the Creative Commons Attribution 4.0 License.
the Creative Commons Attribution 4.0 License.
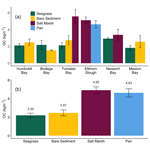
Blue carbon stocks and exchanges along the California coast
Tessa M. Hill
Chelsey Souza
Tessa Filipczyk
Aurora M. Ricart
Sarah Merolla
Lena R. Capece
Brady C O'Donnell
Kristen Elsmore
Walter C. Oechel
Kathryn M. Beheshti
Salt marshes and seagrass meadows can sequester and store high quantities of organic carbon (OC) in their sediments relative to other marine and terrestrial habitats. Assessing carbon stocks, carbon sources, and the transfer of carbon between habitats within coastal seascapes are each integral in identifying the role of blue carbon habitats in coastal carbon cycling. Here, we quantified carbon stocks, sources, and exchanges in seagrass meadows, salt marshes, and unvegetated sediments in six bays along the California coast. In the top 20 cm of sediment, the salt marshes contained approximately twice as much OC as seagrass meadows did, 4.92 ± 0.36 kg OC m−2 compared to 2.20 ± 0.24 kg OC m−2, respectively. Both salt marsh and seagrass sediment carbon stocks were higher than previous estimates from this region but lower than global and US-wide averages, respectively. Seagrass-derived carbon was deposited annually into adjacent marshes during fall seagrass senescence. However, isotope mixing models estimate that negligible amounts of this seagrass material were ultimately buried in underlying sediment. Rather, the vast majority of OC in sediment across sites was likely derived from planktonic/benthic diatoms and/or C3 salt marsh plants.
- Article
(3081 KB) -
Supplement
(25992 KB) - BibTeX
- EndNote
As carbon dioxide (CO2) concentrations in the oceans and atmosphere continue to rise, interest in measuring the relative quantities of carbon stored within natural ecosystems has increased. These assessments can help improve global and regional climate models, the prediction of future CO2 concentrations related to sources and sinks, and our broader understanding of nature-based climate change solutions (Serrano et al., 2019). Coastal habitats including seagrasses, salt marshes, and mangroves have earned the moniker “blue carbon” habitats for their ability to store and sequester disproportionally high levels of organic carbon (OC) in their sediments relative to other habitat types, thereby potentially serving in a management context to provide carbon mitigation (Lovelock and Duarte, 2019; McLeod et al., 2011). This can be largely attributed to the tendency for these habitats to exhibit high sediment accretion rates and low decomposition rates (Peck et al., 2020; Serrano et al., 2019). This ability has led to increasing interest in blue carbon habitats given that their conservation can prevent significant emission of stored carbon (Lovelock et al., 2017; Pendleton et al., 2012) and their restoration can lead to increased drawdown of atmospheric CO2 (Freedman et al., 2009; Greiner et al., 2013).
1.1 Carbon stock assessments and their significance
Despite global interest, many regions are still lacking basic information on carbon stocks and burial rates in blue carbon habitats. This information forms the foundation for more advanced scientific research and can be extremely valuable within management contexts to develop informed local and regional climate assessments. Salt marshes and seagrass meadows cover extensive portions of North America's west coast, yet their carbon stocks have been relatively understudied compared to other habitats in North America and other blue carbon habitats in different regions of the world (Ouyang and Lee, 2014; Postlethwaite et al., 2018).
Eelgrass (Zostera marina) is the dominant seagrass species in North America and occupies coastal waterways from Alaska to Mexico (Green and Short, 2003). Until recently, seagrass carbon stock data were almost entirely absent along the west coast of North America (see Capece, 2019; Kauffman et al., 2020; O'Donnell, 2017; Poppe and Rybczyk, 2018; Postlethwaite et al., 2018; Röhr et al., 2018). Of these studies, very little data came from central or southern California seagrass meadows despite the fact that this region represents a key temperate to subtropical transition in Z. marina's range (Cabello-Pasini et al., 2003). As a result of the paucity of data from the west coast of North America, this region was not represented in previous global syntheses of seagrass carbon stocks (Duarte et al., 2010; Fourqurean et al., 2012; Mazarrasa et al., 2015). Existing work on global seagrass carbon storage identifies that two species of seagrasses in the Mediterranean (Posidonia oceanica) and Australia (Posidonia australis) store significantly more carbon than other seagrass species, including Z. marina (Fourqurean et al., 2012; Lavery et al., 2013; Prentice et al., 2020). These Posidonia species were overrepresented in some early assessments of total global seagrass carbon storage – making these global estimates unreliable when applied to management decisions or climate models (Johannessen and Macdonald, 2016; Kennedy et al., 2010). In fact, the geographic and interspecies variability in carbon stocks is likely greater than was initially anticipated (Macreadie et al., 2018; Postlethwaite et al., 2018).
Salt marsh carbon stocks are similarly understudied in western North America, with published carbon stock data from only four estuaries in the region and very minimal spatial coverage and analyses performed in three of these locations (Brevik and Homburg, 2004; Callaway et al., 2012; Kauffman et al., 2020; Patrick and DeLaune, 1990). Existing analyses of North American freshwater wetlands and salt marshes are typically dominated by studies along the east coast (Nahlik and Fennessy, 2016; Wilkinson et al., 2018; with the exception of Holmquist et al., 2018). Within west coast studies, considerable variation in carbon stocks is observed (Callaway et al., 2012; Chmura et al., 2003). Similar to seagrass meadows, these regions have distinctly different oceanographic and geomorphological regimes, which can drive differences in sediment carbon storage. Thus, seagrass and salt marsh carbon storage data collected from understudied regions and across varying environmental gradients are necessary for understanding carbon stock variability and its drivers.
1.2 Blue carbon sources and drivers
Despite increasing information on blue carbon habitats in the last decade, a number of questions remain before we can fully understand their role in regional carbon cycling and climate adaptation (Macreadie et al., 2019). We do not yet fully understand what drives variation in carbon stocks – a key aspect of ensuring the protection and enhancement of these carbon services in the future. Previous work demonstrates that numerous factors can control carbon accumulation in coastal sediments including overlying biomass, topography, hydrology, mineralogy, and remineralization rates (Kelleway et al., 2016; Lima et al., 2020; Mazarrasa et al., 2018; Prentice et al., 2019). In particular, sediment grain size has been demonstrated to be a significant predictor of carbon stocks (Dahl et al., 2016; O'Donnell, 2017; Serrano et al., 2016) as it affects decomposition rates, likely related to the deposition of small particles and low resuspension from the attenuation of water flow by seagrasses (Bos et al., 2007; Conley et al., 2017; Gambi et al., 1990; Hendriks et al., 2008). Through similar pathways, fine, silty sediments trapped in tidal salt marshes can also increase carbon storage (e.g., Zhou et al., 2007).
The relative importance of each potential driver of carbon stock variability may be highly dependent on the environmental setting, species composition, and the interactive effects of these drivers. For example, in Australian salt marshes, overlying vegetation was shown to only significantly affect carbon stocks in sandy and mixed grain size sediments, having no effect on stocks in fine sediments (Kelleway et al., 2016). However, in other cases vegetation has been the primary predictor of carbon stocks in salt marshes, irrespective of grain size (Lovelock et al., 2014; Saintilan et al., 2013). Similar region- and species-specific complexities between carbon stocks, overlying vegetation, and mineralogy have been described in seagrass meadows (e.g., Lima et al., 2020; Serrano et al., 2016), meriting further investigation to understand these complex interactions.
Knowing the relative contributions of locally produced (autochthonous) and imported (allochthonous) carbon also elucidates the underlying mechanisms by which blue carbon habitats store and accumulate carbon. Overlying vegetation can significantly impact sediment carbon stocks; however, it is rarely the dominant source of carbon buried within blue carbon habitats (Kennedy et al., 2010; Ewers Lewis et al., 2020; Mazarrasa et al., 2015; Prentice et al., 2019). In many cases, contributions from terrestrial habitats, macroalgae, and suspended particulate organic material contribute as much or more to carbon buried in blue carbon sediments than autochthonous sources do (Drexler et al., 2020; Kennedy et al., 2010; Leorri et al., 2018; Ricart et al., 2020). Thus, local primary production could significantly contribute to net annual carbon drawdown within a given habitat yet play a minor role in carbon burial due to lateral export or remineralization of particulate organic carbon. Understanding lateral carbon transport elucidates the role of blue carbon habitats in broader, system-wide energy flows and carbon cycling (Hyndes et al., 2014; Ricart et al., 2015). For instance, much of the carbon from laterally exported biomass may be remineralized in the water column or as wrack in nearby habitats (Attard et al., 2019; Liu et al., 2019), serving an important ecological role and altering the production and biogeochemical cycles of recipient systems (Hyndes et al., 2014; Ince et al., 2007; Valiela and Cole, 2002). Given that blue carbon habitats are highly productive (Duarte and Cebrián, 1996), support high carbon burial (e.g., McLeod et al., 2011), and can co-occur within small geographic ranges (Alongi, 2018; Bouillon and Connolly, 2009), laterally exported carbon from one blue carbon habitat may be entrapped and buried in a neighboring habitat. From this landscape-scale perspective, exchanges between blue carbon habitats could increase their capacity for carbon burial or alter the sources of buried carbon. While some coastal studies have estimated lateral carbon fluxes (Jiménez et al., 2017; Liu et al., 2019), very few studies place these lateral fluxes into landscape-scale contexts that also address carbon burial in recipient habitats (Bouillon and Connolly, 2009; Duarte and Krause-Jensen, 2017; Ricart et al., 2017).
