the Creative Commons Attribution 4.0 License.
the Creative Commons Attribution 4.0 License.
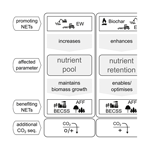
Ideas and perspectives: Synergies from co-deployment of negative emission technologies
Jens Hartmann
Numerous publications propose the deployment of negative emission technologies, which intend to actively remove CO2 from the atmosphere with the goal to reach the 1.5∘ target as discussed by the IPCC. The increasing number of scientific studies on the individual potential of different envisaged technologies and methods indicates that no single method has enough capacities to mitigate the issue by itself. It is thus expected that technology portfolios are deployed. As some of them utilize the same environmental compartment, co-deployment effects are expected. Those effects are particularly important to evaluate with respect to additional CO2 uptake. Considering soils as one of the main affected compartments, we see a plethora of processes which can positively benefit from each other, canceling out negative side effects or increasing overall CO2 sequestration potentials. To derive more reliable estimates of negative emission potentials and to evaluate common effects on global carbon pools, it is now necessary to intensively study interrelated effects of negative emission technology deployment while minimizing negative side effects.
- Article
(942 KB) -
Supplement
(9997 KB) - BibTeX
- EndNote
As global mean temperatures are projected to increase further, strategies to mitigate climate change in time by decreasing CO2 emissions seem to slowly take effect (Jackson et al., 2015). Some CO2 emission pathways include negative carbon emission strategies (Fuss et al., 2014, 2016; Rogelj et al., 2018) that essentially capture CO2 from the atmosphere in different ways, storing them in the long term as CO2 molecules, or as organic and inorganic compounds (Caldeira et al., 2013). All discussed options and technologies have yet to reach the large-scale deployment stage (Minx et al., 2018; Nemet et al., 2018). Most technologies are immature, lacking deep research on the global potential, technical feasibility, economics of deployment, and especially an assessment of the expected side effects (National Research Council, 2015; Fuss et al., 2018).
The proposed negative emission technologies (NETs) encompass highly technical engineering solutions as well as methods that rely on natural processes, like growth of biomass (e.g., bioenergy with carbon capture and storage (BECCS), and afforestation), soil carbon increase, biochar, and chemical weathering (e.g., Enhanced Weathering (EW) and ocean liming). As these methods are aimed to be integrated in global biogeochemical cycles and will redistribute carbon between reservoirs (Keller et al., 2018), their interaction is inevitable if NETs are deployed at the largest scale. As such, it must be assessed how the co-deployment of NETs will affect the individual and overall efficiency since until now publications have focussed generally on single NETs, disregarding any effects on concurrent deployment of additional technologies.
Findings from NET-specific literature suggest that assessing the effects of combined NET rollout is advisable and future research should include CO2-sequestration-enhancing side effects that could increase the overall potential of NETs. However, the principal interaction between proposed methods needs to be studied in detail beforehand to understand effects on the carbon pools (Fig. 1).
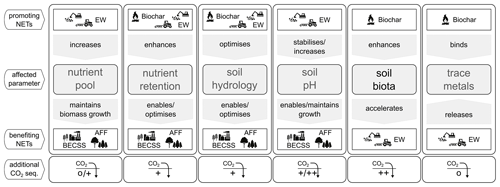
Figure 1Overview of effects from combining land-based negative emission technologies (bioenergy production coupled with carbon capture and storage, BECCS; afforestation, AFF; Enhanced Weathering, EW; and biochar). The additional CO2 sequestration is a qualitative estimate based on the author's personal assessment. Technology symbols courtesy of William Lamb (MCC Berlin).
While biomass-based NETs like afforestation and BECCS are widely discussed, EW is underrepresented in this discussion (Minx et al., 2018). EW relies on the uptake of CO2 via dissolution of minerals based on the natural process of chemical weathering. EW is facilitated by the application of finely ground rock on (agricultural) land, preferably in areas with elevated temperatures and rainfall. The resources for this NET have to be mined and, depending on the type and scale of rollout, the extraction of material can result in the creation of extensive mining areas. However, under a sustainable approach, affected environments could later be used to create biodiversity hotspots (e.g., Tropek et al., 2010; Benes et al., 2003).
It is unavoidable that the intended CO2 sequestration effect by weathering is naturally accompanied by the release of elements with consequences for the environment (Kantola et al., 2017) and consequently the involved carbon pools. The release of elements that are important plant nutrients (e.g., potassium, phosphorus, magnesium) can be beneficial for additional CO2 sequestration via organic carbon formation. In addition, the soil hydrology can be improved, and cation exchange capacity increased under optimal grain size distribution and mineral selection. In contrast, effects of potentially harmful trace element release (by choosing less suitable material) might need to be alleviated. However, an integrated framework to achieve optimization of interrelated effects between land-based NETs has yet to be developed, specifically for the global-scale management of carbon pools.
To tackle the issue of climate change with negative carbon emission strategies on a global and comprehensive scale, it seems advisable to consider all proposed terrestrial biomass-based NETs, like BECSS, afforestation, and biochar, to explore synergistic effects (Fig. 1). A scenario can be envisioned, in which rock powder and biochar are applied to agricultural land, which is used for bioenergy plant production (for further use in BECSS technology). Rock material would release geogenic nutrients and biochar could enhance the release of nutrients (Atkinson et al., 2010) and the overall crop productivity (Jeffery et al., 2011).
In combination with envisioned and deployed afforestation efforts, which often take place in tropical areas with depleted soils (Nilsson and Schopfhauser, 1995; Grainger, 1988; Zomer et al., 2008), rock powder deployment for EW could be an added, if not essential, benefit. The low capacity of these soils to retain highly soluble industrial fertilizers suggests the use of other forms of slow release fertilizer, like rock dust as a complement (Leonardos et al., 1987; Manning, 2015), or new emerging rock-based fertilizers (Ciceri and Allanore, 2019), which can, as a side effect, increase the retention of industrial fertilizers, which may still be needed. The ultimate need for an intense management and design of a suitable soil to supply suitable conditions for tree growth can be deduced from a published extreme scenario, which envisions large-scale afforestation of deserts (Ornstein et al., 2009).
It seems advisable to combine proposed NET methods to achieve an optimal carbon pool management for negative emissions and ensure food security over centuries at the global scale. To achieve this, interdisciplinary efforts are necessary (Fig. 1) and some of the key issues are reviewed here to point out the future research directions.
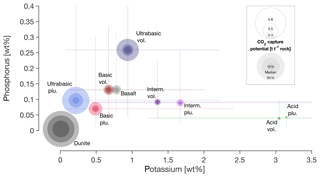
Figure 2The averaged relative K and P contents of igneous rocks (middle point: median; whiskers: 10th and 90th percentiles, some cutoff at extreme ends for better graphic representation), classified by SiO2 content (ultrabasic: < 45 %; basic: 45 %–52 %; intermediate: 52 %–63 %; acid: >63 %). The circles indicate their potential to capture CO2. Statistical data are from the GEOROC database (Sarbas, 2008), details in Sect. S3. Documentation on CO2 capture potential calculation in Sect. S4. A map with the global distribution of all classes is available in Sect. S5. Basalt and dunite were added separately as reference for commonly discussed rock types.
Increasing atmospheric CO2 concentrations and an increasing world population will lead to challenges in the nutritional supply for large parts of Earth's population (Smith and Myers, 2018; Myers et al., 2014). In combination with partly declining resources of natural mineral fertilizers (Manning, 2015, and Sect. S1 in the Supplement), alternative nutrient supplies, i.e., from rock products, are of high interest (Ciceri and Allanore, 2019). This idea has been discussed earlier (van Straaten, 2006, 2002) and was recently revived in the context of EW (Beerling et al., 2018; Hartmann et al., 2013). However, this issue extends further, if biomass-based NETs are considered for large-scale deployment. While nutrients like P or K are normally supplied via mineral dissolution in natural systems, nitrogen is in general supplied via fixation of N from the atmosphere (Graham and Vance, 2000). In some ecosystems N supply via rocks might be a relevant source (Houlton et al., 2018; Holloway and Dahlgren, 2002). In general, and specifically under intensified demand scenarios created by enhanced biomass growth rock, rock N supply will not keep up with the demand.
