poor fen, suggesting that fractionation during methanogenesis leads to an increase in δ13C-DIC. As the peat soils in the Rajang delta are also anaerobic and nutrient-poor, it is likely that methanogenesis plays a role there as well. This is consistent with high reported soil CH4 concentrations of up to 1465 ppm in a peat under an oil palm plantation in Sarawak (Melling et al., 2005). It would therefore be of great interest to investigate CH4 concentrations in the Rajang River in the future. (2) CO2 evasion is also known to lead to a gradual increase in δ13C-DIC values until in equilibrium with the atmosphere (with a slightly positive δ13C-DIC; Polsenaere and Abril, 2012; Venkiteswaran et al., 2014; Campeau et al., 2018). Due to intracarbonate equilibrium fractionation, dissolved CO2 is more depleted in δ13C than the other carbonate species. Thus, if it is removed, δ13C of the remaining DIC increases. This effect depends on pH and is more pronounced in near-neutral waters and less strong in very acidic water (Campeau et al., 2018). We sampled the lower river reaches downstream of Kapit, which corresponds to approximately the last 200 km of the river. In addition, the terrain is much steeper upstream of Kapit than in the lower river reaches, so that CO2 fluxes to the atmosphere are presumably much higher due to higher turbulence. This means that a large fraction of CO2 had likely already been emitted from the river surface before reaching Kapit, leading to the observed high δ13C-DIC values.
4.2.2 pCO2
pCO2 in the Rajang River was higher than in most Southeast Asian rivers without peat influence, such as the Mekong River (pCO2=1090 µatm; Li et al., 2013), the Red River (pCO2=1589 µatm; Le et al., 2017) or the freshwater parts of the Lupar and Saribas Rivers (pCO2=1274 µatm and 1159 µatm; Müller et al., 2016, Table 4, Fig. 6). This could be attributed to the peat influence. However, the Rajang River has a pCO2 at the low end of values reported for peat-draining rivers (Fig. 6): Wit et al. (2015) report values between 2400 µatm in the Batang Hari (peat coverage = 5 %) and 8555 µatm in the Siak River (peat coverage = 22 %).
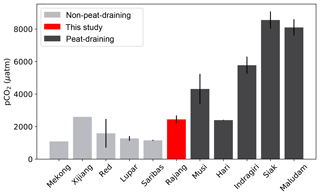
Figure 6Comparison of average pCO2 values in Southeast Asian rivers. Colors distinguish peat-draining rivers from nonpeat-draining rivers. The Rajang River (this study) is highlighted in red.
A meaningful comparison is also the one between the Rajang River and the Indragiri River, Indonesia, because they have a similar peat coverage (Rajang: 11 %, Indragiri: 12 %) and peat coverage has previously been considered as a good predictor of river CO2 emissions (Wit et al., 2015). However, pCO2 in the Indragiri (5777 µatm) was significantly higher than in the Rajang, which was associated with a lower pH (6.3, numbers from Wit et al., 2015). A simple exercise using CO2Sys illustrates how important it is to consider both pH and pCO2 when comparing different peat-draining rivers. At the given temperature, salinity and pH, the pCO2 of 5777 µatm in the Indragiri corresponds to a DIC value of 327 µmol L−1. At a hypothetical pH of 6.8, as measured in the Rajang River, this DIC value corresponds, under otherwise unchanged conditions, to a pCO2 of 2814 µatm – which is very close to the average values measured in the peat area of the Rajang River. The close coupling of pH and pCO2 implies that peat coverage in a river basin is insufficient as the sole predictor of CO2 fluxes. Rather, the relationship between peat coverage and pCO2 must be viewed in the context of the rivers' pH, and drivers of pH must be more carefully considered. Note also that peat coverage is usually reported for the entire catchment (e.g., Wit et al., 2015; Rixen et al., 2016) and does not reveal how much peat is found in estuarine vs. freshwater reaches, which complicates comparisons further.
δ13C-DIC in the Indragiri was lower (−16.8 ‰, Wit, 2017) than in the Rajang (−7.0 ‰). While this can be partially attributed to the different pH, it can also be interpreted as a greater contribution of respiratory CO2 in the Indragiri, while the Rajang might be more strongly influenced by weathering. This would also help to explain the higher pH in the Rajang River.
While DOC and pCO2 are positively related to discharge in most rivers (e.g., Bouillon et al., 2012), this pattern is sometimes reversed in peat-draining rivers. This is due to dilution, when rainfall exceeds the infiltration capacity of the wet soil and water runs off at the surface (Clark et al., 2008; Rixen et al., 2016). In the Rajang River, pCO2 was slightly higher in the nonpeat area during the wet season, in agreement with many nonpeat-draining tropical rivers (Bouillon et al., 2012; Teodoru et al., 2015; Scofield et al., 2016). However, the seasonality of pCO2 was very small, similar to other Malaysian rivers (Müller et al., 2016) and in line with the small seasonal variability of DOC concentrations in the Rajang River (Martin et al., 2018). Note that since our data were collected during two single surveys, they represent only a snapshot and do not allow strong claims about seasonality.