This study addresses these globally relevant topics of research in a relatively understudied region by answering the following questions:
-
How much organic carbon is stored in seagrass and salt marsh sediments across a latitudinal gradient?
-
What are the sources of carbon buried within sediments?
-
Is carbon exchanged between blue carbon habitats within the coastal landscape?
2.1 Study sites
Sediment cores from salt marshes and seagrass meadows, along with neighboring unvegetated sediments (hereafter “bare sediment” near seagrass meadows and “pan” near salt marshes), were collected from six bays across a latitudinal gradient in California (Fig. 1a). A total of 82 sediment cores were collected, 30 of which have been discussed previously (O'Donnell, 2017) and are included here for comparison. The number of cores collected in each site and general site characteristics are described in Table 1. While all sampled seagrass meadows were dominated by a single seagrass species (Zostera marina), salt marshes contained a mixed community of halophytes, predominantly composed of pickleweed (Sarcocornia pacifica), and to a lesser extent salt grass (Distichlis spicata) and marsh jaumea (Jaumea carnosa). Bare sediment cores were collected in unvegetated areas near each seagrass meadow at a minimum of 20 m away from the meadows and at similar depths. Pan cores were collected from patches of unvegetated sediment (2–4 m diameter) found within the salt marsh interior, a natural and semipermanent feature of salt marsh habitats formed by elevational depressions (Escapa et al., 2015). None of our sampling sites were actively restored, and, to our knowledge, respective vegetation has persisted through time.
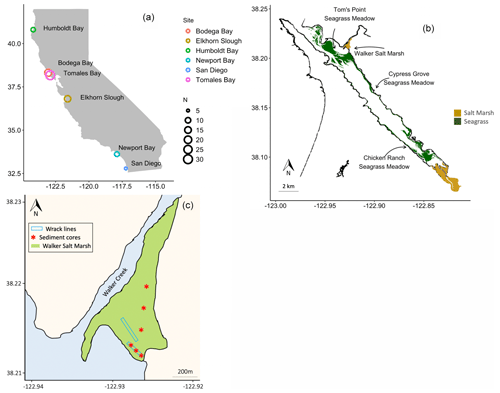
Figure 1Sediment cores were collected from six estuaries across a latitudinal gradient in California, where N = number of cores (a). Seagrass wrack deposition into Walker Salt Marsh from surrounding seagrass meadows (Merkel and Associates, 2017) was estimated, while sediment cores were taken from all four labeled habitats (b). Three sediment cores were collected beneath persistent wrack lines in Walker Salt Marsh, while three were collected from areas that do not receive regular, annual tidal deposition of wrack (c).
We estimated OC stocks in seagrass, salt marsh, pan, and bare sediments (see details below). In addition, we analyzed sediment OC sources for two individual salt marshes in Elkhorn Slough (“Elkhorn salt marsh”) and Tomales Bay (“Walker Salt Marsh”), and we examined carbon exchange between a single seagrass meadow and the neighboring Walker Salt Marsh. Walker Salt Marsh is located approximately 5.5 km from the mouth of Tomales Bay and is in close proximity to extensive meadows of Z. marina – the dominant seagrass in each of the meadows sampled (Fig. 1b). This marsh lies where the mouth of Walker Creek meets bay waters and thus can receive terrestrial and riverine inputs while simultaneously receiving marine inputs from tidal exchange.
2.2 Carbon stocks
All sediment cores were sampled by manually inserting transparent, open-barrel PVC pipes (20 cm length, 5.08 cm diameter). Compaction occurred in 19 % of cores, and a compaction factor was applied when calculating carbon stocks according to Howard et al. (2014). Once extracted, cores were capped and transported to the laboratory upright to prevent mixing of sediment layers. Cores were then immediately extruded into sections at 2 cm intervals. Coarse living plant material (> 1 cm) was manually removed. Each section was dried at 60 ∘C and weighed, and dry bulk density (DBD) was determined by dividing dry bulk mass by the volume of sampling interval.
Each section was then homogenized and divided into three subsamples of 10 g each, and the remaining sample was archived. One of the three subsamples was acidified using 1.12 M HCl to remove and measure total inorganic carbon (Milliman, 1974). The second subsample was analyzed for total organic material (TOM) by the loss-on-ignition method for 4 h at 550 ∘C (Dean, 1974). Total C content (%) and δ13C were additionally determined on a set of 44 of the acidified subsamples randomly selected across each habitat type using an elemental analyzer (PDZ Europa ANCA-GSL, SD ± 0.25 %) integrated with a continuous flow isotope ratio mass spectrometer (PDZ Europa 20-20, SD ± 0.2 ‰) at the UC Davis Stable Isotope Facility. The δ13C ratios are expressed in parts per thousand (‰) relative to VPDB (Vienna Pee Dee Belemnite) according to standard notation (δ13C = × 1000, where R is the ratio 13C 12C). Organic carbon in each core section was then estimated using a power model developed between measured TOM and measured OC in this set of subsamples (as in Craft et al., 1991). Specifically, the equation was applied (r2=0.84; Fig. S1). A power model was selected over a linear model for these data to avoid negative estimates of carbon stocks at low levels of TOM (Fig. S1). Carbon stocks were determined by multiplying DBD (g cm−3) by percent OC.
In each core section, the proportion of fine sediments – the silt and clay fraction (< 63 µm), hereafter “mud” – was quantified. The grain size analysis was conducted by mass loss in the third subsample from each section in 70 of the 82 total cores. The subsample was rinsed through a 63 µm sieve with deionized water, and the remaining sediments were dried at 60 ∘C and re-weighed.
Comparable to previous studies, a core depth of 20 cm was selected (Fourqurean et al., 2012; Prentice et al., 2020; Röhr et al., 2018). As such, stock estimates are presented in kilograms OC per square meter (kg OC m−2) in the top 20 cm of sediment. However, given that the selected depths for stock estimates vary throughout the literature, we extrapolate all data to 1 m when comparing across studies. Some research suggests that OC content remains relatively constant below 10 cm to depths up to 1 m, making this extrapolation appropriate (Callaway et al., 2012; Prentice et al., 2020; Fig. S3), while evidence of downcore variability in other studies makes extrapolation less appropriate (e.g., St. Laurent et al., 2020; Serrano et al., 2012). When comparing the carbon stocks estimated here to those in previous studies, we include each studies' sampled core depths for clarity.
2.3 Carbon sources and exchange
We applied mixing models to assess salt marsh sediment carbon sources to understand within-estuary exchange of carbon among the sampled habitats. Two separate mixing models were produced for (1) Elkhorn salt marsh in Elkhorn Slough and Walker Salt Marsh in Tomales Bay and (2) salt marsh sediments under seagrass wrack versus not under wrack in Walker Salt Marsh. At Walker Salt Marsh, a total of six sediment cores were collected. Three of these sediment cores were collected from the interior marsh, while three were collected from underneath a seagrass wrack line along the tidal edge of the marsh (Fig. 1c). Z. marina in this region is known to undergo a period of senescence as photoperiod shortens in the fall, as is common in all temperate seagrasses (Fourqurean et al., 1997). Historical imagery of the site shows persistent seagrass wrack concentrated along these tide lines that consistently appears in early fall as seagrass senesces (Fig. S2; Google Earth, 2020). The biomass of seagrass wrack along this tide line at the time of core collection was quantified along an 80 m transect within the marsh by collecting all seagrass present in a 1 m2 quadrat every 10 m. This material was taken back to the lab, sorted into aboveground biomass (AGB) and belowground biomass (BGB), rinsed, dried (60 ∘C), and weighed. Biomass data from seagrass in nearby meadows were previously published (see O'Donnell, 2017) and are used here for reference. Sediment cores collected from beneath wrack lines were sectioned at 2 cm intervals and analyzed for total OC and δ13C content according to the methods described above. Total nitrogen content was also determined on an unacidified portion of the same 44 sediment subsamples used in carbon analyses (Thermo Finnigan Flash 1112 Series elemental analyzer, SD ± 0.02 %). Data from Walker Salt Marsh are also displayed as “shallow” (surface sediment to 10 cm deep) and “deep” (10 cm and deeper) to facilitate the interpretation of changes with depth.
