the Creative Commons Attribution 4.0 License.
the Creative Commons Attribution 4.0 License.
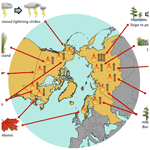
Reviews and syntheses: Arctic fire regimes and emissions in the 21st century
Juha Aalto
Ville-Veikko Paunu
Steve R. Arnold
Sabine Eckhardt
Zbigniew Klimont
Justin J. Fain
Nikolaos Evangeliou
Ari Venäläinen
Nadezhda M. Tchebakova
Elena I. Parfenova
Kaarle Kupiainen
Amber J. Soja
Lin Huang
Simon Wilson
In recent years, the pan-Arctic region has experienced increasingly extreme fire seasons. Fires in the northern high latitudes are driven by current and future climate change, lightning, fuel conditions, and human activity. In this context, conceptualizing and parameterizing current and future Arctic fire regimes will be important for fire and land management as well as understanding current and predicting future fire emissions. The objectives of this review were driven by policy questions identified by the Arctic Monitoring and Assessment Programme (AMAP) Working Group and posed to its Expert Group on Short-Lived Climate Forcers. This review synthesizes current understanding of the changing Arctic and boreal fire regimes, particularly as fire activity and its response to future climate change in the pan-Arctic have consequences for Arctic Council states aiming to mitigate and adapt to climate change in the north. The conclusions from our synthesis are the following. (1) Current and future Arctic fires, and the adjacent boreal region, are driven by natural (i.e. lightning) and human-caused ignition sources, including fires caused by timber and energy extraction, prescribed burning for landscape management, and tourism activities. Little is published in the scientific literature about cultural burning by Indigenous populations across the pan-Arctic, and questions remain on the source of ignitions above 70∘ N in Arctic Russia. (2) Climate change is expected to make Arctic fires more likely by increasing the likelihood of extreme fire weather, increased lightning activity, and drier vegetative and ground fuel conditions. (3) To some extent, shifting agricultural land use and forest transitions from forest–steppe to steppe, tundra to taiga, and coniferous to deciduous in a warmer climate may increase and decrease open biomass burning, depending on land use in addition to climate-driven biome shifts. However, at the country and landscape scales, these relationships are not well established. (4) Current black carbon and PM2.5 emissions from wildfires above 50 and 65∘ N are larger than emissions from the anthropogenic sectors of residential combustion, transportation, and flaring. Wildfire emissions have increased from 2010 to 2020, particularly above 60∘ N, with 56 % of black carbon emissions above 65∘ N in 2020 attributed to open biomass burning – indicating how extreme the 2020 wildfire season was and how severe future Arctic wildfire seasons can potentially be. (5) What works in the boreal zones to prevent and fight wildfires may not work in the Arctic. Fire management will need to adapt to a changing climate, economic development, the Indigenous and local communities, and fragile northern ecosystems, including permafrost and peatlands. (6) Factors contributing to the uncertainty of predicting and quantifying future Arctic fire regimes include underestimation of Arctic fires by satellite systems, lack of agreement between Earth observations and official statistics, and still needed refinements of location, conditions, and previous fire return intervals on peat and permafrost landscapes. This review highlights that much research is needed in order to understand the local and regional impacts of the changing Arctic fire regime on emissions and the global climate, ecosystems, and pan-Arctic communities.
- Article
(1652 KB) - Full-text XML
-
Supplement
(549 KB) - BibTeX
- EndNote
- Included in Encyclopedia of Geosciences
For more than a decade, climate modelling studies have projected an “invasion” of fires to the Arctic regions (Krawchuk et al., 2009). In this paper, we review the current understanding of the changing Arctic fire regime and its impacts on fire emissions, providing a foundation for future systemic pan-Arctic fire and fire emissions analyses and coordination in the context of the Arctic Council members, Permanent Participants, observers, and working groups. This review paper is also the first to link emissions with a changing fire regime for the pan-Arctic. Previous published reviews on fires in the high northern latitudes have linked increasing fire activity in the Arctic and the boreal regions to climate-driven warming and drying (Hu et al., 2015; Walsh et al., 2020). While fires in the Arctic, defined as latitudes above 66∘ N by the Arctic Monitoring and Assessment Programme (AMAP) definition (AMAP, 1998), are not new (Wein, 1976), a consensus of evidence suggests that tundra fires are increasing (Hu et al., 2015; Masrur et al., 2018), with a potential for novel fire regimes (Young et al., 2016). Fire regimes are often defined as the main characteristics of fire activity for a given location: frequency, typical sizes of fires, annual burned area, severity, seasonality, type (surface, ground, or crown fires), and ignition cause (human or natural) (Hanes et al., 2019).