Due to an El Niño event, temperatures in Southeast Asia were unusually high in late 2015 and 2016, with a temperature extreme in April 2016 prevailing in most of Southeast Asia (Thirumalai et al., 2017) and unusually hot conditions also recorded in Sibu (Fig. 2b). Given that weathering rates increase at elevated temperatures, DIC from weathering could have been enhanced over other years, although this seems unlikely because DIC was relatively low and in agreement with previous studies. Another factor to be taken into account is that decomposition in the dry upper soil layer is more intense at higher temperatures, and with incipient rainfall, all the resultant DOC is flushed out to the river. Therefore, it is possible that during the year of our measurements, DOC concentrations were higher than usual, and respiratory CO2 may therefore have been enhanced compared to other years.
4.2.3 Impact of the peatlands on the CO2 emissions from the Rajang River
The fact that pCO2 was significantly higher in the peat area than in the nonpeat area implies, at first glance, that the peat areas are a source of CO2 to the river. However, this difference has to be interpreted with caution, as the entire peat area is under tidal influence (Fig. 1b). In the following, we will present several arguments that suggest that the peatlands exert only a small influence on the CO2 emissions from the Rajang River.
- a.
DOC. One indicator of peatland influence on a river's carbon budget is DOC. DOC concentrations in the Rajang River delta were reported to range between 1.4 and 3 mg L−1 (Martin et al., 2018). This is at the low end of the range of DOC concentrations reported for peat-draining rivers in Indonesia: These range from 2.9 mg L−1 in the Musi River (peat coverage = 3.5 %) up to 21.9 mg L−1 in the Siak River (peat coverage = 22 %; Wit et al., 2015). Rivers whose catchment area is entirely covered by peat exhibit even higher DOC concentrations, with 52 mg L−1 (wet season) and 44 mg L−1 (dry season) in the Sebangau River, Indonesia (Moore et al., 2011), and 44 mg L−1 in the Maludam River, Sarawak, Malaysia (Müller et al., 2015). The Rajang River compares rather to rivers like Lupar and Saribas, Malaysia, which exhibit DOC concentrations of 1.8 and 3.7 mg L−1 in their freshwater parts (no peat influence, Müller et al., 2016). Consequently, DOC concentrations imply that the peatlands' influence on the Rajang's DOC is rather small.
- b.
Mixing model. An alternative approach is to theoretically calculate the increase in Rajang River pCO2 that would result from the influx from peatlands. For this, we created a simple model to simulate the mixing of two water masses (see Supplement), one with a pH of 6.8 and a pCO2 of 2434 µatm (designed to resemble the Rajang River, nonpeat area) and the other with a pH of 3.8 and a pCO2 of 8100 µatm (designed to resemble peat-draining tributaries, based on values for the peat-draining Maludam River in Sarawak, Müller et al., 2015). For simplicity, we assumed that mixing occurs at salinity = 0 and that the temperature in both water bodies is the same (28.4 ∘C). From these values, DIC and total alkalinity (TA) of the two water bodies were calculated using CO2Sys. Since they can be assumed to be conservative, we simply calculated DIC and TA of the mixture as
where pc is the peat coverage in the basin (pc = 0.11). From DIC and TA, the pCO2 of the mixture was computed (pCO2=3058 µatm). This means that if all peat-draining tributaries in the Rajang River basin had a pCO2 of 8100 µatm and a pH of 3.8, the pCO2 in the peat area would be enhanced by around 600 µatm. However, this increase in pCO2 is obviously gradual. For example, in the city of Sibu, peat coverage was estimated at around 2 %, for which the described mixing model yields a pCO2 of 2548 µatm (Fig. S3). In most parts of the delta, dilution with seawater already plays a role. Therefore, the mixing model was extended, assuming that at pc = 3 %, salinity is still zero and then linearly increases until pc = 11 %, S=32, DIC µmol L−1 and TA µmol L−1 (two end-member mixing model; see Supplement). As a result, pCO2 would theoretically not exceed 2605 µatm if the peat-draining tributaries were the only source of CO2 downstream of Kanowit (Fig. S3).
However, pCO2 in the peat area was 2990 µatm (wet) and 2994 µatm (dry) and 3005 µatm (wet) and 2783 µatm (dry) in the delta, so there must be another source of CO2. Since pCO2 in Sarikei varied 2-fold with the tidal cycle, it seems likely that a large part of the difference in pCO2 between the nonpeat area and peat area is actually a difference between river and tidal river. Tidal variability is often seen in estuaries (e.g., Bouillon et al., 2007; Oliveira et al., 2017), largely due to conservative mixing. However, during rising tide in Sarikei, we observed pCO2 values of almost 6000 µatm, which is higher than the freshwater end-member, suggesting that other effects also play a role. Among those are decomposition of organic matter in intertidal sediments (Alongi et al., 1999; Cai et al., 1999) and subsequent transport of the produced CO2 to the river, as well as groundwater input (Rosentreter et al., 2018).