The contributions of carbon sources to each core section were then estimated with a mixing model using δ13C and N:C ratios as tracers. Given δ15N can be altered during early diagenesis (e.g., Benner et al., 1991), we selected δ13C and C:N ratios as the primary geochemical tracers and therefore did not include fractionation factors in the model (Craven et al., 2017). N:C ratios are utilized rather than C:N ratios because mixed fractions returned by the model are based on the denominator, and thus 13C : 12C and N:C both estimate the fractional contribution of 12C (Craven et al., 2017; Perdue and Koprivnjak, 2007). However, C:N ratios are used in text and figures given this format is more typically presented in the literature. We used the Bayesian mixing model SIAR 4.2 (Parnell and Jackson, 2013) to estimate the contributions of several source groups selected according to the dominant plant and algal species observed within the selected salt marshes. The sources included C3 salt marsh plants, C4 salt marsh plants (Distichlis spicata), seagrass (Z. marina), and plankton/benthic diatoms. Seagrass geochemistry was estimated from seagrass leaves collected from meadows near the selected salt marshes in Newport Bay, Tomales Bay, and Bodega Bay (Fig. 1a; Capece, 2019). Representative geochemistry for all other sources has been published previously and was used here from samples collected in San Francisco Bay, approximately 60 km south of Walker Salt Marsh (see Cloern et al., 2002, for full methods). All salt marsh sediment cores were collected from areas of the marsh that were dominated by the aforementioned species, and as such, other marsh species were not considered (e.g., Spartina sp.). While lower densities of other C3 marsh plants may have been present in some cored sites, the variability demonstrated by the selected source samples likely encompasses much of this variability given the utilization of the same photosynthetic pathway (Cloern et al., 2002). Plankton samples were collected from estuarine water samples, while benthic diatom samples were collected from both salt marsh surface sediments and neighboring mudflats (Cloern et al., 2002). These sources have similar isotopic values and are pooled here and referred to as “diatoms” for simplicity, acknowledging that this encompasses contributions from multiple planktonic and benthic sources. Given the overlapping isotopic values of C3 plants and diatoms, these two sources were pooled in mixing models, allowing an estimate of diatom and/or C3 plant contributions to marsh sediments, in addition to contributions from seagrass and C4 plants.
2.4 Statistical analyses
To evaluate decay of carbon through time, we tested for significant changes in OC in each habitat type with core section depth (a proxy for time) by fitting data to a generalized linear mixed model (GLMM) using maximum likelihood with “depth” and “site” as fixed effects and “core” as a random effect and using a gamma distribution and log link function to account for non-normality. In sites and habitats that demonstrated significant OC changes with depth, the rate of decay was estimated from the slope of its associated model. In all analyses to follow, OC across all sections in each core were averaged, and statistics were performed on these core averages. After inspecting data for normality and homogeneity of variance, differences in OC and grain size between habitat types and between sites were analyzed using simple linear models (SLMs; significance defined by α=0.05). Specifically, these data were fit to a linear model using ordinary least squares with “site” and “habitat (i.e., seagrass, salt marsh, bare sediment, pan) as fixed effects, including their interaction. The relationships between TOM (%) and grain size were analyzed using simple linear regressions, whereby a grain size filter was selectively applied to determine the point at which the relationship between the two was no longer significant (p<0.05). Differences in δ13C (‰) or C:N ratios between sediment depth (“surface” versus “deep”), and between sediments collected under wrack versus not under wrack, were also tested with SLMs using ordinary least squares with “depth” or “under wrack” as fixed effects. When necessary, data were log transformed. Tukey's post hoc analyses were conducted for multiple comparisons. All statistical analyses were performed in R software (R Core Team, 2018).
3.1 Carbon stock assessments
Down core OC demonstrates high variability, resulting in few significant differences in OC with depth (Fig. 2). Specifically, only Newport Bay salt marsh sediments exhibited significant loss of OC down core, which declined at a rate of 0.001 g C cm−3 per centimeter depth (Fig. 2e; GLMM, T-value = −4.7, SE = 0.01, p<0.05). Significant differences between OC stocks emerged when cores were compared between habitats, with salt marshes containing significantly more carbon than both bare sediment and seagrass meadows (Fig. 3b; SLM, p<0.01, F statistic = 13.3, DF = 3). Specifically, within the top 20 cm of sediment, salt marsh sediments contained 4.92 ± 0.36 kg OC m−2, while seagrass meadows contained 2.20 ± 0.24 kg OC m−2 (Fig. 3b; mean ± SE). Seagrass meadow carbon stocks were not significantly different than those of nearby bare sediments (Tukey's post hoc analysis, p>0.05), which contained an average of 2.47 ± 0.32 kg OC m−2. Pan sediments also contained higher carbon than seagrass meadows did (Tukey's post hoc analysis, p>0.05). A full list of carbon stocks is displayed in Table S1. Tukey's post hoc analysis indicates that in Tomales Bay, salt marshes contained significantly more carbon than seagrass meadows (Fig. 3a; p<0.05), while bare sediment and seagrass carbon stocks did not significantly differ from one another (p>0.05). We did not detect any other significant differences when comparing carbon stocks across habitat types within each individual site (Tukey's post hoc analysis, p>0.05).
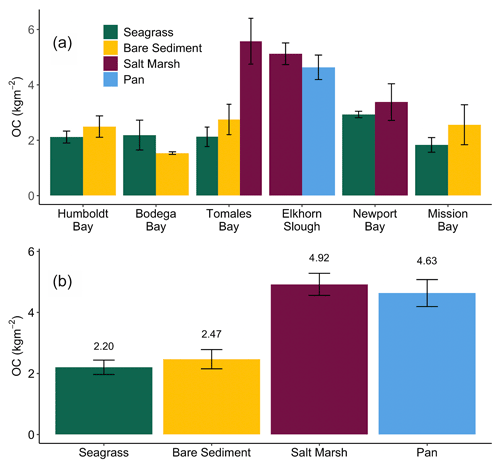
Figure 3Average (± SE) total organic carbon (OC) stocks (kg m−2) across all cores collected from each habitat type within each site in this study (a). Average (± SE) total organic carbon stocks (kg m−2) across all cores collected within each habitat type in this study (b). Stocks are representative of the top 20 cm of sediment.
We observed a very strong relationship between grain size and storage of organic material, especially at lower TOM (%) values (Fig. 4). Specifically, the observed linear relationship between grain size and TOM is strongest when sediment is 8.5 % TOM or less (linear model, r2=0.95, p<0.05, F statistic = 4957, DF = 269). However, as the proportion of fine sediments in each sample increases, the relationship between grain size and TOM weakens rapidly (from r2=0.73 to 0.53) after sediments consisting of more than 82 % mud are included (linear model, p<0.05, r2=0.53, F statistic = 233.1, DF = 201). Our results indicate that grain size was similar between sites (SLM, p>0.05, F statistic = 2.7, DF = 3) but differed between habitat types (Fig. 5a; SLM, p<0.05, F statistic = 5.7, DF = 3), with salt marsh sediments demonstrating significantly greater percent mud than both seagrass and bare sediment (Tukey's post hoc analysis, p<0.05). Recognizing that the sample size of pan cores was low, pan sediments displayed comparable percent mud to salt marsh sediments, but there were no significant differences between pan grain size and the other habitat types' grain sizes (Tukey's post hoc analysis, p>0.05). Tukey's post hoc analyses did not indicate any significant differences in grain size between habitat types within each site (Fig. 5a; p>0.05).
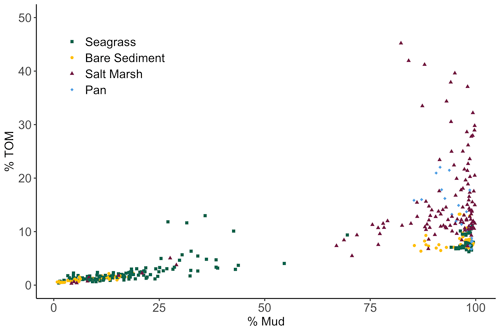
Figure 4Biplot showing the relationship among total organic material (TOM) (%) of each 2 cm core section from each habitat type plotted against sediment grain size (% Mud).
3.2 Carbon sources and exchange
3.2.1 Wrack deposition
While we conducted only one ground survey of seagrass wrack lines within Walker Salt Marsh in October 2019, historical aerial imagery was utilized to verify that these wrack lines appear reliably in this season in similar locations each year (Fig. S2). This phenomenon can be viewed as far back as 2002, before which images are of low quality or unavailable (Google Earth, 2020). These wrack lines typically persist throughout the winter, becoming indiscernible via aerial imagery by spring. Previous data collected by the authors (O'Donnell, 2017) quantified seagrass senescence within the nearby Tom's Point seagrass meadow (Fig. 1b), where average summer seagrass biomass was 440 ± 59.4 g m−2 and winter seagrass biomass was 115 ± 16.5 g m−2 (AGB and BGB, mean ± SE; Table 2). Within the neighboring Walker Salt Marsh, fall estimates of wrack demonstrated that 106 ± 24.6 g m−2 of seagrass (dry weight) was deposited along tide lines (Table 2). While both seagrass AGB and BGB are included in this value, seagrass BGB only accounted for 3.5 % of total seagrass biomass measured (Table 2). Analysis of seagrass leaves collected from Tomales Bay demonstrated that seagrass material was composed of 31.6 % of OC. Thus, we estimate Walker Salt Marsh receives 33.4 ± 7.6 g OC m−2 in the form of seagrass along wrack lines each year.
Table 2Living seagrass aboveground biomass (AGB) and belowground biomass (BGB) were collected in Tom's Point seagrass meadow (Fig. 1b) in both summer and winter (g m−2). Total seagrass wrack delivered to Walker Salt Marsh was quantified as AGB and BGB (g m−2) and converted to carbon (g C m−2) using a 31.6 % carbon conversion rate.