Many options of carbon dioxide removal rely on the production of biomass (i.e., biochar, afforestation, carbon capture and storage from bioenergy (BECCS), biofuels). These carbon dioxide removal (CDR) methods demand, if driven to an optimum, more geogenic nutrients than typically available to plants from the soil–rock systems in the long term, specifically in humid, tropical areas, where soils are deeply weathered and show naturally low nutrient contents (Hamdan and Bumham, 1996) that could not supply an additional intense biomass growth. A study on commercially exploited forests in the US points out that intensive harvest can withdraw more nutrients from the soils than can naturally be resupplied (de Oliveira Garcia et al., 2018).
The intensive withdrawal of nutrients should be included in a framework for biogeochemical cycle management under NET deployment. The withdrawal of K and P from cropland amounts globally to more than 8 Mt a−1 each (Fig. S2-2). For many ecosystems the natural resupply and potentially limiting effects under absence of deliberate fertilization practices are unknown or merely based on meta-analyses or model studies.
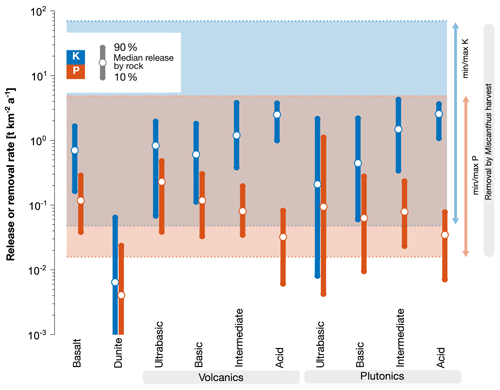
Figure 3Weathering release rates (circles; bars as variability indicator) of P and K from selected rocks (assuming their full dissolution under a natural subtropical weathering scenario) and extraction of those nutrients by harvesting Miscanthus energy grass (blue and red areas indicate range between min. and max. nutrient content of different Miscanthus species multiplied by min. and max. yield reported in Brosse et al. (2012), Tables S2-1 and S2-2). Details on rock dissolution and nutrient release rates in Sect. S6 and on plant nutrient removal and additional Miscanthus data on major crops in Sect. S2. Dunite values for the 10th percentile were cut off for better overall visibility.
Due to desired global carbon sequestration goals (as in models for afforestation), growth rates will likely be driven to the maximum, which implies an increased demand of nutrients. Models show that N and P limit the global carbon sequestration potential for forests (Goll et al., 2012; Kracher, 2017). Nutrient release by EW can therefore play a relevant role in supporting the high demand. Particular rock classes contain, on average, higher K, P (Fig. 3), or micronutrients like Zn or Se, than others. To ensure a balanced supply of the needed elements, it is therefore necessary to consider more than one specific rock type during EW application.
Considering a subtropical weathering scenario in combination with Miscanthus growth for BECCS, acid igneous rocks show a high potential to (partly) resupply extracted potassium, while (ultra-)basic rocks can (partly) resupply phosphorus (Fig. 3). Many earlier studies on EW focussed on dunite to maximize inorganic CO2 sequestration, with the side effect of adding high levels of Ni and Cr to the system (e.g., Schuiling and Krijgsman, 2006; Hangx and Spiers, 2009). Later, basalt was added to the discussion (Beerling et al., 2018; Strefler et al., 2018; Hartmann et al., 2013). It is characterized by an elevated geogenic nutrient supply compared to ultrabasic rocks like dunite (Fig. 3), but still features a sufficiently high inorganic CO2 sequestration potential (Fig. 2, and Strefler et al., 2018). Future application scenarios will likely use a mixture of locally available material to optimize both organic and inorganic carbon storage. Optimizing the nutrient composition may come at the price of reducing the inorganic carbon sequestration potential, as some rock types with high nutrient content have low sequestration potentials (Fig. 2). If additional soil properties, like cation exchange capacity, water content/hydrology, and pH, are optimized, this reduction of inorganic carbon sequestration may be compensated for by elevated biomass uptake and organic carbon storage.
The introduction of additional nutrients to the soil system will not necessarily lead to an additional CO2 uptake and increased CO2 sequestration potentials of biomass-based NETs, if enough nutrients are supplied by traditional fertilization. However, forest areas may benefit from slow-release nutrients available long term as they may be less easy to resupply on a regular basis by agrotechnical machinery. Also, industrial fertilizer may be unaffordable in low-income regions; thus rock products could replace parts of the fertilizer (Ciceri and Allanore, 2019). A wider adoption of rock product utilization may also lead to the development of new and optimized application techniques.
Nutrients released from industrial fertilizers or from natural rock products can be taken up by the plant, washed away, or retained by the properties of the soil. The latter is called retention capacity and is important to store nutrients in a plant-available form. It has been shown that the weathering of basaltic material increases the cation exchange capacity, leading to an increased retention of nutrients (Anda et al., 2013, 2015). This is especially important for areas in which nutrients from industrial fertilizer material are quickly washed out, e.g., from the deeply weathered soils (e.g., Oxisols) in tropical regions (Leonardos et al., 1987; Ciceri et al., 2017). In such settings, it will be favorable to establish improved soil conditions with optimized nutrient retention.
Another application case is the fertilization of forests, specifically in areas which are reforested after agricultural use. With increasing atmospheric CO2 concentrations, an increase in biomass productivity in nonagricultural areas is expected through the CO2 fertilization effect (e.g., Ciais et al., 1995; Körner et al., 2007; Norby and Zak, 2011), especially with regard to afforestation efforts and general tree growth. This effect has yet to be clearly shown (Leuzinger et al., 2011), and is likely limited by soil fertility (Oren et al., 2001; Bader et al., 2013). It can already be observed that nutrient supply by rock weathering, specifically P, K, Mg, and Ca, can be the limiting factor of tree growth under elevated atmospheric CO2 (Jonard et al., 2015). Woodland soils might be amended with selected minerals or rocks to supply sufficient nutrients to keep up growth under elevated atmospheric pCO2 conditions and organically bind carbon, a scenario that should be explored further for its potential to enlarge affected carbon pools. At some point, depending on the environmental setting, biomass growth will be limited by nutrient supply and as such, model outputs for CO2 sequestration potentials of afforestation are likely to be overestimated, if geogenic nutrient cycles are not included in the assessment, as Goll et al. (2012) have shown, for example, for the C, N, and P cycles using a model.
The CO2 sequestration effect of afforestation is even larger if soil organic carbon changes are taken into consideration: depending on the underlying lithology, the organic carbon pools can be increased (Li et al., 2017), a process that may be optimized by the spreading of selected rock products.
Overall, specific element deficits in soils need to be mapped, since they can also affect the plant content of valuable, if not essential, elements for human nutrition (Zhang et al., 2017; Hengl et al., 2017; White and Zasoski, 1999). It is necessary to be able to predict which application amounts of elements causes a certain response in the biomass pool above and below ground. Such data are still scarce and inconclusive (Manning, 2010) but are needed if EW should be used as a method to help manage carbon and nutrient pools.
Biochar is another NET that has a beneficial effect on the retention of nutrients (Fig. 1). Due to its large surface area and increased cation exchange capacity, nutrients can be sustainably retained in soils (Lehmann, 2007; Liang et al., 2006), effectively saving applied fertilizer (Laird et al., 2010).