Over the past 4 decades, fire activity has increased in Alaska and the Sakha Republic of Russia but decreased slightly in the Northwest Territories of Canada, indicating large spatio-temporal variability of pan-Arctic fire dynamics (York et al., 2020). Further, in the past 3 years, there have been large fires in Fennoscandia in 2018, Alaska and Greenland in 2019, and the Russian Federation in 2020, mainly in the boreal zone, i.e. at and above 50∘ N, but with expanding fires into the Arctic region (Walsh et al., 2020), even reaching as far north as the Arctic Ocean in eastern Siberia (Kharuk et al., 2021). Thus, quantifying the impact of climate change, human ignition sources, and biophysical parameters, such as availability and/or distribution of aboveground fuels, permafrost thaw, and drying of peat, on increased fire activity in the Arctic and boreal regions is needed to understand the emerging Arctic fire regime (Krawchuk and Moritz, 2011). Here we define an emerging fire regime in the Arctic as documented increased frequency and lengthened seasonality (earlier springtime fires and fires later in autumn) of both natural and human-caused surface and ground fires (i.e. peat) increasing total fire emissions within the Arctic (see Table S1 in the Supplement for a list of all key terms).
For this review paper, the definition of open biomass burning in the Arctic will include wildland fires (sometimes referred to as and encompassing wildfires, forest fires, peat fires, and prescribed fires in natural areas) and fires in human-dominated landscapes (i.e. agricultural open burning, prescribed burning in agroforestry, timber, rangelands), with natural fires (lightning-caused ignitions) and human-caused fires differentiated where possible using reported statistics and geospatial methods. Given the strong influence of boreal systems on the Arctic in terms of fire disturbance, emissions, and shifting vegetation, we have included boreal fire regimes in this review, while specifically identifying each climatic zone as needed. Open biomass burning is a known disturbance in the Arctic Council region1 (AMAP, 2011, 2015). The 2015 Arctic Monitoring and Assessment Programme (AMAP) assessment on black carbon (BC) and ozone as Arctic climate forcers noted key characteristics of open biomass burning in the Arctic region, including human influence on both ignition and fuel management, significant interannual variation in fire events and emissions, spatial and seasonal clustering of burning related to active land management, and fuel conditions (AMAP, 2015). Since 2015, evidence of direct climate change influence on large, early season fires has increased (Wang et al., 2017) as well as fuelling extreme wildfires at the wildland–urban interface (WUI) and not just remote boreal forests and Arctic tundra (Abatzoglou and Williams, 2016; Kirchmeier-Young et al., 2019). In terms of burned area, 2015 was the largest fire year for the Alaskan tundra ecoregion (Michaelides et al., 2019).
Under future climate change, an overall increase in fires is expected in the Arctic Council region, indicating that associated emissions are also likely to increase. For instance, natural fires, defined as lightning-caused fires, may increase as lightning is predicted to increase (Púčik et al., 2017; Veraverbeke et al., 2017; Bieniek et al., 2020), under Representative Concentration Pathways (RCPs) 4.5 (stabilizing emissions) and 8.5 (high emissions) developed for the Intergovernmental Panel on Climate Change (IPCC) Fifth Assessment Report (AR5). Likewise, using the same scenarios, wildfire emissions of BC, CO, NOx, PM2.5, and SO2 could exceed anthropogenic emissions in northeastern Europe, including Sweden and Finland, by 2090 (Knorr et al., 2016). There is a clear consensus that the emerging Arctic fire regime will be marked by shifts in fire seasons; i.e. likelihood of extreme fires later in the growing season will occur in the boreal forests of eastern Canada (Boulanger et al., 2013); central and northwestern Canada (Boulanger et al., 2014); and European Russia, West Siberia, and the Far East (Sherstyukov and Sherstyukov, 2014). By the end of the 21st century under RCP6.0 (stabilizing emissions with higher CO2 equivalency than RCP4.5), the annual chance of large tundra fire in Alaska will be almost one in four, i.e. a range of 13 %–23 % predicted increases (Hu et al., 2015). Moreover, Wang et al. (2017) noted that a recent lengthening in the fire season in Canada has led to the increase in the total number of fire spread days, leading to large increases in total fire size and emissions for early season fires like the Fort McMurray megafire in Alberta. Lengthening the fire season, a component of the emerging Arctic fire regimes, means increased potential for more and larger fire emissions throughout the fire season, starting earlier in spring and lasting later into autumn.