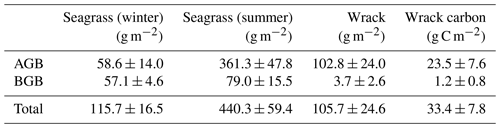
3.2.2 Sediment carbon sources
In Walker Salt Marsh, surface (< 10 cm) wrack sediments had higher δ13C values than sediments collected from the interior of the marsh (non-wrack sediments) and thus were more similar to the δ13C values of seagrass (Fig. 6; SLM, p<0.05; F statistic = 27.3; DF = 18). Shallow wrack sediments had an average δ13C of −22.5 ± 0.38 ‰, while non-wrack sediments had an average δ13C of −24.9 ± 0.26 ‰ (mean ± SE). However, when both shallow and deep (> 10 cm) sediments were included, wrack sediment δ13C did not significantly differ from non-wrack sediments (SLM, p>0.05, F statistic = 0.34, DF = 31). C:N sediment ratios did not significantly differ from one another regardless of collection depth or location (SLMs, p>0.05). When data were applied to a mixing model, apparent discrepancies in seagrass-derived carbon contributions between shallow and deep, as well as wrack and non-wrack sediments, were insignificant (SIAR mixing model, Table S2). Similarly, no significant quantity of seagrass-derived carbon was detected in Elkhorn salt marsh sediments (SIAR mixing model, Table S2). Rather, models estimate that sediments were derived almost entirely from either diatoms or C3 plants – 83 % in Elkhorn Slough and 88 % in Walker Salt Marsh (Fig. 7; Table S2). Thus, regardless of site, depth, or the presence of wrack, model results estimate no significant storage of seagrass-derived carbon in sediment (Table S2). Instead, C3 plants and/or diatoms are the primary significant contributors to underlying carbon storage across salt marshes in all locations.
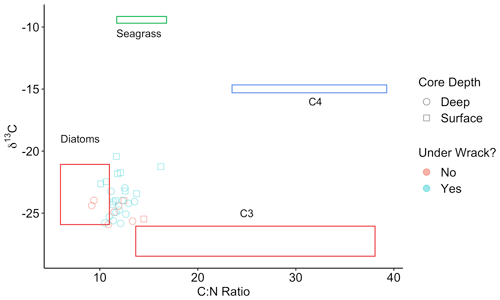
Figure 6Points represent the δ13C and C:N ratios of Walker Salt Marsh sediment data, collected from underneath persistent wrack lines (blue) and from areas free of wrack (red) (see Fig. 1c). Sediment subsamples collected from the surface to 10 cm deep are labeled as surface samples (square) and below 10 cm are labeled as deep samples (circle). Colored boxes represent sources (means ± SD) from diatoms (red), seagrass (green), C4 salt marsh plants (blue), and C3 salt marsh plants (red).
4.1 Carbon stocks
The lack of clear downcore trends (Fig. 2) could suggest relative OC stability with depth and through time or that there have been minimal changes to factors such as vegetation and grain size through time. The persistence of this pattern is supported by the longer cores sampled in seagrass and bare sediment sites (Fig. S3). One possible explanation for significant downcore OC losses in Newport Bay salt marsh is change in historic hydrography. Specifically, previous sediment profiles from this region also found finer surface sediments above coarser materials associated with the conversion from an erosional to a depositional system in the early 20th century due to urbanization of the surrounding area (Trimble, 2003). This change in grain size down core may drive the associated OC loss down core, discussed further below. Although we did not quantify sediment carbon accumulation rates here, previous work in Tomales Bay seagrass meadows indicate rates of 11.37–15.16 g C m−2 yr−1 via 210Pb dating (O'Donnell, 2017). By these accumulation rate estimates, we estimate the top 20 cm of sediment sampled here accumulated over approximately 100–130 years. Given the relatively slow accumulation rates and lack of down core trends, we interpret that our data represent realistic stock assessments for each respective habitat and location rather than being reflective of shallow surface sediment carbon stocks.
Determining drivers of OC storage variation across habitats is notably complex, in which a mix of factors such as grain size, elevation, hydrodynamic energy, and vegetation type may influence underlying sediment OC storage (Kelleway et al., 2016; Lima et al., 2020; Miyajima et al., 2015). Here, sediment grain size and its associated drivers allow us to further examine variation in sediment OC storage variation. Specifically, grain size likely contributed to the observed inter-habitat differences in OC storage given its strong correlation with TOM. Despite no significant differences in grain size between habitat types within each site (likely due to high grain size variability and low relative sample sizes), apparent qualitative differences allude to possible explanations of stock differences. For example, seagrass sediments had a lower percent mud than salt marsh sediments in Tomales Bay, a trend that was reversed in Newport Bay (Fig. 5a; Table S1). Similarly, seagrass sediments did not always contain a higher percent mud than neighboring bare sediments, contrary to what is typically reported (Bos et al., 2007; Conley et al., 2017; Mazarassa et al., 2015), which may explain their apparent lack of sediment OC differences. Overall, salt marshes had greater OC storage and smaller average grain size than seagrass meadows. The periodic, lower energy inundation of the sampled marsh and pan habitats may facilitate the observed smaller grain sizes by preventing the resuspension of small particles that might occur in higher hydrodynamic energy or submerged systems (Christiansen et al., 2000; Yang et al., 2008).
In marsh and pan habitats, biological factors such as primary production and decomposition rates may become more important drivers of carbon burial than would be true in dominantly sandy sediments (Miyajima et al., 2017). It is possible that root systems of overlying vegetation add bulk organic material into available sediment space, contributing to increased carbon deposition and decreased space for mineral accumulation (e.g., Rogers et al., 2019). Yet despite the absence of vegetation in pan sites, we did not observe significant differences in carbon stocks between pan sediments and surrounding salt marsh sediments. This could be attributed to (1) significant carbon contributions from surrounding salt marsh biomass to pan sites overtime, (2) historical marsh recovery along pan edges and subsequent burial of vegetation captured in pan cores, (3) variable decomposition rates in both pan and salt marsh sediments, or (4) the expected effect of canopy vegetation on salt marsh sediment being too small relative to other drivers, producing statistically insignificant results. In sum, although seagrasses and salt marshes can facilitate carbon storage by altering grain size distributions, hydrodynamics and geomorphology play a critical role in grain size distributions and therefore carbon storage. The hydrographic changes in Newport Bay salt marshes associated with decreased grain sizes further emphasize this point, drawing attention to the importance of watershed and sediment management to regional carbon storage. Furthermore, although grain size is clearly a key driver in carbon storage, our data demonstrate that it becomes of limited use as a predictor of carbon stocks after the proportion of mud exceeds 82 % (Fig. 4). This demonstrates that using grain size as a cost-effective way to estimate carbon stocks (as has been suggested, for example, by Serrano et al., 2016) only appears possible in sandy and mixed grain size sediments within the sites studied here. Carbon stocks in fine sediment sites above this threshold cannot be estimated using grain size alone – a management-relevant finding for efforts to incorporate habitat-specific carbon storage into regional climate plans.
Table 3Summary of previously reported carbon stocks in seagrass meadows and tidal wetlands, all normalized to the top 1 m of sediment. Studies that included any data from California are denoted by a. Values are reported as mean ± SE, unless otherwise noted. b Fourqurean et al. (2012) value represents median OC storage.
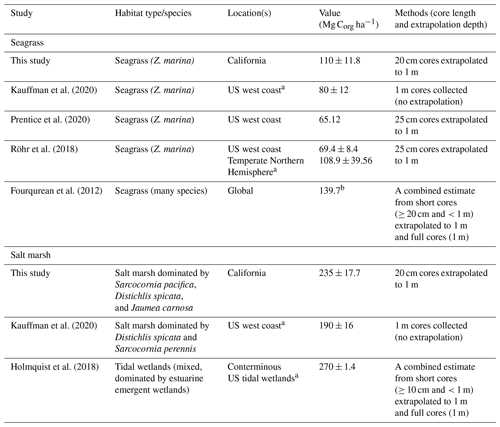
4.2 Global and regional comparisons
With recent reviews on coastal carbon stocks in salt marshes and seagrass meadows, we can compare our data to global and regional averages (no sufficient syntheses of pan or bare sediment carbons stocks were identified). We find that carbon storage in California seagrass sediments studied here is lower than global estimates yet is higher than regional estimates of Z. marina from the US west coast (Table 3). Early estimates from the North American west coast were markedly low, with one study of Z. marina from British Columbia measuring average stocks of 1.34 kg C m−3 with a maximum OC of 1.3 %, compared to the 11.01 ± 1.18 kg C m−3 and a maximum OC of 7.0 % estimated here (Postlethwaite et al., 2018). While estimates from studies averaging over broader US west coast regions are higher than this Canadian case study (Table 3), the data presented here are still higher than regional estimates, suggesting a possible latitudinal difference in carbon storage between the northern and southern regions of the North American west coast. This is not surprising given the variation in environmental and geomorphic conditions over this large stretch of coastline, which likely influence the carbon storage capacity, along with the factors discussed here such as hydrodynamics or grain size conditions.