- c.
δ13C-DIC. If the peatlands acted as a significant source of CO2 to the Rajang River, it would be expected that this had an impact on the δ13C-DIC values. In the peat-draining Maludam River, δ13C-DIC averaged −28.55 ‰ (Müller et al., 2015). Thus, the influx of peat-draining tributaries to the Rajang River (with an average δ13C-DIC of −7 ‰) would theoretically decrease δ13C-DIC. This was not observed. Instead, the main source identified by the Keeling plot was consistent with groundwater input, with a δ13C-DIC of −18.6 ‰. However, given the constraints on the applicability of the Keeling plot method discussed above, the effect of DIC inputs from peat on δ13C-DIC might simply not be detectable. Other processes which influence δ13C-DIC as discussed above might also prevent identification of a peat signal in the δ13C-DIC data.
In summary, we were unable to discern a large impact of peatlands on the DIC budget of the Rajang River. It is possible that, because the peatlands are located close to the coast in this system, mixing with seawater occurs before significant effects on the pCO2 are theoretically possible. This means that not only the peat coverage in the catchment is relevant, but also how much of this peat is found in estuarine reaches. These findings support the arguments of Müller et al. (2015) and Wit et al. (2015) that material derived from coastal peatlands is swiftly transported to the ocean, explaining why peat-draining rivers may not necessarily be strong sources of CO2 to the atmosphere.
The Rajang River is a typical Southeast Asian river, transporting large amounts of terrestrial material to the South China Sea. In contrast to other Southeast Asian rivers with similar peat coverage, the impact of the peatlands on the Rajang River's pCO2 appeared to be rather small, probably due to the proximity of the peatlands to the coast. As a consequence, CO2 emissions from the Rajang River were moderate compared to other Southeast Asian rivers and low if compared to Southeast Asian peat-draining rivers.
Calibrated data used in this paper are available as a Supplement table to this paper. Raw data are available at the Institute of Environmental Physics, University of Bremen, Bremen, Germany, and will be provided upon request by Denise Müller-Dum, Thorsten Warneke, or Justus Notholt.
The supplement related to this article is available online at: https://doi.org/10.5194/bg-16-17-2019-supplement.
DMD, TW, TR and MM designed this study. DMD performed all measurements and sample preparations during the first survey. AC, AB and MM performed the measurements and sample preparations during the second survey. JO and BE analyzed the DIC and isotopic data, and DMD analyzed all other data. All coauthors contributed to the interpretation and discussion of the results. DMD prepared the paper with contributions from all coauthors.
The authors declare that they have no conflict of interest.
This article is part of the special issue “Biogeochemical processes in highly dynamic peat-draining rivers and estuaries in Borneo”. It is not associated with a conference.
We would like to thank the Sarawak Biodiversity Centre for permission to
conduct research in Sarawak waters (permit no. SBC-RA-0097-MM and export
permits SBC-EP-0040-MM and SBC-EP-0043-MM). We also thank Aazani Mujahid
(University of Malaysia Sarawak, Kuching, Malaysia) for her extensive
organizational help in preparing the campaigns. Further, we would like to
thank all students and scientists from Swinburne University of Technology and
UNIMAS in Malaysia for their help during the sampling trips. Lukas Chin and
the “SeaWonder” crew are acknowledged for their support during the cruises.
The study was supported by the Central Research Development Fund of the
University of Bremen, the MOHE FRGS Grant (FRGS/1/2015/WAB08/SWIN/02/1),
SKLEC Open Research Fund (SKLEC-KF201610) and ARC Linkage Grant
LP150100519.
The article processing charges for
this open-access
publication were covered by the University
of Bremen.
Edited by: Steven
Bouillon
Reviewed by: two anonymous referees
Alin, S. R., de Fátima F. L. Rasera, M., Salimon, C., I., Richey, J. E., Holtgrieve, G. W., Krusche A. V., and Snidvongs, A.: Physical controls on carbon dioxide transfer velocity and flux in low-gradient river systems and implications for regional carbon budgets, J. Geophys. Res., 116, G01009, https://doi.org/10.1029/2010JG001398, 2011.
Alkhatib, M., Jennerjahn, T. C., and Samiaji, J.: Biogeochemistry of the Dumai River Estuary, Sumatra, Indonesia, a Tropical Black-Water River, Limnol. Oceanogr. 52, 2410–2417, 2007.
Allen, G. H. and Pavelsky, T. M.: Global extent of rivers and streams, Science, 361, 585–588, https://doi.org/10.1126/science.aat0636, 2018.