For the past 2 decades, it has been well established that understanding fire regimes improves emission estimates from fires in high northern latitudes (Conard and Ivanova, 1997; Soja et al., 2004a) and may even be necessary for creating emission models (van der Werf et al., 2010). Further, climate change is expected to alter fire regimes and likely increase emissions (Sommers et al., 2014). For that reason, this review also includes emission estimates from adjacent boreal fires as well as temperate fire sources known to impact the Arctic region via increased atmospheric abundance and deposition of black carbon as well as greenhouse gas emissions. This review paper took shape from policy questions (Table S2) that the Expert Group on Short-Lived Climate Forcers (SLCF EG) of AMAP, a Working Group of the Arctic Council, was asked to answer for its 2021 Assessment Impacts of short-lived climate forcers on Arctic climate, air quality, and human health (AMAP, 2021). Our specific objectives are to
-
identify and review the key drivers of the Arctic fires today and in the future to characterize an emerging Arctic fire regime, with potential changes (paper Sects. 2–3 and policy question 1 in Table S2);
-
characterize fire emissions from ground- and satellite-based data sources in the Arctic, boreal, and temperate regions that impact the Arctic (paper Sect. 4 and policy questions 1 and 3–5 in Table S2);
-
contextualize emissions from the Arctic fire regime with other sectoral sources for the pan-Arctic (paper section 5 and policy questions 5–6 in Table S2);
-
identify key challenges and research questions that could improve understanding, monitoring, and management of Arctic fires in the 21st century (paper Sects. 6–8 and policy questions 2 and 6 in Table S2).
Our focus is SLCF emissions, but we note that wildfires are also a source of CO2 and other contaminants of environmental and human health concern in the Arctic, including mercury and polycyclic aromatic hydrocarbons (PAHs).
Broadly speaking, wildfires are driven by climate and weather conditions influencing flammability, fuels, and fuel conditions (Silva and Harrison, 2010; de Groot et al., 2013). Ignition from lightning strikes, fire weather (i.e. temperature, humidity, precipitation, and wind), and fuel abundance (build-up) and conditions (moisture) are the typical controlling processes for “natural” fires, i.e. fires not caused directly by human activity. Human-caused fires are driven by fuel management to reduce fire risk, land management in agricultural and timber landscapes, cultural practices, and accidents (Granström and Niklasson, 2008; Bowman et al., 2020).
Historically, both climate and humans have influenced fire activity in the pan-Arctic region. Paleofire meta-analysis of boreal biomass burning during the Holocene (4000 to 200 years ago) for the boreal zone of North America and Fennoscandia shows general trends in boreal biomass burning were primarily controlled by climatic changes, mainly mean annual precipitation in Alaska, northern Quebec, and northern Fennoscandia and summer temperatures in central Canada and central Fennoscandia (Molinari et al., 2018). Boreal needleleaf evergreen fuel composition at the landscape level across Alaska and central and southern Fennoscandia was secondary to climatic controls. These paleofire results align with recent findings by Walker et al. (2020), showing fine-scale drainage conditions, overstorey tree species composition, and fuel accumulation rates across 417 sites in boreal and taiga ecoregions of northwestern Canada and Alaska were more important than incidental fire weather in terms of fire severity and subsequent carbon emissions. Pollen-based reconstructions show prehistoric and early historic human settlements increased during wetter climates in Minusinsk Hollow in south-central Siberia, where grain and pasture yields increased 2-fold, rather than dry periods that favoured pastoralist lifestyles (Blyakharchuk et al., 2014), highlighting the connections between fire, climate, and human-dominated landscapes.