Recent studies of the US west coast and of conterminous US tidal wetlands allow for the comparison with salt marsh data collected here. Much like with seagrass meadows, California salt marsh carbon storage was also higher than previous estimates from the US west coast (Kauffman et al., 2020; Table 3). Both the data presented here and those from Kauffman et al. (2020) (Table 3) were collected from similar marsh elevation zones and species compositions, reducing some potential for these factors to result in the observed differences in carbon stocks. Previous work has found that high-marsh-zone sediments are likely to contain greater OC than mid- and lower-marsh zones and that less inundation can facilitate increased root productivity and increased OC (the sites presented here would be considered “lower-marsh zones”) (Blum, 1993; Connor et al., 2001; Zhou et al., 2007). Moreover, sediments beneath other common marsh species in this region (e.g., Spartina sp.) were not sampled yet may have differing productivity levels, resulting in OC stock differences. This may contribute to the variation between our estimated salt marsh carbon stock and that of conterminous US tidal wetlands (Holmquist et al., 2018), which includes a variety of habitat types including forested and shrub-dominated tidal wetlands.
4.3 Carbon sources and exchanges
Across both salt marshes assessed in this study, diatoms and/or C3 plants were the dominant carbon sources in sediment. Although the isotopic values resulting from C4 and seagrass sources are poorly resolved, the lack of significant values from either source allows for interpretation. Specifically, seagrasses do not significantly contribute to any OC sources in sediment, while C4 plants may contribute minimally to OC in sediment in Elkhorn Slough (1.1 %–11.9 %; Table S2). Thus, despite similar isotopic values between seagrass and C4 plants, if seagrass-derived organic matter was buried under wrack sediments, we would expect to see higher δ13C values in wrack sediments with depth. The insignificant differences in δ13C between wrack and non-wrack sediments suggest that seagrass is not ultimately being buried, but rather carbon derived from C3 plants and diatoms are ultimately retained in sediment.
Several underlying causes may lead to this high proportion of diatom and/or C3-derived carbon (Fig. 7). The recalcitrance of both autochthonous and allochthonous material in sediment can vary depending on the material's composition (e.g., Burdige, 2007), and thus knowing the sources and composition of deposited material aids in understanding its chance of long-term burial. In seagrass meadows, previous work demonstrated that seagrass BGB likely contributes most to autochthonous carbon burial when compared to AGB due to higher proportions of refractory compounds and decreased grazing pressure (Trevathan-Tackett et al., 2017). This may serve to explain our lack of seagrass signal in salt marsh sediments under wrack. Seagrass wrack material deposited on top of the salt marsh is predominantly AGB, likely breaking down or being transported elsewhere before any appreciable portion reaches long-term sediment carbon pools. Instead, the dominant C3 signal is likely driven by the presence of pickleweed species (Sarcocornia sp.), which have a considerable portion of belowground biomass. Despite the presence of salt grass (Distichlis spicata), the relative lack of C4-derived carbon in sediment may be due to a few potential factors: (1) salt grass is less common relative to pickleweed within our sites, (2) based on our field observations, root systems of salt grass do not seem to penetrate as deeply or contain as much BGB as those of pickleweed, and (3) salt grass contains less woody tissue than pickleweed, making it less refractory (Jepson Flora Project, 2020). In the case of diatom and planktonic sources, their significant presence in sediment may be due to a greater abundance overall or due to complex preservation pathways such as the facilitation of carbon burial by binding sediments through extracellular polymeric substances (Drexler et al., 2020; Macreadie et al., 2019; Oakes and Eyre, 2013).
From the seasonal senescence indicated by seagrass meadow biomass data (Table 2), we infer that a significant amount of seagrass AGB is either degraded within the meadow or exported for remineralization or deposition elsewhere. Given that strong tidal flows can occur within meadows and that high densities of seagrass wrack were observed in a neighboring salt marsh, lateral export of AGB from the meadow is likely. Nonetheless, the majority of OC deposited into the marsh in the form of seagrass wrack is likely remineralized over the course of the year – with wrack decomposition outpacing a sediment accumulation rate that might support carbon preservation. While wrack remineralization can support local estuarine food web metabolism, it can also produce emissions (Jiménez et al., 2017; Liu et al., 2019). However, these emissions are small when compared to the levels of carbon sequestration within the marsh – if all of the seagrass wrack along the wrack lines was remineralized annually, this degradation would only contribute 33 ± 7.8 g C m−2 yr−1 to the atmosphere (Table 2). For comparison, dating (primarily 137Cs) from salt marshes nearby estimates carbon accumulation as 174 ± 45 g C m−2 yr−1 (Ouyang and Lee, 2014). Thus, even along wrack lines, carbon accumulation far outpaces carbon release from the breakdown of allochthonous material. Although estimates of decomposition and accumulation rates in this site could further inform these concepts, the slow annual cycle of seagrass wrack deposition and disappearance observed in the aerial imagery (Fig. S2) and the lack of seagrass-derived carbon in underlying sediment make a compelling case that little of this material is ultimately buried.
We find that California salt marshes can store approximately twice as much carbon as seagrass meadows do within this region. Grain size – an easier metric to quantify than carbon stock – can be used to estimate regional carbon storage in sandy and mixed grain size sediments. This information can serve to inform local and regional management plans in efforts to prioritize and quantify carbon storage across these habitat types. While seagrass meadows may act as local sinks for carbon, they also export substantial amounts of AGB annually, which can be remineralized and converted to CO2 rather than being buried in neighboring blue carbon habitats. C3 salt marsh plants and/or diatoms contributed to the OC pools in all three salt marshes under study likely due to their prevalence within our sites and their resistance to degradation. This comprehensive study assesses several key research needs in blue carbon science informing current efforts to prioritize and quantify carbon storage across these habitat types.
All data included in this manuscript will be made publicly available on the Dryad data repository (https://doi.org/10.5061/dryad.m0cfxpp31, last access: 5 July 2021, Ward, 2021).
The supplement related to this article is available online at: https://doi.org/10.5194/bg-18-4717-2021-supplement.
MAW contributed to the conceptualization, investigation, methodology, writing (original draft preparation), and formal analysis. TMH contributed to the conceptualization, funding support, and writing (review and editing). CS contributed to the investigation (field and lab support) and writing (review and editing). TF contributed to the investigation (field and lab support) and writing (review and editing). AMR contributed to the methodology, investigation, and writing (review and editing). SM contributed to the investigation (field and lab support) and writing (review and editing). LRC contributed to the writing (review and editing). BCO contributed to the investigation (field and lab support), methodology, and writing (review and editing). KE contributed to the investigation (field and lab support) and writing (review and editing). WCO contributed to the writing (review and editing). KMB contributed to the investigation (field and lab support) and writing (review and editing).
The authors declare that they have no conflict of interest.
Publisher’s note: Copernicus Publications remains neutral with regard to jurisdictional claims in published maps and institutional affiliations.
We acknowledge Al Carranza, Grant Susner, Amanda Nordstrom, Siena Watson, Camille Frias, Daphne Bradley, Anya Morrill, Natalie Rossi, and Jezebel Powers for laboratory and field assistance. This work was supported by California Sea Grant (R/HCME-03, support to Tessa Hill, Brian Gaylord, Eric Sanford, and Kristy Kroeker), the California Ocean Protection Council (support to Tessa Hill, Brian Gaylord, Eric Sanford, and Kristy Kroeker), the CSU Council on Ocean Affairs, Science and Technology (support to Melissa Ward), and the San Diego ARCS foundation (support to Melissa Ward). Jim Cloern also generously provided data to support isotopic mixing models. We also thank Audubon Canyon Ranch for site access from their Cypress Grove Research Center. Lastly, we would like to thank Peter Bowler, whose mentorship and legacy in wetland conservation has sparked lifelong collaborations and scientific careers.
This research has been supported by California Sea Grant (grant no. R/HCME-03).
This paper was edited by Tyler Cyronak and reviewed by Toshihiro Miyajima and Fernanda Adame.
Alongi, D. M.: Blue carbon coastal sequestration for climate change mitigation. Springer International Publishing, Briefs in Climate Studies, https://doi.org/10.1007/978-3-319-91698-9, 2018.
Attard, K. M., Rodil, I. F., Berg, P., Norkko, J., Norkko, A., and Glud, R. N.: Seasonal metabolism and carbon export potential of a key coastal habitat: The perennial canopy-forming macroalga Fucus vesiculosus, Limnol. Ocean., 64, 149–164, https://doi.org/10.1002/lno.11026, 2019.
Benner, R., Fogel, M. L., and Sprague, E. K.: Diagenesis of belowground biomass of Spartina alterniflora in salt-marsh sediments, Limnol. Ocean., 36, 1358–1374, https://doi.org/10.4319/lo.1991.36.7.1358, 1991.
Blum, L. K.: Spartina alterniflora root dynamics in a Virginia marsh, Mar. Ecol. Prog. Ser., 102, 169–178, 1993.
Bos, A. R., Bouma, T. J., de Kort, G. L. J., and van Katwijk, M. M.: Ecosystem engineering by annual intertidal seagrass beds: Sediment accretion and modification, Estuar. Coast. Shelf Sci., 74, 344–348, https://doi.org/10.1016/j.ecss.2007.04.006, 2007.
Bouillon, S. and Connolly, R. M.: Carbon Exchange Among Tropical Coastal Ecosystems, in: Ecological Connectivity among Tropical Coastal Ecosystems, edited by: Nagelkerken, I., Springer Netherlands, Dordrecht, 45–70, 2009.
Brevik, E. C. and Homburg, J. A.: A 5000 year record of carbon sequestration from a coastal lagoon and wetland complex, Southern California, USA, CATENA, 57, 221–232, https://doi.org/10.1016/j.catena.2003.12.001, 2004.