Increased nutrient retention may increase the overall CO2 sequestration potential of biomass-based NETs through the long-term availability of nutrients. However, the order of magnitude of the effect remains to be shown.
The availability of water is essential for high crop yields (Rockstrom et al., 2007), and soil hydraulic properties fundamentally steer the availability of water to plants (Bodner et al., 2015; Pinheiro et al., 2019). The soil hydraulic conductivity is a measure of how easily water can percolate though the soil column. It depends largely on the grain size distribution of the soil. Roughly, coarse (sandy) soils have a higher hydraulic conductivity than fine (clayey) soils (Rawls et al., 1982). Spreading large amounts of rock products with very small grain sizes (silty to clayey) on land potentially leads to a decrease in soil hydraulic conductivity, which may lead to decreased weathering speeds due to local pore water oversaturation or enhanced surface runoff. However, there are some indications that the addition of biochar can be used to control hydraulic conductivity (Masiello et al., 2015; Barnes et al., 2014), which could enable the use of smaller grain sizes for EW, enhancing its potential, which strongly depends on the grain size (Strefler et al., 2018).
As another hydraulic property, the water holding capacity determines how much water is kept in the soils and potentially is available to plants. This parameter becomes increasingly important with more frequently appearing droughts due to climate change (Kang et al., 2009). Biochar could be used to improve the water holding capacities of soils (Omondi et al., 2016; Liu et al., 2017), and also increase the plant available water in some cases (Masiello et al., 2015). This may render dryer regions or areas with unfavorable soil physical properties (Basso et al., 2013) usable for bioenergy plants and/or afforestation. There are also indications that improvement of soil hydrology by biochar may increase yield potentials (Akhtar et al., 2014; Xu et al., 2015; Al-Wabel et al., 2018).
It is important to point out that all potential changes in soil physical properties due to biochar application strongly depend on its type, more specifically the feedstock and production temperature (Gul et al., 2015). The combination of rock product and biochar application, however, was not addressed in previous research at all but may provide relevant potential to increase and maintain soil carbon.
Soil pH steers the availability of elements to plants (Kabata-Pendias, 2010; Loomis and Morris, 1983). At pH values well below 7, nutrients become less available to plants and potentially harmful trace metals are successively mobilized. Nitrogen-fixing bacteria are also depending on a specific pH (Graham and Vance, 2000). Soil acidification on heavily used cropland is a problem (Helyar and Porter, 1989), which may lead to a decrease in crop yields. The main reason is the higher mobility of most exchangeable metals at low pH, which decreases logarithmically with increasing pH (Kabata-Pendias, 2010; Robinson et al., 1996; Tack et al., 1996; Harter, 1983). Levels of pH 6 and higher generally ensure very low levels of exchangeable harmful metals, with the exception of arsenic, depending on the oxidation state (Dixit and Hering, 2003). The release of base cations from rock flour leads to a soil pH increase. Studies have demonstrated the effectiveness of basalt powder application in raising the soil pH up to 8 and higher (e.g., Gillman et al., 2001; Nunes et al., 2014). The effect is similar to agricultural liming, which is a common practice to counteract soil acidification on cropland (West and McBride, 2005). Despite the fast dissolution rate of carbonate minerals, they have in general, until today, not been considered for EW scenarios, because of possible carbonate precipitation and subsequent CO2 release in the ocean (Hartmann et al., 2013) or due to the potential release of CO2 by excess fertilizer application (Semhi et al., 2000; Perrin et al., 2008). The potential of carbonates in EW strategies remains to be studied, while silicate application is the focus of recent research (Taylor et al., 2015). It could be a potential economic benefit to replace agricultural lime with silicate rock flour, bearing in mind that silicate dissolution rates are in general several orders of magnitude lower, with strong variability between different minerals (Lasaga, 1995; Brantley et al., 2008). Thus, the efficacy is decreased due to the slower release rate of cations, but other properties like nutrient retention or soil hydrology might be improved (see Sect. 4). It remains to be investigated how (fast) the termination of pH-stabilizing silicate rock powder application will affect the soils. If relatively immobile potentially harmful metals accumulate at elevated pH values over the application period, an excessive and harmful release of toxic substances might occur in case of a future drop of pH due to changes in pH-controlling minerals, land use, or general environmental conditions. Once the deployment of material rich in trace elements of concern is started, it is obligatory to maintain a stabilized pH environment, strengthening the need for material with low harmful trace element concentrations (requirements may differ depending on ecosystem type).
Assuming that pH stabilization and beneficial changes in soil hydrology (see previous section) are achievable with biochar and EW, a significant additional CO2 uptake can be expected, based on the fact that soils that could not support sustainable biomass growth before are made usable for biomass-based NETs.
Chemical weathering of rocks can be significantly mediated by macro- and microbiota (Schwartzman and Volk, 1989; Uroz et al., 2009; Hoffland et al., 2004; Blouin et al., 2013), although the order of magnitude is a matter of debate (Drever, 1994). This is specifically the case for mycorrhizal fungi and microbes, which create physicochemical conditions that accelerate the dissolution of minerals (Taylor et al., 2015). The weatherability of minerals depends on the type of bioinoculant (Nishanth and Biswas, 2008; Benzerara et al., 2005; Cuadros, 2018), implicating that a supervision and management of the soil microbiota is necessary to optimize both crop yields and rock weathering. Microbial populations in soils respond to the addition of biochar (Warnock et al., 2007) by providing a refuge for bacteria and fungi (Pietikainen et al., 2000; Saito, 1990), increasing nutrient availability, creating favorable pH conditions, and other processes discussed in Lehmann et al. (2011).
Earthworms have been observed to thrive in biochar-amended soils (Topoliantz and Ponge, 2005). Increased abundance of earthworms will likely increase bioturbation effects (Carcaillet, 2001; Major et al., 2010), leading to a better distribution of biochar and rock flour in deeper layers of the amended soils, increasing reactive surfaces of mineral grains. Bioturbation might also be a key process to achieve high CO2 sequestration rates by weathering, as earthworms can enhance mineral weathering (Carpenter et al., 2007, 2008) and contribute to the downward transport of added rock products into deeper soil layers (Taylor et al., 2015).
Soils are an important sink in the environmental cycling of trace metals (Kabata-Pendias, 1993). In addition to naturally occurring concentrations, depending on the underlying lithology, the major source of trace metals to soils is agricultural practice, leading to an enrichment due to the application of manure, sewage sludge, fertilizers, and pesticides, which all contain metals to a certain extent (Gonnelli and Renella, 2013). Field studies using sewage sludge as fertilizer have shown a marked uptake by the crops and increased mobilization of trace metals in the runoff water (Alloway, 2013). Adding to the anthropogenic input, the introduction of additional rock products with elevated levels of trace metals (Fig. 4) could lead to a critical exceedance of environmental thresholds if improper rock material is used due to inconsiderate management. This, however, relates to the solubility of minerals within the used rock type and the redox and pH conditions. An EW soil incubation experiment using an olivine-rich rock product, with elevated Ni and Cr concentrations in the source material (Fig. 4), showed only a few occurrences of elevated Cr levels but no Ni increase in the aquatic solution compared to a blank treatment, leading to the conclusion that the soil solid phase will be successively enriched in those elements (Renforth et al., 2015).
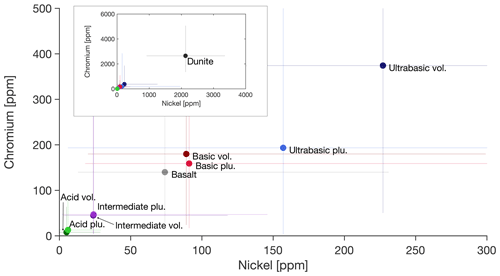
Figure 4Contents of Ni and Cr in igneous rocks, classified by SiO2 content (see Fig. 2). Circles indicate median values; whiskers are the 10th and 90th percentiles. Extreme values of percentiles were cut off for better visibility of data. The inset shows a visualization of dunite as it features extremely high values. For detailed statistics, see Sect. S7.