Open biomass burning from anthropogenic activities like agriculture, timber, and energy extraction are expected to increase in the Arctic as climate change expands human-dominated landscapes northward, increasing potential ignition sources (Fig. 1). The 2019 Greenland wildfire, which consumed surface vegetation and high-carbon soils for nearly a month, was caused when a campfire ignited dry ground near a public camping site of the world-renowned Arctic Circle Trail (McGwinn, 2019), indicating that tourism will need to adapt to increased fire risk in tundra landscapes. Greenland wildfires in 2017 and 2019 occurred east of Sisimiut in tundra areas with low vegetative cover and degraded permafrost but high-carbon soils during warm, dry, and sunny summers (Evangeliou et al., 2019). Timber extraction and site preparation, including operation of machinery and vehicles on ground covered in dry wood residues, currently cause large wildfires in the Arctic Council region, including the 2014 Västmanland fire in Sweden ignited by forestry vehicles during subsoiling activities (Lidskog et al., 2019), which actively burned for 18 d, creating a burn scar of over 14 000 ha (Pimentel and Arheimer, 2021). Northward agricultural expansion will likely increase human-caused open burning as wheat and maize production is expected to grow in previously permafrost areas of west Siberia (Parfenova et al., 2019). West Siberia is currently a minor source region of agricultural burning (Hall and Loboda, 2017), with many farmers insisting that fire is necessary to clear fields under present-day management and resource constraints despite bans on open agricultural burning (Theesfeld and Jelenik, 2017). This northward agricultural land could expand into the cold regions of the boreal zone (Kicklighter et al., 2014; King et al., 2018), nearing the Arctic Circle for central Siberia (Tchebakova et al., 2016). Of course, the northward agricultural transitions will also be dependent on local and/or in situ conditions limiting its expansion, such as inferior soils, existing land uses not compatible with agricultural conversion, and topographic limitations (Ioffe and Nefedova, 2004; Dronin and Kirilenko, 2011; Tchebakova et al., 2011). However, given the degraded conditions of most abandoned agricultural land in the steppes of Siberia and high interest in northern agricultural development by neighbouring Asian countries, northward development of grains and other commodity crops is expected (Prishchepov et al., 2020). Finally, suppression of wildfire in Canadian boreal communities has increased their likelihood of burning, allowing fuels to build up in and near populated places (Parisien et al., 2020), calling into question what other wildland–urban interfaces in the Arctic region may experience increased fire risk and fires due to long-term aggressive fire suppression.
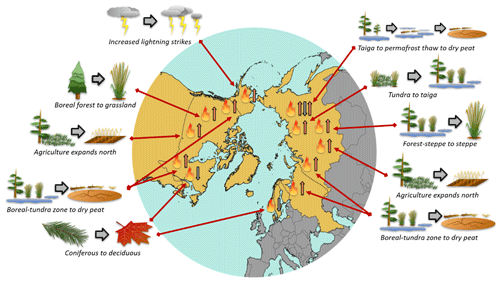
Figure 1A sample of peer-reviewed future Arctic fire risk variables due to expected ecological and meteorological transitions by mid-century and late 21st century climate change for Arctic Council member states. Upward arrows indicate increase in fire risk and downward arrows indicate a decrease in fire risk, with the location of the arrows approximate to the location of fire risk from the literature and not projections for a given country; the dashed line indicates the boundary between European Russia and Siberia and the Russian Far East. Note that taiga is used in northern forest zones completely contained in Russia while boreal is used for the rest of the pan-Arctic northern forests.