Alongi, D. M., Tirendi, F., Dixon, P., Trott, L. A., and Brunskill, G. J.: Mineralization of Organic Matter in Intertidal Sediments of a Tropical Semi-enclosed Delta, Estuar. Coast. Shelf S., 48, 451–467, 1999.
Aufdenkampe, A. K., Mayorga, E., Raymond, P. A., Melack, J. M., Doney, S. C., Alin, S. R., Aalto, R. E., and Yoo, K.: Riverine coupling of biogeochemical cycles between land, oceans, and atmosphere, Front. Ecol. Environ., 9, 53–60, https://doi.org/10.1890/100014, 2011.
Borges, A. V., Vanderborght, J.-P., Schiettecatte, L.-S., Gazeau, F., Ferrón-Smith, S., Delille, B., and Frankignoulle, M.: Variability of the Gas Transfer Velocity of CO2 in a Macrotidal Estuary (the Scheldt), Estuaries, 27, 593–603, 2004.
Borges, A. V., Abril, G., Darchambeau, F., Teodoru, C. R., Deborde, J., Vidal, L. O., Lambert, T., and Bouillon, S.: Divergent biophysical controls of aquatic CO2 and CH4 in the World's two largest rivers, Nature Sci. Rep., 5, 15614, https://doi.org/10.1038/srep15614, 2015.
Bouillon, S., Middelburg, J. J., Dehairs, F., Borges, A. V., Abril, G., Flindt, M. R., Ulomi, S., and Kristensen, E.: Importance of intertidal sediment processes and porewater exchange on the water column biogeochemistry in a pristine mangrove creek (Ras Dege, Tanzania), Biogeosciences, 4, 311–322, https://doi.org/10.5194/bg-4-311-2007, 2007.
Bouillon, S., Yambélé, A., Spencer, R. G. M., Gillikin, D. P., Hernes, P. J., Six, J., Merckx, R., and Borges, A. V.: Organic matter sources, fluxes and greenhouse gas exchange in the Oubangui River (Congo River basin), Biogeosciences, 9, 2045–2062, https://doi.org/10.5194/bg-9-2045-2012, 2012.
Cai, W.-J., Pomeroy, L. R., Moran, M. A., and Wang, Y.: Oxygen and carbon dioxide mass balance for the esuarine-intertidal marsh complex of five rivers in the southeastern U.S., Limnol. Oceanogr., 44, 639–649, 1999.
Campeau, A., Wallin, M. B., Giesler, R., löfgren, S., Mörth, C.-M., Schiff, S., Venkiteswaran, J. J., and Bishop, K.: Multiple sources and sinks of dissolved inorganic carbon across Swedish streams, refocusing the lens of stable C isotopes, Nature Sci. Rep., 7, 9158, https://doi.org/10.1038/s41598-017-09049-9, 2017.
Campeau, A., Bishop, K., Nilsson, M. B., Klemedtsson, L., Laudon, H., Leith, F. I., Öquist, M., and Wallin, M. B.: Stable carbon iosotopes reveal soil-stream DIC linkages in contrasting headwater catchments, J. Geophys. Res.-Biogeo., 123, 149–167, https://doi.org/10.1002/2017JG004083, 2018.
Chen, C.-T. A., Huang, T.-H., Chen, Y.-C., Bai, Y., He, X., and Kang, Y.: Air–sea exchanges of CO2 in the world's coastal seas, Biogeosciences, 10, 6509–6544, https://doi.org/10.5194/bg-10-6509-2013, 2013.
Clark, J. M., Lane, S. N., Chapman, P. J., and Adamson, J. K.: Link between DOC in near surface peat and stream water in an upland catchment, Sci. Total Environ., 404, 308–315, https://doi.org/10.1016/j.scitotenv.2007.11.002, 2008.
Dai, M., Yin, Z., Meng, F., Liu, Q., and Cai, W.-J.: Spatial distribution of riverine DOC inputs to the ocean: an updated global synthesis, Curr. Opin. Env. Sust., 4, 170–178, https://doi.org/10.1016/j.cosust.2012.03.003, 2012.
Das, A., Krishnaswami, S., and Bhattacharya, S. K.: Carbon isotope ratio of dissolved inorganic carbon (DIC) in rivers draining the Deccan Traps, India: Sources of DIC and their magnitudes, Earth Planet. Sc. Lett., 236, 419–429, 2005.
Dickson, A., Sabine, C., and Christian, G.: Guide to best practices for ocean CO2 measurements, North Pacific Marine Science Organization (PICES) Special Publication, 191 pp., 3rd edn., available at: http://cdiac.ess-dive.lbl.gov/ftp/oceans/Handbook_2007/Guide_all_in_one.pdf (last access: 29 June 2018), 2007.
DID: Department of Irrigation and Drainage Sarawak: Resource Centre-IRBM 22 Basins, available at: http://www.did.sarawak.gov.my/modules/web/pages.php?mod=webpage&sub=page&id=315&menu_id=0&sub_id=314 (last access: 29 June 2018), 2017.