The availability of heavy metals to biota remains an issue of ongoing discussion (Nagajyoti et al., 2010). The main elements of concern in source rocks with the highest sequestration potential (ultramafic rocks) are Ni and Cr. In particular the earlier discussed dunite application for EW must trigger discussion about its high Cr and Ni contents (Fig. 4) and is therefore ruled out for large-scale application on cropland.
If an application with rocks of high trace metal concentrations of concern is considered, it is necessary to stabilize the soil pH even after cessation of such actions in order to maintain the fixation of toxic elements because of the strong pH control on metal mobility. A study of long-term sewage sludge application has shown that the pH had to be stabilized by liming in order to prevent phytotoxicity of Cu and Zn (McBride et al., 2004). Additionally, the metal availability to plants has been shown to be influenced by the soil texture, with marked differences for different elements (Qian et al., 1996). This underlines the necessity to control or specifically design the grain size distribution of the soil to control water content, pH, and oxygen content. To further ameliorate the issue, biochar, which has been shown to immobilize heavy metals in soils, depending on feedstock and production conditions (Ahmad et al., 2014; Beesley et al., 2011), could be jointly applied with rock powder. This would mean that potential limitations of fertilizer or rock spreading due to thresholds put in place for environmental protection could be overcome by a sensible management of biochar utilization. Applying biochar products does not remove elements of concern, but the problem of heavy metal accumulation could be dampened by bioremediation through heavy-metal-accumulating plants (Rajkumar et al., 2012). This in turn could be a potential new source of raw material for industrial use (Schuiling, 2013), though it is likely not applicable on a global scale since this would compete against food and energy plant production, which is already an issue (Tilman et al., 2009).
The alleviation of trace metal effects does not directly affect CO2 sequestration rates but could overall increase potential deployment areas for EW.
Looking forward it is likely that a portfolio of options will be established to optimize the sequestration effect and minimize negative impacts. The combination of previously separately studied NETs to increase the sequestered carbon pool should consider the management of biogeochemical cycles and optimize the combined application of Enhanced Weathering and biochar in the context of biomass-based methods like BECCS and afforestation to maximize carbon capture as well as food production. It is therefore essential to address combined effects of NET co-deployment in future research projects.
As all presented interactions take place in the soil, future research should put a focus on creating an optimized soil product for optimal long-term sustainable carbon management. We propose that research around biomass-based NET interactions becomes the science of artificial soil products, which are most likely created on depleted and degraded soils, especially in the (sub)tropics. It may consist of the locally available “base soil” mixed with biochar products to enhance hydraulic properties and nutrient retention, as well as rock powder, which raises the soil pH, provides nutrients, and sequesters CO2 at the same time. This engineered and managed soil could increase carbon pools and crop production, while contributing to tackle the issue of climate change. It remains to be studied where suitable material is available at the regional scale (Sect. S5). The parameterization of element release rates permitting a sustainable management is still subject to large uncertainties and the effects of massive rock product spreading will change the soil structure to an extent that remains to be explored.
The introduction of non-authigenic material into the environment, even if of bio- or geogenic origin, will increase the entropy of the system, making it difficult and expensive (from the viewpoint of energy and economics) to quickly revert back into the “undisturbed” state once large-scale deployment has started. Thus, the continuous deployment of NETs at the global scale at an order of magnitude that would measurably impact atmospheric CO2 levels must be seriously weighed. However, the high probability of NET adoption in the near future makes it imperative to create efficient cooperation networks across all involved disciplines in order to conceive the necessary knowledge on actual CO2 sequestration potentials and century-scale global carbon pool changes.
No unique data were created. All dataset sources used were referenced. All resulting data, underlying the figures, are provided in the Supplement.
The supplement related to this article is available online at: https://doi.org/10.5194/bg-16-2949-2019-supplement.
This article was conceived by the joint work of JH and TA. Both participated in discussions, planning, and writing, with the lead of TA.
The authors declare that they have no conflict of interest.
This research was executed with the financial support of the Deutsche Forschungsgemeinschaft (DFG, German Research Foundation) priority program on “Climate Engineering–Risks, Challenges and Opportunities?” and specifically the CEMICS2 project. Further support came from the DFG under Germany's Excellence Strategy – EXC 2037 “Climate, Climatic Change, and Society” – project number 390683824, contribution to the Center for Earth System Research and Sustainability (CEN) of Universität Hamburg. We are grateful for the constructive comments and suggestions from the reviewers and editor.
This research has been supported by the Deutsche Forschungsgemeinschaft (DFG grant nos. SPP1689, EXC177, and EXC2037).
This paper was edited by Anja Rammig and reviewed by two anonymous referees.
Ahmad, M., Rajapaksha, A. U., Lim, J. E., Zhang, M., Bolan, N., Mohan, D., Vithanage, M., Lee, S. S., and Ok, Y. S.: Biochar as a sorbent for contaminant management in soil and water: a review, Chemosphere, 99, 19–33, https://doi.org/10.1016/j.chemosphere.2013.10.071, 2014.
Akhtar, S. S., Li, G., Andersen, M. N., and Liu, F.: Biochar enhances yield and quality of tomato under reduced irrigation, Agricultural Water Management, 138, 37–44, https://doi.org/10.1016/j.agwat.2014.02.016, 2014.
Alloway, B. J. (Ed.): Sources of Heavy Metals and Metalloids in Soils, in: Heavy Metals in Soils: Trace Metals and Metalloids in Soils and their Bioavailability, Springer Netherlands, Dordrecht, 11–50, 2013.
Al-Wabel, M. I., Hussain, Q., Usman, A. R. A., Ahmad, M., Abduljabbar, A., Sallam, A. S., and Ok, Y. S.: Impact of biochar properties on soil conditions and agricultural sustainability: A review, Land Degrad. Dev., 29, 2124–2161, https://doi.org/10.1002/ldr.2829, 2018.
Anda, M., Shamshuddin, J., and Fauziah, C. I.: Increasing negative charge and nutrient contents of a highly weathered soil using basalt and rice husk to promote cocoa growth under field conditions, Soil Till. Res., 132, 1–11, https://doi.org/10.1016/j.still.2013.04.005, 2013.
Anda, M., Shamshuddin, J., and Fauziah, C. I.: Improving chemical properties of a highly weathered soil using finely ground basalt rocks, Catena, 124, 147–161, https://doi.org/10.1016/j.catena.2014.09.012, 2015.
Atkinson, C., Fitzgerald, J., and Hipps, N.: Potential mechanisms for achieving agricultural benefits from biochar application to temperate soils: a review, Plant Soil, 337, 1–18, https://doi.org/10.1007/s11104-010-0464-5, 2010.
Bader, M. K. F., Leuzinger, S., Keel, S. G., Siegwolf, R. T. W., Hagedorn, F., Schleppi, P., Körner, C., and Lee, J.: Central European hardwood trees in a high-CO2 future: synthesis of an 8-year forest canopy CO2enrichment project, J. Ecol., 101, 1509–1519, https://doi.org/10.1111/1365-2745.12149, 2013.
Barnes, R. T., Gallagher, M. E., Masiello, C. A., Liu, Z., and Dugan, B.: Biochar-Induced Changes in Soil Hydraulic Conductivity and Dissolved Nutrient Fluxes Constrained by Laboratory Experiments, PLoS ONE, 9, e108340, https://doi.org/10.1371/journal.pone.0108340, 2014.
Basso, A. S., Miguez, F. E., Laird, D. A., Horton, R., and Westgate, M.: Assessing potential of biochar for increasing water-holding capacity of sandy soils, GCB Bioenergy, 5, 132–143, https://doi.org/10.1111/gcbb.12026, 2013.