Burdige, D. J.: Preservation of Organic Matter in Marine Sediments: Controls, Mechanisms, and an Imbalance in Sediment Organic Carbon Budgets?, Chem. Rev., 107, 467–485, https://doi.org/10.1021/cr050347q, 2007.
Cabello-Pasini, A., Muñiz-Salazar, R., and Ward, D. H.: Annual variations of biomass and photosynthesis in Zostera marina at its southern end of distribution in the North Pacific, Aquat. Bot., 76, 31–47, https://doi.org/10.1016/S0304-3770(03)00012-3, 2003.
Callaway, J. C., Borgnis, E. L., Turner, R. E., and Milan, C. S.: Carbon Sequestration and Sediment Accretion in San Francisco Bay Tidal Wetlands, Estuar. Coast., 35, 1163–1181, https://doi.org/10.1007/s12237-012-9508-9, 2012.
Capece, L.: The origin of sedimentary organic carbon in temperate seagrass meadows in California estuaries, Thesis 22619435, University of California, Davis, ProQuest Dissertations Publishing, 2019.
Chmura, G. L., Anisfeld, S. C., Cahoon, D. R., and Lynch, J. C.: Global carbon sequestration in tidal, saline wetland soils, Global Biogeochem. Cy., 17, 1111, https://doi.org/10.1029/2002GB001917, 2003.
Christiansen, T., Wiberg, P. L., and Milligan, T. G.: Flow and sediment transport on a tidal salt marsh surface, Estuarine, Coast. Shelf Sci., 50, 315–331, https://doi.org/10.1006/ecss.2000.0548, 2000.
Cloern, J. E., Canuel, E. A., and Harris, D.: Stable carbon and nitrogen isotope composition of aquatic and terrestrial plants of the San Francisco Bay estuarine system, Limnol. Ocean., 47, 713–729, https://doi.org/10.4319/lo.2002.47.3.0713, 2002.
Conley, D. C., Austin, M., Davidson, I., Buscombe, D., and Masselink, G.: Grain size selection in seagrass beds, Coast. Dynam., 11, 200, 2017.
Connor, R. F., Chmura, G. L., and Beecher, C. B.: Carbon accumulation in bay of fundy salt marshes: Implications for restoration of reclaimed marshes, Global Biogeochem. Cy., 15, 943–954, https://doi.org/10.1029/2000GB001346, 2001.
Craft, C. B., Seneca, E. D., and Broome, S. W.: Loss on Ignition and Kjeldahl Digestion for Estimating Organic Carbon and Total Nitrogen in Estuarine Marsh Soils: Calibration with Dry Combustion, Estuaries, 14, 175, https://doi.org/10.2307/1351691, 1991.
Craven, K. F., Edwards, R. J., and Flood, R. P.: Source organic matter analysis of saltmarsh sediments using SIAR and its application in relative sea-level studies in regions of C4 plant invasion, Boreas, 46, 642–654, https://doi.org/10.1111/bor.12245, 2017.
Cyronak, T., Andersson, A. J., D'Angelo, S., Bresnahan, P., Davidson, C., Griffin, A., Kindeberg, T., Pennise, J., Takeshita, Y., and White, M.: Short-Term Spatial and Temporal Carbonate Chemistry Variability in Two Contrasting Seagrass Meadows: Implications for pH Buffering Capacities, Estuar. Coast., 41, 1282–1296, https://doi.org/10.1007/s12237-017-0356-5, 2018.
Dahl, M., Deyanova, D., Gütschow, S., Asplund, M. E., Lyimo, L. D., Karamfilov, V., Santos, R., Björk, M., and Gullström, M.: Sediment properties as important predictors of carbon storage in Zostera marina meadows: A Comparison of four European areas, PLoS ONE, 11, e0167493, https://doi.org/10.1371/journal.pone.0167493, 2016.
Dean, W. E.: Determination of carbonate and organic matter in calcareous sediments and sedimentary rocks by loss on ignition; comparison with other methods, J. Sediment. Res., 44, 242–248, https://doi.org/10.1306/74D729D2-2B21-11D7-8648000102C1865D, 1974.
Drexler, J. Z., Davis, M. J., Woo, I., and De La Cruz, S.: Carbon sources in the sediments of a restoring vs. historically unaltered salt marsh, Estuar. Coast., 43, 1345–1360, https://doi.org/10.1007/s12237-020-00748-7, 2020.
Duarte, C. M. and Cebrián, J.: The fate of marine autotrophic production, Limnol. Ocean., 41, 1758–1766, https://doi.org/10.4319/lo.1996.41.8.1758, 1996.
Duarte, C. M. and Krause-Jensen, D.: Export from seagrass mead- 95 ows contributes to marine carbon sequestration, Front. Mar. Sci., 4, https://doi.org/10.3389/fmars.2017.00013, 2017.
Duarte, C. M., Marbà, N., Gacia, E., Fourqurean, J. W., Beggins, J., Barrón, C., and Apostolaki, E. T.: Seagrass community metabolism: Assessing the carbon sink capacity of seagrass meadows, Global Biogeochem. Cy., 24, GB4032, https://doi.org/10.1029/2010GB003793, 2010.
Escapa, M., Perillo, G. M. E., and Iribarne, O.: Biogeomorphically driven salt pan formation in Sarcocornia-dominated salt-marshes, Geomorphology, 228, 147–157, https://doi.org/10.1016/j.geomorph.2014.08.032, 2015.
Ewers Lewis, C. J., Young, M. A., Ierodiaconou, D., Baldock, J. A., Hawke, B., Sanderman, J., Carnell, P. E., and Macreadie, P. I.: Drivers and modelling of blue carbon stock variability in sediments of southeastern Australia, Biogeosciences, 17, 2041–2059, https://doi.org/10.5194/bg-17-2041-2020, 2020.
Fourqurean, J. W., Moore, T. O., Fry, B., and Hollibaugh, J. T.: Spatial and temporal variation in ratios, δ15N, and δ13C of eelgrass Zostera marina as indicators of ecosystem processes, Tomales Bay, California, USA, 157, 147–157, https://doi.org/10.3354/meps157147, 1997.
Fourqurean, J. W., Duarte, C. M., Kennedy, H., Marbà, N., Holmer, M., Mateo, M. A., Apostolaki, E. T., Kendrick, G. A., Krause-Jensen, D., McGlathery, K. J., and Serrano, O.: Seagrass ecosystems as a globally significant carbon stock, Nat. Geosci., 5, 505–509, https://doi.org/10.1038/ngeo1477, 2012.
Freedman, B., Stinson, G., and Lacoul, P.: Carbon credits and the conservation of natural areas, Environ. Rev., 17, 1–19, https://doi.org/10.1139/A08-007, 2009.
Gambi, M., Nowell, A., and Jumars, P.: Flume observations on flow dynamics in Zostera marina (eelgrass) beds, Mar. Ecol. Prog. Ser., 61, 159–169, https://doi.org/10.3354/meps061159, 1990.
Google Earth: Tomales Bay, California, USA. 38∘12′42.4′′ N 122∘55′39.7′′ W, 2020.
Greiner, J. T., McGlathery, K. J., Gunnell, J., and McKee, B. A.: Seagrass restoration enhances “Blue Carbon” sequestration in coastal waters, PLoS ONE, 8, e72469, https://doi.org/10.1371/journal.pone.0072469, 2013.
Green, E. P. and Short, F. T. (Eds.): World Atlas of Seagrasses, University of California Press, Berkeley, USA, 324 pp., 2003.
Hendriks, I., Sintes, T., Bouma, T., and Duarte, C.: Experimental assessment and modeling evaluation of the effects of the seagrass Posidonia oceanica on flow and particle trapping, Mar. Ecol. Prog. Ser., 356, 163–173, https://doi.org/10.3354/meps07316, 2008.
Holmquist, J. R., Windham-Myers, L., Bliss, N., Crooks, S., Morris, J. T., Megonigal, J. P., Troxler, T., Weller, D., Callaway, J., Drexler, J., Ferner, M. C., Gonneea, M. E., Kroeger, K. D., Schile-Beers, L., Woo, I., Buffington, K., Breithaupt, J., Boyd, B. M., Brown, L. N., Dix, N., Hice, L., Horton, B. P., MacDonald, G. M., Moyer, R. P., Reay, W., Shaw, T., Smith, E., Smoak, J. M., Sommerfield, C., Thorne, K., Velinsky, D., Watson, E., Grimes, K. W., and Woodrey, M.: accuracy and precision of tidal wetland soil carbon mapping in the conterminous United States, Sci. Rep., 8, 9478, https://doi.org/10.1038/s41598-018-26948-7, 2018.
Howard, J., Hoyt, S., Isensee, K., Pidgeon, E., and Telszewski, M. (Eds.): Coastal Blue Carbon: Methods for assessing carbon stocks and emissions factors in mangroves, tidal salt marshes, and seagrass meadows. Conservation International, Intergovernmental Oceanographic Commission of UNESCO, International Union for Conservation of Nature, Arlington, Virginia, USA, 48–50, 2014.
Hyndes, G. A., Nagelkerken, I., McLeod, R. J., Connolly, R. M., Lavery, P. S., and Vanderklift, M. A.: Mechanisms and ecological role of carbon transfer within coastal seascapes, Biolog. Rev., 89, 232–254, https://doi.org/10.1111/brv.12055, 2014.