Dlugokencky, E. J., Lang, P. M., Mund, J. W., Crotwell, A. M., Crotwell, M. J., and Thoning, K. W.: Atmospheric carbon dioxide dry air mole fractions from the NOAA ESRL Carbon Cycle Cooperative Global Air Sampling Network, 1968–2016, Version: 2017-07-28, available at: ftp://aftp.cmdl.noaa.gov/data/trace_gases/co2/flask/surface, last access: 13 June 2018.
Dommain, R., Couwenberg, J., and Joosten, H.: Development and carbon sequestration of tropical peat domes in south-east Asia: links to post-glacial sea-level changes and Holocene climate variability, Quaternary Sci. Rev., 30, 999–1010, https://doi.org/10.1016/j.quascirev.2011.01.018, 2011.
DWD: German Weather Service: Climate data center, Station ID 96421, available at: ftp://ftp-cdc.dwd.de/pub/CDC/observations_global/CLIMAT/, last access: 27 August 2018.
Gaveau, D. L. A., Sloan, S., Molidena, E., Yaen, H., Sheil, D., Abram, N. K., Ancrenaz, M., Nasi, R., Quinones, M., Wielaard, N., and Meijaard, E.: Four Decades of Forest Persistence, Clearance and Logging on Borneo, PlosOne, 9, e101654, https://doi.org/10.1371/journal.pone.0101654, 2014.
Gaveau, D. L. A., Salim, M., and Arjasakusuma, S.: Deforestation and industrial plantations development in Borneo, Center for International Forestry Research (CIFOR), V2, REGBorneo_PlantedIOPP_1973to2016_CIFOR, https://doi.org/10.17528/CIFOR/DATA.00049, 2016.
Griffith, D. W. T.: Synthetic calibration and quantitative analysis of gas-phase FT-IR spectra, Appl. Spectrosc., 50, 59–70, 1996.
Griffith, D. W. T., Deutscher, N. M., Caldow, C., Kettlewell, G., Riggenbach, M., and Hammer, S.: A Fourier transform infrared trace gas and isotope analyser for atmospheric applications, Atmos. Meas. Tech., 5, 2481–2498, https://doi.org/10.5194/amt-5-2481-2012, 2012.
Harun, S., Dambul, R., Abdullah, M. H., and Mohamed, M.: Spatial and seasonal variations in surface water quality of the Lower Kinabatangan River Catchment, Sabah, Malaysia, J. Tropical Biol. Conserv., 11, 117–131, 2014.
Huang, T. H., Fu, Y. H., Pan, P. Y., and Chen, C. T. A.: Fluvial carbon fluxes in tropical rivers, Curr. Opin. Env. Sust., 4, 162–169, https://doi.org/10.1016/j.cosust.2012.02.004, 2012.
Huang, T. H., Chen, C. T. A, Tseng, H. C., Lou, J. Y., Wang, S. L., Yang, L., Kandasamy, S., Gao, X., Wang, J. T., Aldrian, E., Jacinto, G. S., Anshari, G. Z., Sompongchaiyakul, P., and Wang, B. J.: Riverine carbon fluxes to the South China Sea, J. Geophys. Res.-Biogeo., 122, 1239–1259, https://doi.org/10.1002/2016JG003701, 2017.
Johnson, J. E.: Evaluation of a seawater equilibrator for shipboard analysis of dissolved oceanic trace gases, Anal. Chim. Ac., 395, 119–132, 1999.
Kalnay, E., Kanamitsu, M., Kistler, R., Collins, W., Deaven, D., Gandin, L., Iredell, M., Saha, S., White, G., Woollen, J., Zhu, Y., Chelliah, M., Ebisuzaki, W., Higgins, W., Janowiak, J., Mo, K. C., Ropelewski, C., Wang, J., Leetmaa, A., Reynolds, R. Jenne, R., and Joseph, D.: The NCEP/NCAR 40-year reanalysis project, B. Am. Meteor. Soc., 77, 437–470, 1996.
Keeling, C. D.: The concentration and isotopic abundances of atmospheric carbon dioxide in rural areas, Geochim. Cosmoschim. Ac., 13, 322–334, 1958.
Kumagai, T., Saitoh, T. M., Sato, Y., Takahashi, H., Manfroi, O. J., Morooka, T., Kuraji, K., Suzuki, M., Yasunari, T., and Komatsu, H.: Annual water balance and seasonality of evapotranspiration in a Bornean tropical rainforest, Agr. Forest Meteorol., 128, 81–92, https://doi.org/10.1016/j.agrformet.2004.08.006, 2005.
Lauerwald, R., Laruelle, G. G., Hartmann, J., Ciais, P., and Regnier, P. A. G.: Spatial patterns in CO2 evasion from the global river network, Global Biogeochem. Cy., 29, 1–21, https://doi.org/10.1002/2014GB004941, 2015.