Beerling, D. J., Leake, J. R., Long, S. P., Scholes, J. D., Ton, J., Nelson, P. N., Bird, M., Kantzas, E., Taylor, L. L., Sarkar, B., Kelland, M., DeLucia, E., Kantola, I., Muller, C., Rau, G., and Hansen, J.: Farming with crops and rocks to address global climate, food and soil security, Nat. Plants, 4, 138–147, https://doi.org/10.1038/s41477-018-0108-y, 2018.
Beesley, L., Moreno-Jiménez, E., Gomez-Eyles, J. L., Harris, E., Robinson, B., and Sizmur, T.: A review of biochars' potential role in the remediation, revegetation and restoration of contaminated soils, Environ. Poll., 159, 3269–3282, https://doi.org/10.1016/j.envpol.2011.07.023, 2011.
Benes, J., Kepka, P., and Konvicka, M.: Limestone quarries as refuges for European xerophilous butterflies, Conserv. Biol., 17, 1058–1069, https://doi.org/10.1046/j.1523-1739.2003.02092.x, 2003.
Benzerara, K., Yoon, T. H., Menguy, N., Tyliszczak, T., and Brown, G. E., Jr.: Nanoscale environments associated with bioweathering of a Mg-Fe-pyroxene, P. Natl. Acad. Sci. USA, 102, 979–982, https://doi.org/10.1073/pnas.0409029102, 2005.
Blouin, M., Hodson, M. E., Delgado, E. A., Baker, G., Brussaard, L., Butt, K. R., Dai, J., Dendooven, L., Peres, G., Tondoh, J. E., Cluzeau, D., and Brun, J. J.: A review of earthworm impact on soil function and ecosystem services, Eur. J. Soil Sci., 64, 161–182, https://doi.org/10.1111/ejss.12025, 2013.
Bodner, G., Nakhforoosh, A., and Kaul, H.-P.: Management of crop water under drought: a review, Agron. Sustain. Dev., 35, 401–442, https://doi.org/10.1007/s13593-015-0283-4, 2015.
Brantley, S. L., Kubicki, J. D., and White, A. F.: Kinetics of water-rock interaction, Springer, 2008.
Brosse, N., Dufour, A., Meng, X., Sun, Q., and Ragauskas, A.: Miscanthus: a fast-growing crop for biofuels and chemicals production, Biofuel Bioprod. Bior., 6, 580–598, https://doi.org/10.1002/bbb.1353, 2012.
Caldeira, K., Bala, G., and Cao, L.: The Science of Geoengineering, Annu. Rev. Earth Pl. Sc., 41, 231–256, https://doi.org/10.1146/annurev-earth-042711-105548, 2013.
Carcaillet, C.: Soil particles reworking evidences by AMS 14C dating of charcoal, Cr. Acad. Sci. II A, 332, 21–28, https://doi.org/10.1016/s1251-8050(00)01485-3, 2001.
Carpenter, D., Hodson, M. E., Eggleton, P., and Kirk, C.: Earthworm induced mineral weathering: Preliminary results, Eur. J. Soil Biol., 43, S176–S183, https://doi.org/10.1016/j.ejsobi.2007.08.053, 2007.
Carpenter, D., Hodson, M. E., Eggleton, P., and Kirk, C.: The role of earthworm communities in soil mineral weathering: a field experiment, Mineral. Mag., 72, 33–36, https://doi.org/10.1180/minmag.2008.072.1.33, 2008.
Ciais, P., Tans, P. P., Trolier, M., White, J. W. C., and Francey, R. J.: A Large Northern Hemisphere Terrestrial CO2 Sink Indicated by the 13C/12C Ratio of Atmospheric CO2, Science, 269, 1098–1102, https://doi.org/10.1126/science.269.5227.1098, 1995.
Ciceri, D., de Oliveira, M., Stokes, R. M., Skorina, T., and Allanore, A.: Characterization of potassium agrominerals: Correlations between petrographic features, comminution and leaching of ultrapotassic syenites, Miner. Eng., 102, 42–57, https://doi.org/10.1016/j.mineng.2016.11.016, 2017.
Ciceri, D. and Allanore, A.: Local fertilizers to achieve food self-sufficiency in Africa, Sci. Total Environ., 648, 669–680, https://doi.org/10.1016/j.scitotenv.2018.08.154, 2019.
Cuadros, J.: Clay minerals interaction with microorganisms: a review, Clay Miner., 52, 235–261, https://doi.org/10.1180/claymin.2017.052.2.05, 2018.
de Oliveira Garcia, W., Amann, T., and Hartmann, J.: Increasing biomass demand enlarges negative forest nutrient budget areas in wood export regions, Sci. Rep., 8, 5280, https://doi.org/10.1038/s41598-018-22728-5, 2018.
Dixit, S. and Hering, J. G.: Comparison of arsenic(V) and arsenic(III) sorption onto iron oxide minerals: implications for arsenic mobility, Environ. Sci. Technol., 37, 4182–4189, https://doi.org/10.1021/es030309t, 2003.
Drever, J. I.: The Effect of Land Plants on Weathering Rates of Silicate Minerals, Geochim. Cosmochim. Ac., 58, 2325–2332, https://doi.org/10.1016/0016-7037(94)90013-2, 1994.
Fuss, S., Canadell, J. G., Peters, G. P., Tavoni, M., Andrew, R. M., Ciais, P., Jackson, R. B., Jones, C. D., Kraxner, F., Nakicenovic, N., Le Quere, C., Raupach, M. R., Sharifi, A., Smith, P., and Yamagata, Y.: Betting on negative emissions, Nat. Clim. Change, 4, 850–853, https://doi.org/10.1038/nclimate2392, 2014.
Fuss, S., Jones, C. D., Kraxner, F., Peters, G. P., Smith, P., Tavoni, M., van Vuuren, D. P., Canadell, J. G., Jackson, R. B., Milne, J., Moreira, J. R., Nakicenovic, N., Sharifi, A., and Yamagata, Y.: Research priorities for negative emissions, Environ. Res. Lett., 11, 115007, https://doi.org/10.1088/1748-9326/11/11/115007, 2016.
Fuss, S., Lamb, W. F., Callaghan, M. W., Hilaire, J., Creutzig, F., Amann, T., Beringer, T., de Oliveira Garcia, W., Hartmann, J., Khanna, T., Luderer, G., Nemet, G. F., Rogelj, J., Smith, P., Vicente, J. L. V., Wilcox, J., del Mar Zamora Dominguez, M., and Minx, J. C.: Negative emissions – Part 2: Costs, potentials and side effects, Environ. Res. Lett., 13, 063002, https://doi.org/10.1088/1748-9326/aabf9f, 2018.
Gillman, G. P., Burkett, D. C., and Coventry, R. J.: A laboratory study of application of basalt dust to highly weathered soils: effect on soil cation chemistry, Soil Res., 39, 799-811, https://doi.org/10.1071/SR00073, 2001.
Goll, D. S., Brovkin, V., Parida, B. R., Reick, C. H., Kattge, J., Reich, P. B., van Bodegom, P. M., and Niinemets, Ü.: Nutrient limitation reduces land carbon uptake in simulations with a model of combined carbon, nitrogen and phosphorus cycling, Biogeosciences, 9, 3547–3569, https://doi.org/10.5194/bg-9-3547-2012, 2012.
Gonnelli, C. and Renella, G.: Chromium and Nickel, in: Heavy Metals in Soils: Trace Metals and Metalloids in Soils and their Bioavailability, edited by: Alloway, B. J., Springer Netherlands, Dordrecht, 313–333, 2013.