Ince, R., Hyndes, G. A., Lavery, P. S., and Vanderklift, M. A.: Marine macrophytes directly enhance abundances of sandy beach fauna through provision of food and habitat, Estuar. Coast. Shelf Sci., 74, 77–86, https://doi.org/10.1016/j.ecss.2007.03.029, 2007.
Jepson Flora Project: Jepson eFlora, available at: https://ucjeps.berkeley.edu/eflora/, last access: 5 February 2020.
Jiménez, M. A., Beltran, R., Traveset, A., Calleja, M. L., Delgado-Huertas, A., and Marbà, N.: Aeolian transport of seagrass (Posidonia oceanica) beach-cast to terrestrial systems, Estuar. Coast. Shelf Sci., 196, 31–44, https://doi.org/10.1016/j.ecss.2017.06.035, 2017.
Johannessen, S. C. and Macdonald, R. W.: Geoengineering with seagrasses: is credit due where credit is given?, Environ. Res. Lett., 11, 113001, https://doi.org/10.1088/1748-9326/11/11/113001, 2016.
Kauffman, J. B., Giovanonni, L., Kelly, J., Dunstan, N., Borde, A., Diefenderfer, H., Cornu, C., Janousek, C., Apple, J., and Brophy, L.: Total ecosystem carbon stocks at the marine-terrestrial interface: Blue carbon of the Pacific Northwest Coast, United States, Glob. Change Biol., 26, 5679–5692, https://doi.org/10.1111/gcb.15248, 2020.
Kelleway, J. J., Saintilan, N., Macreadie, P. I., and Ralph, P. J.: Sedimentary factors are key predictors of carbon storage in SE australian saltmarshes, Ecosystems, 19, 865–880, https://doi.org/10.1007/s10021-016-9972-3, 2016.
Kennedy, H., Beggins, J., Duarte, C. M., Fourqurean, J. W., Holmer, M., Marbà, N., and Middelburg, J. J.: Seagrass sediments as a global carbon sink: Isotopic constraints, Global Biogeochem. Cy., 24, GB4026, https://doi.org/10.1029/2010GB003848, 2010.
Largier, J. L., Hollibaugh, J. T., and Smith, S. V.: Seasonally hypersaline estuaries in mediterranean-climate regions, Estuar. Coast. Shelf Sci., 45, 789–797, https://doi.org/10.1006/ecss.1997.0279, 1997.
Lavery, P. S., Mateo, M.-Á., Serrano, O., and Rozaimi, M.: Variability in the carbon storage of seagrass habitats and its implications for global estimates of Blue Carbon ecosystem service, PLoS ONE, 8, e73748, https://doi.org/10.1371/journal.pone.0073748, 2013.
Leorri, E., Zimmerman, A. R., Mitra, S., Christian, R. R., Fatela, F., and Mallinson, D. J.: Refractory organic matter in coastal salt marshes-effect on C sequestration calculations, Sci. Tot. Environ., 633, 391–398, https://doi.org/10.1016/j.scitotenv.2018.03.120, 2018.
Lima, M., Do, A. C., Ward, R. D., and Joyce, C. B.: Environmental drivers of sediment carbon storage in temperate seagrass meadows, Hydrobiologia, 847, 1773–1792, https://doi.org/10.1007/s10750-019-04153-5, 2020.
Liu, S., Trevathan-Tackett, S. M., Ewers Lewis, C. J., Ollivier, Q. R., Jiang, Z., Huang, X., and Macreadie, P. I.: Beach-cast seagrass wrack contributes substantially to global greenhouse gas emissions, J. Environ. Manage., 231, 329–335, https://doi.org/10.1016/j.jenvman.2018.10.047, 2019.
Lovelock, C. E., Adame, M. F., Bennion, V., Hayes, M., O'Mara, J., Reef, R., and Santini, N. S.: Contemporary rates of carbon sequestration through vertical accretion of sediments in mangrove forests and saltmarshes of South East Queensland, Australia, Estuar. Coast., 37, 763–771, https://doi.org/10.1007/s12237-013-9702-4, 2014.
Lovelock, C. E., Atwood, T., Baldock, J., Duarte, C. M., Hickey, S., Lavery, P. S., Masque, P., Macreadie, P. I., Ricart, A. M., Serrano, O., and Steven, A.: Assessing the risk of carbon dioxide emissions from blue carbon ecosystems, Front. Ecol. Environ., 15, 257–265, https://doi.org/10.1002/fee.1491, 2017.
Lovelock, C. E. and Duarte, C. M.: Dimensions of Blue Carbon and emerging perspectives, Biol. Lett., 15, 20180781, https://doi.org/10.1098/rsbl.2018.0781, 2019.
Macreadie, P. I., Ewers-Lewis, C. J., Whitt, A. A., Ollivier, Q., Trevathan-Tackett, S. M., Carnell, P., and Serrano, O.: Comment on “Geoengineering with seagrasses: is credit due where credit is given?”, Environ. Res. Lett., 13, 028002, https://doi.org/10.1088/1748-9326/aaa7ad, 2018.
Macreadie, P. I., Anton, A., Raven, J. A., Beaumont, N., Connolly, R. M., Friess, D. A., Kelleway, J. J., Kennedy, H., Kuwae, T., Lavery, P. S., Lovelock, C. E., Smale, D. A., Apostolaki, E. T., Atwood, T. B., Baldock, J., Bianchi, T. S., Chmura, G. L., Eyre, B. D., Fourqurean, J. W., Hall-Spencer, J. M., Huxham, M., Hendriks, I. E., Krause-Jensen, D., Laffoley, D., Luisetti, T., Marbà, N., Masque, P., McGlathery, K. J., Megonigal, J. P., Murdiyarso, D., Russell, B. D., Santos, R., Serrano, O., Silliman, B. R., Watanabe, K., and Duarte, C. M.: The future of Blue Carbon science, Nat. Commun., 10, 1–13, https://doi.org/10.1038/s41467-019-11693-w, 2019.
Mazarrasa, I., Marbà, N., Lovelock, C. E., Serrano, O., Lavery, P. S., Fourqurean, J. W., Kennedy, H., Mateo, M. A., Krause-Jensen, D., Steven, A. D. L., and Duarte, C. M.: Seagrass meadows as a globally significant carbonate reservoir, Biogeosciences, 12, 4993–5003, https://doi.org/10.5194/bg-12-4993-2015, 2015.
Mazarrasa, I., Samper-Villarreal, J., Serrano, O., Lavery, P. S., Lovelock, C. E., Marbà, N., Duarte, C. M., and Cortés, J.: Habitat characteristics provide insights of carbon storage in seagrass meadows, Mar. Pollut. Bull., 134, 106–117, https://doi.org/10.1016/j.marpolbul.2018.01.059, 2018.
Mcleod, E., Chmura, G. L., Bouillon, S., Salm, R., Björk, M., Duarte, C. M., Lovelock, C. E., Schlesinger, W. H., and Silliman, B. R.: A blueprint for blue carbon: toward an improved understanding of the role of vegetated coastal habitats in sequestering CO2, Front. Ecol. Environ., 9, 552–560, https://doi.org/10.1890/110004, 2011.
Merkel and Associates: Inc. 2017 Tomales Bay Eelgrass Inventory, prepared the National Oceanic Atmospheric Administration (NOAA) Greater Farallones National Marine Sanctuary, December, 2017.
Milliman, J. D.: Precipitation and Cementation of Deep-Sea Carbonate Sediments, in: Deep-Sea Sediments: Physical and Mechanical Properties, edited by: Inderbitzen, A. L., Springer US, Boston, MA., 463–476, 1974.
Miyajima, T., Hori, M., Hamaguchi, M., Shimabukuro, H., Adachi, H., Yamano, H., and Nakaoka, M.: Geographic variability in organic carbon stock and accumulation rate in sediments of East and Southeast Asian seagrass meadows, Global Biogeochem. Cy., 29, 397–415, https://doi.org/10.1002/2014GB004979, 2015.
Miyajima, T., Hori, M., Hamaguchi, M., Shimabukuro, H., and Yoshida, G.: Geophysical constraints for organic carbon sequestration capacity of Zostera marina seagrass meadows and surrounding habitats, 62, 954–972, https://doi.org/10.1002/lno.10478, 2017.
Nahlik, A. M. and Fennessy, M. S.: Carbon storage in US wetlands, Nat. Commun., 7, 13835, https://doi.org/10.1038/ncomms13835, 2016.
O'Donnell, B. C.: Carbon sequestration within Northeastern Pacific seagrass meadows, Thesis 10607483, University of California, Davis, ProQuest Dissertations Publishing, 1–83, 2017.
Oakes, J. M. and Eyre, B. D.: Transformation and fate of microphytobenthos carbon in subtropical, intertidal sediments: potential for long-term carbon retention revealed by 13C-labeling, Biogeosciences, 11, 1927–1940, https://doi.org/10.5194/bg-11-1927-2014, 2014.
Ouyang, X. and Lee, S. Y.: Updated estimates of carbon accumulation rates in coastal marsh sediments, Biogeosciences, 11, 5057–5071, https://doi.org/10.5194/bg-11-5057-2014, 2014.
Parnell, A. C. and Jackson, A. L.: SIAR: stable isotope analysis in R. R package version 4.2., available at: http://CRAN.R-project.org/package=siar (last access: 2 July 2021), 2013.