Le, T. P. Q., Dao, V. N., Rochelle-Newall, E., Garnier, J., Lu, X., Billen, G., Duong, T. T., Ho, C. T., Etcheber, H., Nguyen, T. M. H., Nguyen, T. B. N., Nguyen, B. T., Le, N. D., and Pham, Q. L.: Total organic carbon fluxes of the Red River System (Vietnam), Earth Surf. Proc. Land., 42, 1329–1341, https://doi.org/10.1002/esp.4107, 2017.
Le, T. P. Q., Marchand, C., Ho, C. T., Le, N. D., Duong, T. T., Lu, X., Doan, P. K., Nguyen, T. K., Nguyen, T. M. H., and Vu, D. A.: CO2 partial pressure and CO2 emission along the lower Red River (Vietnam), Biogeosciences, 15, 4799–4814, https://doi.org/10.5194/bg-15-4799-2018, 2018.
Lehner, B, Verdin, K., and Jarvis, A.: HydroSHEDS technical documentation, World Wildlife Funds US, Washington, DC, 1st edn., available at: https://hydrosheds.cr.usgs.gov/webappcontent/HydroSHEDS_TechDoc_v10.pdf (last access: 23 December 2018), 2006.
Lewis, E. and Wallace, D. W. R.: Program Developed for CO2 System Calculations, ORNL/CDIAC-105, Carbon Dioxide Information Analysis Center, Oak Ridge National Laboratory, U.S. Department of Energy, Oak Ridge, Tennessee, 1998.
Li, S., Lu, X. X., and Bush, R. T.: CO2 partial pressure and CO2 emission in the Lower Mekong River, J. Hydrol., 504, 40–56, https://doi.org/10.1016/j.jhydrol.2013.09.024, 2013.
Ling, T.-Y., Soo, C.-L., Sivalingam, J.-R., Nyanti, L., Sim, S.-F., and Grinang, J.: Assessment of the water and sediment quality of tropical forest streams in upper reaches of the Baleh River, Sarawak, Malaysia, subjected to logging activities, J. Chem., 2016, 8503931, https://doi.org/10.1155/2016/8503931, 2016.
Ling, T.-Y., Soo, C.-L., Phan, T.-P., Nyanti, L., Sim, S.-F., and Grinang, J.: Assessment of Water Quality of Batang Rajang at Pelagus Area, Sarawak, Malaysia, Sains. Malays., 46, 401–411, https://doi.org/10.17576/jsm-2017-4603-07, 2017.
Ling Lee, H., Koh, H. L. and Al'Rabia'ah, H. A.: Predicting soil loss from logging in Malaysia, GIS and Remote Sensing in Hydrology, Water Resources and Environment, Proceedings of ICGRHWE held at the Three Gorges Dam, China, September 2003, IAHS Publ. 289, 2004.
MacKinnon, K.: The ecology of Kalimantan, in: The ecology of Indonesia series, vol. 3, Oxford University Press, Oxford 1996.
Martin, P., Cherukuru, N., Tan, A. S. Y., Sanwlani, N., Mujahid, A., and Müller, M.: Distribution and cycling of terrigenous dissolved organic carbon in peatland-draining rivers and coastal waters of Sarawak, Borneo, Biogeosciences, 15, 6847–6865, https://doi.org/10.5194/bg-15-6847-2018, 2018.
Melling, L., Hatano, R., and Goh, K. J.: Methane fluxes from three ecosystems in tropical peatland of Sarawak, Malaysia, Soil Biol. Biochem. 37, 1445–1453, https://doi.org/10.1016/j.soilbio.2005.01.001, 2005.
Milliman, J. D. and Farnsworth, K. L.: River Discharge to the Coastal Ocean: A Global Synthesis, Cambridge University Press, March 2011.
Moore, S., Gauci, V., Evans, C. D., and Page, S. E.: Fluvial organic carbon losses from a Bornean blackwater river, Biogeosciences, 8, 901–909, https://doi.org/10.5194/bg-8-901-2011, 2011.
Moore, S., Evans, C. D., Page, S. E., Garnett, M. H., Jones, T. G., Freeman, C., Hooijer, A., Wiltshire, A. J., Limin, S. H., and Gauci, V.: Deep instability of deforested tropical peatlands revealed by fluvial organic carbon fluxes, Nature, 493, 660–663, https://doi.org/10.1038/nature11818, 2013.
Müller, D., Warneke, T., Rixen, T., Müller, M., Jamahari, S., Denis, N., Mujahid, A., and Notholt, J.: Lateral carbon fluxes and CO2 outgassing from a tropical peat-draining river, Biogeosciences, 12, 5967–5979, https://doi.org/10.5194/bg-12-5967-2015, 2015.