Graham, P. H. and Vance, C. P.: Nitrogen fixation in perspective: an overview of research and extension needs, Field Crop. Res., 65, 93–106, https://doi.org/10.1016/S0378-4290(99)00080-5, 2000.
Grainger, A.: Estimating Areas of Degraded Tropical Lands Requiring Replenishment of Forest Cover, International Tree Crops Journal, 5, 31–61, https://doi.org/10.1080/01435698.1988.9752837, 1988.
Gul, S., Whalen, J. K., Thomas, B. W., Sachdeva, V., and Deng, H.: Physico-chemical properties and microbial responses in biochar-amended soils: Mechanisms and future directions, Agr. Ecosyst. Environ., 206, 46–59, https://doi.org/10.1016/j.agee.2015.03.015, 2015.
Hamdan, J. and Bumham, C. P.: The contribution of nutrients from parent material in three deeply weathered soils of Peninsular Malaysia, Geoderma, 74, 219–233, https://doi.org/10.1016/s0016-7061(96)00062-6, 1996.
Hangx, S. J. T. and Spiers, C. J.: Coastal spreading of olivine to control atmospheric CO2 concentrations: A critical analysis of viability, Int. J. Greenh. Gas Con., 3, 757–767, https://doi.org/10.1016/j.ijggc.2009.07.001, 2009.
Harter, R. D.: Effect of Soil pH on Adsorption of Lead, Copper, Zinc, and Nickel1, Soil Sci. Soc. Am. J., 47, 47–51, https://doi.org/10.2136/sssaj1983.03615995004700010009x, 1983.
Hartmann, J., West, A. J., Renforth, P., Köhler, P., De La Rocha, C. L., Wolf-Gladrow, D. A., Dürr, H. H., and Scheffran, J.: Enhanced chemical weathering as a geoengineering strategy to reduce atmospheric carbon dioxide, supply nutrients, and mitigate ocean acidification, Rev. Geophys., 51, 113–149, https://doi.org/10.1002/rog.20004, 2013.
Helyar, K. R. and Porter, W. M.: 2 – Soil Acidification, its Measurement and the Processes Involved, in: Soil Acidity and Plant Growth, edited by: Robson, A., Academic Press, 61–101, 1989.
Hengl, T., Leenaars, J. G. B., Shepherd, K. D., Walsh, M. G., Heuvelink, G. B. M., Mamo, T., Tilahun, H., Berkhout, E., Cooper, M., Fegraus, E., Wheeler, I., and Kwabena, N. A.: Soil nutrient maps of Sub-Saharan Africa: assessment of soil nutrient content at 250 m spatial resolution using machine learning, Nutr. Cycl. Agroecosys., 109, 77–102, https://doi.org/10.1007/s10705-017-9870-x, 2017.
Hoffland, E., Kuyper, T. W., Wallander, H., Plassard, C., Gorbushina, A. A., Haselwandter, K., Holmstrom, S., Landeweert, R., Lundstrom, U. S., Rosling, A., Sen, R., Smits, M. M., van Hees, P. A., and van Breemen, N.: The role of fungi in weathering, Front. Ecol. Environ., 2, 258–264, https://doi.org/10.1890/1540-9295(2004)002[0258:TROFIW]2.0.CO;2, 2004.
Holloway, J. M. and Dahlgren, R. A.: Nitrogen in rock: Occurrences and biogeochemical implications, Glob. Biogeochem. Cy., 16, 1118, https://doi.org/10.1029/2002gb001862, 2002.
Houlton, B. Z., Morford, S. L., and Dahlgren, R. A.: Convergent evidence for widespread rock nitrogen sources in Earth's surface environment, Science, 360, 58–62, https://doi.org/10.1126/science.aan4399, 2018.
Jackson, R. B., Canadell, J. G., Le Quere, C., Andrew, R. M., Korsbakken, J. I., Peters, G. P., and Nakicenovic, N.: Reaching peak emissions, Nat. Clim. Change, 6, 7–10, https://doi.org/10.1038/nclimate2892, 2015.
Jeffery, S., Verheijen, F. G. A., van der Velde, M., and Bastos, A. C.: A quantitative review of the effects of biochar application to soils on crop productivity using meta-analysis, Agr. Ecosyst. Environ., 144, 175–187, https://doi.org/10.1016/j.agee.2011.08.015, 2011.
Jonard, M., Fürst, A., Verstraeten, A., Thimonier, A., Timmermann, V., Potočić, N., Waldner, P., Benham, S., Hansen, K., Merilä, P., Ponette, Q., de la Cruz, A. C., Roskams, P., Nicolas, M., Croisé, L., Ingerslev, M., Matteucci, G., Decinti, B., Bascietto, M., and Rautio, P.: Tree mineral nutrition is deteriorating in Europe, Glob. Change Biol., 21, 418–430, https://doi.org/10.1111/gcb.12657, 2015.
Kabata-Pendias, A.: Behavioral Properties of Trace-Metals in Soils, Appl. Geochem., 8, 3–9, https://doi.org/10.1016/S0883-2927(09)80002-4, 1993.
Kabata-Pendias, A.: Trace Elements in Soils and Plants, 4th edn., CRC Press, 548 pp., 2010.
Kang, Y., Khan, S., and Ma, X.: Climate change impacts on crop yield, crop water productivity and food security – A review, Prog. Nat. Sci., 19, 1665–1674, https://doi.org/10.1016/j.pnsc.2009.08.001, 2009.
Kantola, I. B., Masters, M. D., Beerling, D. J., Long, S. P., and DeLucia, E. H.: Potential of global croplands and bioenergy crops for climate change mitigation through deployment for enhanced weathering, Biol. Lett., 13, 20160714, https://doi.org/10.1098/rsbl.2016.0714, 2017.
Keller, D. P., Lenton, A., Littleton, E. W., Oschlies, A., Scott, V., and Vaughan, N. E.: The Effects of Carbon Dioxide Removal on the Carbon Cycle, Curr. Clim. Change Rep., 4, 250–265, https://doi.org/10.1007/s40641-018-0104-3, 2018.
Körner, C., Morgan, J., and Norby, R.: CO2 Fertilization: When, Where, How Much?, in: errestrial Ecosystems in a Changing World, Global Change – The IGBP Series, edited by: Canadell, J. G., Pataki, D. E., and Pitelka, L. F., Springer, Berlin, Heidelberg, 2007.
Kracher, D.: Nitrogen-Related Constraints of Carbon Uptake by Large-Scale Forest Expansion: Simulation Study for Climate Change and Management Scenarios, Earth's Future, 5, 1102–1118, https://doi.org/10.1002/2017ef000622, 2017.
Laird, D., Fleming, P., Wang, B., Horton, R., and Karlen, D.: Biochar impact on nutrient leaching from a Midwestern agricultural soil, Geoderma, 158, 436–442, https://doi.org/10.1016/j.geoderma.2010.05.012, 2010.
Lasaga, A. C.: Fundamental approaches in describing mineral dissolution and precipitation rates, Rev. Mineral Geochem., 31, 23–86, 1995.
Lehmann, J.: Bio-energy in the black, Front. Ecol. Environ., 5, 381–387, https://doi.org/10.1890/1540-9295(2007)5[381:Bitb]2.0.Co;2, 2007.
Lehmann, J., Rillig, M. C., Thies, J., Masiello, C. A., Hockaday, W. C., and Crowley, D.: Biochar effects on soil biota – A review, Soil Biol. Biochem., 43, 1812–1836, https://doi.org/10.1016/j.soilbio.2011.04.022, 2011.
Leonardos, O. H., Fyfe, W. S., and Kronberg, B. I.: The use of ground rocks in laterite systems: An improvement to the use of conventional soluble fertilizers?, Chem. Geol., 60, 361–370, https://doi.org/10.1016/0009-2541(87)90143-4, 1987.