Patrick, W. H. and DeLaune, R. D.: Subsidence accretion and sea level rise in south San Francisco Bay marshes, Limnol. Ocean., 35, 1389–1395, https://doi.org/10.4319/lo.1990.35.6.1389, 1990.
Peck, E. K., Wheatcroft, R. A., and Brophy, L. S.: Controls on Sediment Accretion and Blue Carbon Burial in Tidal Saline Wetlands: Insights From the Oregon Coast, USA, J. Geophys. Res.-Biogeo., 125, e2019JG005464, https://doi.org/10.1029/2019JG005464, 2020.
Pendleton, L., Donato, D. C., Murray, B. C., Crooks, S., Jenkins, W. A., Sifleet, S., Craft, C., Fourqurean, J. W., Kauffman, J. B., Marbà, N., Megonigal, P., Pidgeon, E., Herr, D., Gordon, D., and Baldera, A.: Estimating global “Blue Carbon” emissions from conversion and degradation of vegetated coastal ecosystems, edited by: Thrush, S., PLoS ONE, 7, e43542, https://doi.org/10.1371/journal.pone.0043542, 2012.
Perdue, E. M. and Koprivnjak, J. F.: Using the C/N ratio to estimate terrigenous inputs of organic matter to aquatic environments, Estuar. Coast. Shelf Sci., 73, 65–72, https://doi.org/10.1016/j.ecss.2006.12.021, 2007.
Poppe, K. L. and Rybczyk, J. M.: Carbon Sequestration in a Pacific Northwest Eelgrass (Zostera marina) Meadow, BioOne, 92, 80–91, https://doi.org/10.3955/046.092.0202, 2018.
Postlethwaite, V. R., McGowan, A. E., Kohfeld, K. E., Robinson, C. L. K., and Pellatt, M. G.: Low blue carbon storage in eelgrass (Zostera marina) meadows on the Pacific Coast of Canada, PLOS ONE, 13, e0198348, https://doi.org/10.1371/journal.pone.0198348, 2018.
Prentice, C., Hessing-Lewis, M., Sanders-Smith, R., and Salomon, A. K.: Reduced water motion enhances organic carbon stocks in temperate eelgrass meadows, Limnol. Ocean., 64, 2389–2404, https://doi.org/10.1002/lno.11191, 2019.
Prentice, C., Poppe, K. L., Lutz, M., Murray, E., Stephens, T. A., Spooner, A., Hessing-Lewis, M., Sanders-Smith, R., Rybczyk, J. M., Apple, J., Short, F. T., Gaeckle, J., Helms, A., Mattson, C., Raymond, W. W., and Klinger, T.: A Synthesis of Blue Carbon Stocks, Sources, and Accumulation Rates in Eelgrass (Zostera 100 marina) Meadows in the Northeast Pacific, Global Biogeochem. Cy., 34, e2019GB006345, https://doi.org/10.1029/2019GB006345, 2020.
R Core Team: R: A language and environment for statistical computing. R Foundation for Statistical Computing, Vienna, Austria, available at: https://www.R-project.org/ (last access: 2 July 2021), 2018.
Ricart, A., Dalmau, A., Pérez, M., and Romero, J.: Effects of landscape configuration on the exchange of materials in seagrass ecosystems, Mar. Ecol. Prog. Ser., 532, 89–100, https://doi.org/10.3354/meps11384, 2015.
Ricart, A. M., Pérez, M., and Romero, J.: Landscape configuration modulates carbon storage in seagrass sediments, Estuar. Coast. Shelf Sci., 185, 69–76, https://doi.org/10.1016/j.ecss.2016.12.011, 2017.
Ricart, A. M., York, P. H., Bryant, C. V., Rasheed, M. A., Ierodiaconou, D., and Macreadie, P. I.: High variability of Blue Carbon storage in seagrass meadows at the estuary scale, Sci. Rep., 10, 5865, https://doi.org/10.1038/s41598-020-62639-y, 2020.
Rogers, K., Kelleway, J. J., Saintilan, N., Megonigal, J. P., Adams, J. B., Holmquist, J. R., Lu, M., Schile-Beers, L., Zawadzki, A., Mazumder, D., and Woodroffe, C. D.: Wetland carbon storage controlled by millennial-scale variation in relative sea-level rise, Nature, 567, 91–95, https://doi.org/10.1038/s41586-019-0951-7, 2019.
Röhr, M. E., Holmer, M., Baum, J. K., Björk, M., Boyer, K., Chin, D., Chalifour, L., Cimon, S., Cusson, M., Dahl, M., Deyanova, D., Duffy, J. E., Eklöf, J. S., Geyer, J. K., Griffin, J. N., Gullström, M., Hereu, C. M., Hori, M., Hovel, K. A., Hughes, A. R., Jorgensen, P., Kiriakopolos, S., Moksnes, P.-O., Nakaoka, M., O'Connor, M. I., Peterson, B., Reiss, K., Reynolds, P. L., Rossi, F., Ruesink, J., Santos, R., Stachowicz, J. J., Tomas, F., Lee, K.-S., Unsworth, R. K. F., and Boström, C.: Blue carbon storage capacity of temperate Eelgrass (Zostera marina) meadows, Global Biogeochem. Cy., 32, 1457–1475, https://doi.org/10.1029/2018GB005941, 2018.
Saintilan, N., Rogers, K., Mazumder, D., and Woodroffe, C. Allochthonous and autochthonous contributions to carbon accumulation and carbon store in southeastern Australian coastal wetlands, Estuar. Coast. Shelf Sci., 128, 84–92, https://doi.org/10.1016/j.ecss.2013.05.010, 2013.
Schlosser, S. and Eicher, A.: The Humboldt Bay and Eel River Estuary Benthic Habitat Project, California Sea Grant Publication T-075, 246 p, 2012.
Serrano, O., Mateo, M. A., Renom, P., and Julià, R.: Characterization of soils beneath a Posidonia oceanica meadow, Geoderma, 185, 26–36, https://doi.org/10.1016/j.geoderma.2012.03.020, 2012.
Serrano, O., Lavery, P. S., Duarte, C. M., Kendrick, G. A., Calafat, A., York, P. H., Steven, A., and Macreadie, P. I.: Can mud (silt and clay) concentration be used to predict soil organic carbon content within seagrass ecosystems?, Biogeosciences, 13, 4915–4926, https://doi.org/10.5194/bg-13-4915-2016, 2016.
Serrano, O., Kelleway, J. J., Lovelock, C., and Lavery, P. S.: Conservation of Blue Carbon Ecosystems for Climate Change Mitigation and Adaptation, Coastal Wetlands, Elsevier., 965–996, 2019.
St. Laurent, K. A., Hribar, D. J., Carlson, A. J., Crawford, C. M., and Siok, D.: Assessing coastal carbon variability in two Delaware tidal marshes, J. Coast Conserv., 24, 65, https://doi.org/10.1007/s11852-020-00783-3, 2020.
Trevathan-Tackett, S. M., Macreadie, P. I., Sanderman, J., Baldock, J., Howes, J. M., and Ralph, P. J.: A Global Assessment of the Chemical Recalcitrance of Seagrass Tissues: Implications for Long-Term Carbon Sequestration, Front. Plant Sci., 8, 925, https://doi.org/10.3389/fpls.2017.00925, 2017.
Trimble, S. W.: Historical hydrographic and hydrologic changes in the San Diego creek watershed, Newport Bay, California, J. Historic. Geogr., 29, 422–444, https://doi.org/10.1006/jhge.2002.0485, 2003.
Valiela, I. and Cole, M. L.: Comparative evidence that salt marshes and mangroves may protect seagrass meadows from land-derived nitrogen loads, Ecosystems, 5, 92–102, https://doi.org/10.1007/s10021-001-0058-4, 2002.
Van Dyke, E. and Wasson, K.: Historical ecology of a central California estuary: 150 years of habitat change, Estuaries, 28, 173–189, https://doi.org/10.1007/BF02732853, 2005.
Ward, M. A.: Core data – Organic carbon, grain size, elemental/isotopic composition, Dryad, [data set], https://doi.org/10.5061/dryad.m0cfxpp31, last access: 5 July 2021.
Wilkinson, G. M., Besterman, A., Buelo, C., Gephart, J., and Pace, M. L.: A synthesis of modern organic carbon accumulation rates in coastal and aquatic inland ecosystems, Sci. Rep., 8, 15736, https://doi.org/10.1038/s41598-018-34126-y, 2018.
Yang, S. L., Li, H., Ysebaert, T., Bouma, T. J., Zhang, W. X., Wang, Y. Y., Li, P., Li, M., and Ding, P. X.: Spatial and temporal variations in sediment grain size in tidal wetlands, Yangtze Delta: On the role of physical and biotic controls, Estuar. Coast. Shelf Sci., 77, 657–671, https://doi.org/10.1016/j.ecss.2007.10.024, 2008.
Zhou, J., Wu, Y., Kang, Q., and Zhang, J.: Spatial variations of carbon, nitrogen, phosphorous and sulfur in the salt marsh sediments of the Yangtze Estuary in China, Estuar. Coast. Shelf Sci., 71, 47–59, https://doi.org/10.1016/j.ecss.2006.08.012, 2007