Müller, D., Warneke, T., Rixen, T., Müller, M., Mujahid, A., Bange, H. W., and Notholt, J.: Fate of terrestrial organic carbon and associated CO2 and CO emissions from two Southeast Asian estuaries, Biogeosciences, 13, 691–705, https://doi.org/10.5194/bg-13-691-2016, 2016.
Nachtergaele, F., van Velthuizen, H., and Verelst, L.: Harmonized World Soil Database, available at: http://www.fao.org/docrep/018/aq361e/aq361e.pdf (last access: 29 June 2018), 2009.
Ni, H.-G., Lu, F.-H., Luo, X.-L., Tian, H.-Y., and Zeng, E. Y.: Riverine inputs of total organic carbon and suspended particulate matter from the Pearl River Delta to the coastal ocean off South China, Mar. Pollut. Bull., 56, 1150–1157, https://doi.org/10.1016/j.marpolbul.2008.02.030, 2008.
Nightingale, P. D., Malin, G., Law, C. S., Watson, A. J., Liss, P. S., Liddicoat, M. I., Boutin, J., and Upstill-Goddard, R. C.: In situ evaluation of air-sea gas exchange parameterizations using novel conservative and volatile tracers, Global Biogeochem. Cy., 14, 373–387, 2000.
Oakes, J. M., Eyre, B. D., Ross, D. J. and Turner, S. D.: Stable isotopes trace estuarine transformations of carbon and nitrogen from primary- and secondary-treated paper and pulp mill effluent, Environ. Sci. Technol., 44, 7411–7417, https://doi.org/10.1021/es101789v, 2010.
Oliveira, A. P., Cabecadas, G., and Mateus, M. D.: Inorganic carbon distribution and CO2 fluxes in a large European estuary (Tagus, Portugal), Nature Sci. Rep., 7, 7376, https://doi.org/10.1038/s41598-017-06758-z, 2017.
Page, S. E., Rieley, J. O., and Banks, C. J.: Global and regional importance of the tropical peatland carbon pool, Glob. Change Biol., 17, 798–818, https://doi.org/10.1111/j.1365-2486.2010.02279.x, 2011.
Park, J.-H., Nayna, O. K., Begum, M. S., Chea, E., Hartmann, J., Keil, R. G., Kumar, S., Lu, X., Ran, L., Richey, J. E., Sarma, V. V. S. S., Tareq, S. M., Xuan, D. T., and Yu, R.: Reviews and syntheses: Anthropogenic perturbations to carbon fluxes in Asian river systems – concepts, emerging trends, and research challenges, Biogeosciences, 15, 3049–3069, https://doi.org/10.5194/bg-15-3049-2018, 2018.
Pataki, D. E., Ehleringer, J. R., Flanagan, L. B., Yakir, D., Bowling, D. R., Still, C. J., Buchmann, N., Kaplan, J. O., and Berry, J. A.: The application and interpretation of Keeling plots in terrestrial carbon cycle research, Global Biogeochm. Cy., 17, 1022, https://doi.org/10.1029/2001GB001850, 2003.
Polsenaere, P. and Abril, G.: Modelling CO2 degassing from small acidic rivers using water pCO2, DIC and δ13C-DIC data, Geochim. Cosmochim. Ac., 91, 220–239, https://doi.org/10.1016/j.gca.2012.05.030, 2012.
Raymond, P. A. and Cole, J. J.: Gas exchange in rivers and estuaries: Choosing a gas transfer velocity, Estuaries, 24, 312–317, 2001.
Raymond, P. A., Zappa, C. J., Butman, D., Bott, T. L., Potter, J., Mulholland, P., Laursen, A. E., McDowell, W. H., and Newbold, D.: Scaling the gas transfer velocity and hydraulic geometry in streams and small rivers, Limnol. Oceanogr., 2, 41–53, https://doi.org/10.1215/21573689-1597669, 2012.
Raymond, P. A., Hartmann, J., Lauerwald, R., Sobek, S., McDonald, C., Hoover, M., Butman, D., Striegl, R., Mayorga, E., Humborg, C., Kortelainen, P., Dürr, H., Meybeck, M., Ciais, P., and Guth, P.: Global carbon dioxide emissions from inland waters, Nature, 503, 355–359, https://doi.org/10.1038/nature12760, 2013.
Rixen, T., Baum, A., Wit, F., and Samiaji, J.: Carbon leaching from tropical peat soils and consequences for carbon balances, Front. Earth Sci., 4, 74, https://doi.org/10.3389/feart.2016.00074, 2016.
Rosentreter, J. A., Maher, D. T., Erler, D. V., Murray, R., and Eyre, B. D.: Factors controlling seasonal CO2 and CH4 emissions in three tropical mangrove-dominated estuaries in Australia, Estuar. Coast. Shelf S., in review, 2018.
Rózanski, K., Froehlich, K., and Mook, W. G.: Environmental Isotopes in the Hydrological Cycle, Principles and Applications, vol. 3, Surface Water, International Atomic Energy Agency and United Nations Educational, Scientific and Cultural Organization, Krakow/Vienna/Groningen, 2003.