Leuzinger, S., Luo, Y., Beier, C., Dieleman, W., Vicca, S., and Körner, C.: Do global change experiments overestimate impacts on terrestrial ecosystems?, Trends Ecol. Evol., 26, 236–241, https://doi.org/10.1016/j.tree.2011.02.011, 2011.
Li, D., Wen, L., Zhang, W., Yang, L., Xiao, K., Chen, H., and Wang, K.: Afforestation effects on soil organic carbon and nitrogen pools modulated by lithology, Forest Ecol. Manag., 400, 85–92, https://doi.org/10.1016/j.foreco.2017.05.050, 2017.
Liang, B., Lehmann, J., Solomon, D., Kinyangi, J., Grossman, J., O'Neill, B., Skjemstad, J. O., Thies, J., Luizão, F. J., Petersen, J., and Neves, E. G.: Black Carbon Increases Cation Exchange Capacity in Soils, Soil Sci. Soc. Am. J., 70, 1719, https://doi.org/10.2136/sssaj2005.0383, 2006.
Liu, Z., Dugan, B., Masiello, C. A., and Gonnermann, H. M.: Biochar particle size, shape, and porosity act together to influence soil water properties, PLoS One, 12, e0179079, https://doi.org/10.1371/journal.pone.0179079, 2017.
Loomis, R. S. and Morris, J. G.: Agricultural Productivity, BioScience, 33, 338–339, https://doi.org/10.2307/1309328, 1983.
Major, J., Lehmann, J., Rondon, M., and Goodale, C.: Fate of soil-applied black carbon: downward migration, leaching and soil respiration, Glob. Change Biol., 16, 1366–1379, https://doi.org/10.1111/j.1365-2486.2009.02044.x, 2010.
Manning, D. A. C.: Mineral sources of potassium for plant nutrition, A review, Agron. Sustain. Dev., Springer Netherlands, 2, 281–294, 2010.
Manning, D. A. C.: How will minerals feed the world in 2050?, P. Geologist Assoc., 126, 14–17, https://doi.org/10.1016/j.pgeola.2014.12.005, 2015.
Masiello, C., Dugan, B., Brewer, C., Spokas, K., Novak, J.-J., Liu, Z., and Sorrenti, G.: Biochar effects on soil hydrology, in: Biochar for Environmental Management Science, Technology and Implementation, edited by: Lehmann, J. and Joseph, S., Routledge, 2015.
McBride, M. B., Richards, B. K., and Steenhuis, T.: Bioavailability and crop uptake of trace elements in soil columns amended with sewage sludge products, Plant Soil, 262, 71–84, https://doi.org/10.1023/B:Plso.0000037031.21561.34, 2004.
Minx, J. C., Lamb, W. F., Callaghan, M. W., Fuss, S., Hilaire, J., Creutzig, F., Amann, T., Beringer, T., de Oliveira Garcia, W., Hartmann, J., Khanna, T., Lenzi, D., Luderer, G., Nemet, G. F., Rogelj, J., Smith, P., Vicente Vicente, J. L., Wilcox, J., and del Mar Zamora Dominguez, M.: Negative emissions – Part 1: Research landscape and synthesis, Environ. Res. Lett., 13, 063001, https://doi.org/10.1088/1748-9326/aabf9b, 2018.
Myers, S. S., Zanobetti, A., Kloog, I., Huybers, P., Leakey, A. D., Bloom, A. J., Carlisle, E., Dietterich, L. H., Fitzgerald, G., Hasegawa, T., Holbrook, N. M., Nelson, R. L., Ottman, M. J., Raboy, V., Sakai, H., Sartor, K. A., Schwartz, J., Seneweera, S., Tausz, M., and Usui, Y.: Increasing CO2 threatens human nutrition, Nature, 510, 139–142, https://doi.org/10.1038/nature13179, 2014.
Nagajyoti, P. C., Lee, K. D., and Sreekanth, T. V. M.: Heavy metals, occurrence and toxicity for plants: a review, Environ. Chem. Lett., 8, 199–216, https://doi.org/10.1007/s10311-010-0297-8, 2010.
National Research Council: Climate Intervention: Carbon Dioxide Removal and Reliable Sequestration, The National Academies Press, Washington, DC, 154 pp., 2015.
Nemet, G. F., Callaghan, M. W., Creutzig, F., Fuss, S., Hartmann, J., Hilaire, J., Lamb, W. F., Minx, J. C., Rogers, S., and Smith, P.: Negative emissions – Part 3: Innovation and upscaling, Environ. Res. Lett., 13, https://doi.org/10.1088/1748-9326/aabff4, 2018.
Nilsson, S. and Schopfhauser, W.: The carbon-sequestration potential of a global afforestation program, Clim. Change, 30, 267–293, https://doi.org/10.1007/BF01091928, 1995.
Nishanth, D. and Biswas, D. R.: Kinetics of phosphorus and potassium release from rock phosphate and waste mica enriched compost and their effect on yield and nutrient uptake by wheat (Triticum aestivum), Bioresource Technol., 99, 3342–3353, https://doi.org/10.1016/j.biortech.2007.08.025, 2008.
Norby, R. J. and Zak, D. R.: Ecological Lessons from Free-Air CO2 Enrichment (FACE) Experiments, Annu. Rev. Ecol. Evol. S., 42, 181–203, https://doi.org/10.1146/annurev-ecolsys-102209-144647, 2011.
Nunes, J. M. G., Kautzmann, R. M., and Oliveira, C.: Evaluation of the natural fertilizing potential of basalt dust wastes from the mining district of Nova Prata (Brazil), J. Clean Prod., 84, 649–656, https://doi.org/10.1016/j.jclepro.2014.04.032, 2014.
Omondi, M. O., Xia, X., Nahayo, A., Liu, X., Korai, P. K., and Pan, G.: Quantification of biochar effects on soil hydrological properties using meta-analysis of literature data, Geoderma, 274, 28–34, https://doi.org/10.1016/j.geoderma.2016.03.029, 2016.
Oren, R., Ellsworth, D. S., Johnsen, K. H., Phillips, N., Ewers, B. E., Maier, C., Schafer, K. V. R., McCarthy, H., Hendrey, G., McNulty, S. G., and Katul, G. G.: Soil fertility limits carbon sequestration by forest ecosystems in a CO2-enriched atmosphere, Nature, 411, 469–472, https://doi.org/10.1038/35078064, 2001.
Ornstein, L., Aleinov, I., and Rind, D.: Irrigated afforestation of the Sahara and Australian Outback to end global warming, Clim. Change, 97, 409–437, https://doi.org/10.1007/s10584-009-9626-y, 2009.
Perrin, A. S., Probst, A., and Probst, J. L.: Impact of nitrogenous fertilizers on carbonate dissolution in small agricultural catchments: Implications for weathering CO2 uptake at regional and global scales, Geochim. Cosmochim. Ac., 72, 3105–3123, 2008.
Pietikainen, J., Kiikkila, O., and Fritze, H.: Charcoal as a habitat for microbes and its effect on the microbial community of the underlying humus, Oikos, 89, 231–242, https://doi.org/10.1034/j.1600-0706.2000.890203.x, 2000.
Pinheiro, E. A. R., de Jong van Lier, Q., and Šimůnek, J.: The role of soil hydraulic properties in crop water use efficiency: A process-based analysis for some Brazilian scenarios, Agr. Syst., 173, 364–377, https://doi.org/10.1016/j.agsy.2019.03.019, 2019.
Qian, J., Shan, X.-Q., Wang, Z.-J., and Tu, Q.: Distribution and plant availability of heavy metals in different particle-size fractions of soil, Sci. Total Environ., 187, 131–141, https://doi.org/10.1016/0048-9697(96)05134-0, 1996.