3.1 Climate change and future fires
Many future fire modelling approaches use greenhouse gas emission scenarios to project the impact of climate change on future temperature and precipitation – both influencing fuel ignition and subsequent burning (Veira et al., 2016). Increased fire risk will not be uniform across the pan-Arctic (Fig. 1). For instance, permafrost thaw will lead to a rewetting of soils (Wrona et al., 2016), reducing above-ground and below-ground fire risk. Boike et al. (2016) showed that increasing areas of thermokarst lakes were not coincident with areas of increasing fire in the central Sakha Republic. Surface fires can cause permafrost to thaw, producing thermokarst lakes (Jones et al., 2015), which previously have been considered to reduce fire risk (Sofronov et al., 2000) but are not perfect fire breaks as wildfires can “jump” (Sofronov and Volokitina, 2010). Further, changing precipitation regimes in the form of more rainfall in the Arctic for the months of March through December by the end of century using RCP8.5 projections (Bintanja and Andry, 2017) could both reduce fire risk through increased wetness and increase fire risk through more vegetation growth and/or shifting fuel regimes. End-of-century modelled fire–climate interactions under RCP6.0 for Alaska showed summer temperatures and annual precipitation are the most important climatic factors driving the likelihood of new wildland fire regimes in tundra and the boreal forest–tundra boundary (Young et al., 2016). Burned area is predicted to increase 40 % to 50 % in the high latitudes under climate-forcing scenario RCP8.5 given modelled changes in fuel loads, fuel moisture, and increased lightning frequency (Krause et al., 2014). Increased convective cloud formation has been documented in the Russian Arctic (Chernokulsky and Esau, 2019) and the North American boreal forest (Veraverbeke et al., 2017), with a 5 % increase in convective storms in northern Europe projected by the end of the 21st century under RCPs 4.5 and 8.5 (Púčik et al., 2017). In general, lightning frequency is expected to increase over areas north of 50∘ N. The strongest projected relative increase is approximately 100 % across northern Europe under the RCP8.5 scenario by the end of the century (Groenemeijer et al., 2016). Moreover, since summers are expected to become drier in the future (Venäläinen et al., 2020), the role of lightning as an ignition source for wildfires may increase for northern Europe.
Figure 1 depicts transition themes and associated fire risks taken from the scientific literature, with general locations on the map derived from the locations of these studies. These ecological and meteorological studies rely on gridded climate scenarios from future greenhouse gas emission scenarios in order to predict fire risk for mid-century (2050) and late century (2100). First, as boreal forests experience permafrost thaw, where initially wet soils (Wrona et al., 2016; O'Neill et al., 2020) are followed by increasingly dry ground fuels (Turetsky et al., 2015; Box et al., 2019). Topography plays a crucial role in determining shifting habitats, where drying will dominate on tilted surfaces and bogging will dominate on flat terrain (Tchebakova et al., 2009). As the Siberian Arctic tundra is dominated by relatively flat terrain, bogging is predicted to prevail. Second, anticipated transitions of boreal forest to deciduous forest stands would decrease fire risk in eastern Canada and small regions of interior Alaska (Terrier et al., 2013; Foster et al., 2019; Mekonnen et al., 2019), as deciduous species are less flammable than coniferous species (Päätalo, 1998; Krawchuk et al., 2006). Third, expansion of grassland ecosystems is predicated in northwestern Canada and Alaska (Wang et al., 2019; Whitman et al., 2019) and Siberia (Tchebakova et al., 2009, 2016). Fourth, increased lightning strikes will increase fire risk in Alaska (Veraverbeke et al. 2017) but also northern Europe (Púčik et al., 2017). Fifth, the interaction between climate-driven changes in fire regimes and permafrost will compel a decrease in and a northern migration of Siberian taiga, which will result in the transition of tundra to taiga in northern Siberia (Tchebakova et al., 2009, 2011; Sizov et al., 2021). Permafrost is not predicted to thaw deep enough to sustain dark-needled taiga (Pinus sibirica, Abies sibirica, and Picea obovata); nonetheless light-needled coniferous Larix is predicted to continue to dominate in eastern Siberia, maintaining a higher fire risk according to the Russian fire hazard rankings (Melekhov, 1980). The Russian fire hazard ranking systems show a decrease in fire risk from light needle conifers (Scots pine, larch) to deciduous broad-leaf tree species (birch, aspen, willow) that exist between the temperate and boreal zones, as well as along river valleys. Fire risk is also lower in dark-leaf conifers (Melekhov, 1980). Fire return intervals (FRIs) are consistent with Melekhov (1980), with a mean FRI of 36 years (range 17–133) in light coniferous forest compared with a mean FRI of 196 years (range 75–725) in dark-coniferous forest (Furyaev, 1996; Shvidenko and Nilsson, 2000; Soja et al., 2006). Larix are a fire-tolerant species, and dark-leaf coniferous species are a shade-tolerant secondary-succession cohort (Shugart et al., 1992). Sixth, forest–steppe and steppe are predicted to dominate over half of Siberia, largely forced by climate and increases in fire regimes (Tchebakova et al., 2009). The forest–steppe that exists at the southernmost extent of the Siberian boreal forest is transitioning to steppe due to increases in extreme fires that burn the soil organic matter to mineral soil and repeated fires and high temperatures that kill regenerating seedlings. Seventh, northward agricultural expansion may increase human-caused agricultural burning as wheat and maize (silage) establish in previously permafrost areas of east Siberia (Tchebakova et al., 2009; Parfenova et al., 2019), expanding into the cold regions of the boreal zone (King et al., 2018) in North America as well. Finally, a 3-fold increase in permafrost thaw in the boreal zone under RCP4.5 by 2100 is likely to increase the amount of peat fuels available for burning (Nitzbon et al., 2020).