Sa'adi, Z., Shahid, S., Ismail, T., Chung, E.-S., and Wang, X.-J.: Distributional changes in rainfall and river flow in Sarawak, Malaysia, Asia-Pac, J. Atmos. Sci., 53, 489–500, https://doi.org/10.1007/s13143-017-0051-2, 2017.
Sarawak Energy: About Hydropower, available at: http://www.sarawakenergy.com.my/index.php/hydroelectric-projects (last access: 29 June 2018), 2013.
Scofield, V., Melack, J. M., Barbosa, P. M., Amaral, J. H. F., Forsberg, B. R., and Farjalla, V. F.: Carbon dioxide outgassing from Amazonian aquatic ecosystems in the Negro River basin, Biogeochem., 129, 77–91, https://doi.org/10.1007/s10533-016-0220-x, 2016.
Snoussi, M., Haida, S., and Imassi, S.: Effects of the construction of dams on the water and sediment fluxes of the Moulouya and the Sebou Rivers, Morocco, Reg. Environ. Change, 3, 5–12, https://doi.org/10.1007/s10113-001-0035-7, 2002.
Staub, J. R. and Esterle, J. S.: Provenance and sediment dispersal in the Rajang River delta/coastal plain system, Sarawak, East Malaysia, Sedimentary Geology, 85, 191–201, 1993.
Staub, J. R. and Gastaldo, R. A.: Late Quarternary Sedimentation and peat development in the Rajang River Delta, Sarawak, East Malaysia, in: Tropical Deltas of Southeast Asia – Sedimentology, Stratigraphy, and Petroleum Geology, edited by: Hasan Sidi, F., Nummedal, D., Imbert, P., Darman, H., and Posamentier, H. W., SEPM Special Publication No. 76, Tulsa, Oklahoma, USA, 71–87, September 2003.
Staub, J. R., Among, H. L., and Gastaldo, R. A.: Seasonal sediment transport and deposition in the Rajang River delta, Sarawak, East Malaysia, Sediment Geol., 133, 249–264, 2000.
Teodoru, C. R., Nyoni, F. C., Borges, A. V., Darchambeau, F., Nyambe, I., and Bouillon, S.: Dynamics of greenhouse gases (CO2, CH4, N2O) along the Zambezi River and major tributaries, and their importance in the riverine carbon budget, Biogeosciences, 12, 2431–2453, https://doi.org/10.5194/bg-12-2431-2015, 2015.
Thirumalai, K., DiNezio, P. N., Okumura, Y, and Deser, C.: Extreme temperatures in Southeast Asia caused by El Nino and worsened by global warming, Nat. Commun., 8, 15531, https://doi.org/10.1038/ncomms15531, 2017.
Van Asperen, H., Warneke, T., Sabbatini, S., Höpker, M., Nicolini, G., Chiti, T., Papale, D., Böhm, M., and Notholt, J.: Diel variation in isotopic composition of soil respiratory CO2 fluxes: The role of non-steady state conditions, Agr. Forest Meteorol., 234–235, 95–105, https://doi.org/10.1016/j.agrformet.2016.12.014, 2017.
Venkiteswaran, J. J., Schiff, S. L., and Wallin, M. B.: Large carbon dioxide fluxes from headwater boreal and sub-boreal streams, PLOS one, 9, e101756, https://doi.org/10.1371/journal.pone.0101756, 2014.
Vörösmarty, C. J., Meybeck, M., Fekete, B., Sharma, K., Green, P., and Syvitski, J. P. M.: Anthropogenic sediment retention: major global impact from registered river impoundments, Global Planet. Change, 39, 169–190, 2003.
Wanninkhof, R.: Relationship between wind speed and gas exchange over the ocean, J. Geophys. Res., 97, 7373–7382, 1992
Weiss, R.: Carbon dioxide in water and seawater: The solubility of a non-ideal gas, Mar. Chem., 2, 203–215, 1974.
Wit, F.: Carbon footprints of peatland degradation, PhD Thesis, University of Bremen, Bremen, Germany, 2017.
Wit, F., Müller, D., Baum, A., Warneke, T., Setiyo Pranowo, W., Müller, M., and Rixen, T.: The impact of disturbed peatlands on river outgassing in Southeast Asia, Nat. Commun., 6, 10155, https://doi.org/10.1038/ncomms10155, 2015.
WTW GmbH: FDO 925 and FDO 925-P Operating Manual, Weilheim, Germany, 11/2012.
Yao, G., Gao, Q., Wang, Z., Huang, X., He, T., Zhang, Y., Jiao, S. and Ding, J.: Dynamics of CO2 partial pressure and CO2 outgassing in the lower reaches of the Xijiang River, a subtropical monsoon river in China, Sci. Total Environ., 376, 255–266, https://doi.org/10.1016/j.scitotenv.2007.01.080, 2007.