Rajkumar, M., Sandhya, S., Prasad, M. N. V., and Freitas, H.: Perspectives of plant-associated microbes in heavy metal phytoremediation, Biotechnol. Adv., 30, 1562–1574, https://doi.org/10.1016/j.biotechadv.2012.04.011, 2012.
Rawls, W. J., Brakensiek, D. L., and Saxtonn, K. E.: Estimation of Soil Water Properties, T. ASAE, 25, 1316–1320, https://doi.org/10.13031/2013.33720, 1982.
Renforth, P., Pogge von Strandmann, P. A. E., and Henderson, G. M.: The dissolution of olivine added to soil: Implications for enhanced weathering, Appl. Geochem., 61, 109–118, https://doi.org/10.1016/j.apgeochem.2015.05.016, 2015.
Robinson, B. H., Brooks, R. R., Kirkman, J. H., Gregg, P. E. H., and Gremigni, P.: Plant-available elements in soils and their influence on the vegetation over ultramafic (“serpentine”) rocks in New Zealand, J. Roy. Soc. New Zeal., 26, 457–468, https://doi.org/10.1080/03014223.1996.9517520, 1996.
Rockstrom, J., Lannerstad, M., and Falkenmark, M.: Assessing the water challenge of a new green revolution in developing countries, P. Natl. Acad. Sci. USA, 104, 6253–6260, https://doi.org/10.1073/pnas.0605739104, 2007.
Rogelj, J., Popp, A., Calvin, K. V., Luderer, G., Emmerling, J., Gernaat, D., Fujimori, S., Strefler, J., Hasegawa, T., Marangoni, G., Krey, V., Kriegler, E., Riahi, K., van Vuuren, D. P., Doelman, J., Drouet, L., Edmonds, J., Fricko, O., Harmsen, M., Havlík, P., Humpenöder, F., Stehfest, E., and Tavoni, M.: Scenarios towards limiting global mean temperature increase below 1.5 ∘C, Nat. Clim. Change, 8, 325–332, https://doi.org/10.1038/s41558-018-0091-3, 2018.
Saito, M.: Charcoal as a micro-habitat for VA mycorrhizal fungi, and its practical implication, Agr. Ecosyst. Environ., 29, 341–344, https://doi.org/10.1016/0167-8809(90)90298-r, 1990.
Sarbas, B.: The GEOROC Database as Part of a Growing Geoinformatics Network, Geoinformatics 2008 – Data to Knowledge, Potsdam, 2008.
Schuiling, R. D. and Krijgsman, P.: Enhanced weathering: An effective and cheap tool to sequester CO2, Clim. Change, 74, 349–354, https://doi.org/10.1007/s10584-005-3485-y, 2006.
Schuiling, R. D.: Farming nickel from non-ore deposits, combined with CO2 sequestration, Nat. Sci., 5, 445–448, https://doi.org/10.4236/ns.2013.54057, 2013.
Schwartzman, D. W. and Volk, T.: Biotic Enhancement of Weathering and the Habitability of Earth, Nature, 340, 457–460, https://doi.org/10.1038/340457a0, 1989.
Semhi, K., Suchet, P. A., Clauer, N., and Probst, J. L.: Impact of nitrogen fertilizers on the natural weathering-erosion processes and fluvial transport in the Garonne basin, Appl. Geochem., 15, 865–878, https://doi.org/10.1016/S0883-2927(99)00076-1, 2000.
Smith, M. R. and Myers, S. S.: Impact of anthropogenic CO2 emissions on global human nutrition, Nat. Clim. Change, 8, 834–839, https://doi.org/10.1038/s41558-018-0253-3, 2018.
Strefler, J., Amann, T., Bauer, N., Kriegler, E., and Hartmann, J.: Potential and costs of carbon dioxide removal by enhanced weathering of rocks, Environ. Res. Lett., 13, 034010, https://doi.org/10.1088/1748-9326/aaa9c4, 2018.
Tack, F. M., Callewaert, O. W. J. J., and Verloo, M. G.: Metal solubility as a function of pH in a contaminated, dredged sediment affected by oxidation, Environ. Poll., 91, 199–208, https://doi.org/10.1016/0269-7491(95)00049-6, 1996.
Taylor, L. L., Quirk, J., Thorley, R. M. S., Kharecha, P. A., Hansen, J., Ridgwell, A., Lomas, M. R., Banwart, S. A., and Beerling, D. J.: Enhanced weathering strategies for stabilizing climate and averting ocean acidification, Nat. Clim. Change, 6, 402–406, https://doi.org/10.1038/nclimate2882, 2015.
Tilman, D., Socolow, R., Foley, J. A., Hill, J., Larson, E., Lynd, L., Pacala, S., Reilly, J., Searchinger, T., Somerville, C., and Williams, R.: Beneficial Biofuels – The Food, Energy, and Environment Trilemma, Science, 325, 270–271, https://doi.org/10.1126/science.1177970, 2009.
Topoliantz, S. and Ponge, J.-F.: Charcoal consumption and casting activity by Pontoscolex corethrurus (Glossoscolecidae), Appl. Soil Ecol., 28, 217–224, https://doi.org/10.1016/j.apsoil.2004.08.003, 2005.
Tropek, R., Kadlec, T., Karesova, P., Spitzer, L., Kocarek, P., Malenovsky, I., Banar, P., Tuf, I. H., Hejda, M., and Konvicka, M.: Spontaneous succession in limestone quarries as an effective restoration tool for endangered arthropods and plants, J. Appl. Ecol., 47, 139–147, https://doi.org/10.1111/j.1365-2664.2009.01746.x, 2010.
Uroz, S., Calvaruso, C., Turpault, M.-P., and Frey-Klett, P.: Mineral weathering by bacteria: ecology, actors and mechanisms, T. Microbiol., 17, 378–387, https://doi.org/10.1016/j.tim.2009.05.004, 2009.
van Straaten, P.: Rocks for Crops: Agrominerals of sub-Saharan Africa, ICRAF, Nairobi, Kenya, 338 pp., 2002.
van Straaten, P.: Farming with rocks and minerals: challenges and opportunities, An. Acad. Bras. Cienc., 78, 731–747, 2006.
Warnock, D. D., Lehmann, J., Kuyper, T. W., and Rillig, M. C.: Mycorrhizal responses to biochar in soil – concepts and mechanisms, Plant Soil, 300, 9–20, https://doi.org/10.1007/s11104-007-9391-5, 2007.
West, T. O. and McBride, A. C.: The contribution of agricultural lime to carbon dioxide emissions in the United States: dissolution, transport, and net emissions, Agr. Ecosyst. Environ., 108, 145–154, https://doi.org/10.1016/j.agee.2005.01.002, 2005.
White, J. G. and Zasoski, R. J.: Mapping soil micronutrients, Field Crop. Res., 60, 11–26, https://doi.org/10.1016/s0378-4290(98)00130-0, 1999.
Xu, C. Y., Hosseini-Bai, S., Hao, Y., Rachaputi, R. C., Wang, H., Xu, Z., and Wallace, H.: Effect of biochar amendment on yield and photosynthesis of peanut on two types of soils, Environ. Sci. Pollut. Res. Int., 22, 6112–6125, https://doi.org/10.1007/s11356-014-3820-9, 2015.
Zhang, G.-l., Liu, F., and Song, X.-D.: Recent progress and future prospect of digital soil mapping: A review, J. Integr. Agr., 16, 2871–2885, https://doi.org/10.1016/s2095-3119(17)61762-3, 2017.
Zomer, R. J., Trabucco, A., Bossio, D. A., and Verchot, L. V.: Climate change mitigation: A spatial analysis of global land suitability for clean development mechanism afforestation and reforestation, Agr. Ecosyst. Environ., 126, 67–80, https://doi.org/10.1016/j.agee.2008.01.014, 2008.