Previous work has identified the Arctic as a regional “hot spot” for interannual variability of key atmospheric constituents, with wildfire being the major driver of this variability (Fisher et al., 2010; Monks et al., 2012; Voulgarakis et al., 2015). As stated earlier, climate warming can cause more ignitions from lightning (Veraverbeke et al., 2017) and degraded permafrost due to increasing dry ground fuels, including peat (Turetsky et al., 2015), and increased fire severity (Teufel and Sushama, 2019). Using the RCP8.5 scenario, Teufel and Sushama (2019) estimate that a 2.0 ∘C global threshold in temperature increase, which could be reached around 2031, may cause 42 % of pan-Arctic permafrost to abruptly degrade and increase fire severity in Russia, Canada, and Alaska. By the end of the century, wildland fire risk is expected to increase, with length of fire seasons – measured in terms of daily severe fire weather occurrence – predicted to expand by as much as 20 d for high northern latitudes using the A1B (roughly corresponding to RCP6.0), A2 (∼ RCP8.5), and B1 (∼ RCP4.5) scenarios (Flannigan et al., 2013). Similarly, Sherstyukov and Sherstyukov (2014) predict an increase of >50 d of high-fire-risk days by 2100 for Russia under the RCP8.5 scenario, with a potential to double annual forest-fire-burned area. Using CMIP5 model intercomparisons, Lehtonen et al. (2016) found that large (≥0.1 km) boreal forest fires in Finland may double or even triple by the end of century, using RCP4.5 and RCP8.5 scenarios, but with large inter-model variability. Robust predictions of future burned area in wildland and human-dominated landscapes for the Arctic require an understanding and quantitative simulation of the major drivers of fire (specifically climate and fire weather, ignition, fuels, and humans), including coupled dynamics between and among these drivers (Riley et al., 2019).
3.2 Biogeography of future fires
The climate-induced vegetation shifts, which would also modify fire risk and related emissions, present a complex matrix for the Arctic Council member states. Predictions of boreal forest transition to deciduous forest stands would decrease fire risk in eastern Canada and interior Alaska (Terrier et al., 2013; Foster et al., 2019; Mekonnen et al., 2019). Wang et al. (2019) found that these trends are already occurring in Alaska and northwestern Canada using 3 decades of Landsat imagery with a 30 m resolution, as climate drives grass and shrub expansion in the Arctic, and wildfires drive most of the evergreen forest reduction and expansion of deciduous forests in the boreal regions. Further work in mature deciduous forests of Interior Alaska show that current canopy “gaps” are related to ecological shifts to evergreen shrubs and lichens, grasses, and mosses, thus increasing overall fire risk due to presence of these high-flammability coniferous species in these small areas within low-flammability deciduous stands (Alexander and Mack, 2017). Further, with satellite mapping of taiga–tundra vegetation of moderate to high spatial and temporal resolution shows a northern expansion of trees, but with complex patterns of diffuse and abrupt transitions from forests to non-forests (Montesano et al., 2020).
There is a consensus that prolonged fire seasons will become more common, increasing in the eastern boreal forests of Canada (Boulanger et al., 2013), central and northwestern Canada (Boulanger et al., 2014), and European Russia (particularly the Republic of Karelia and Leningradskaya oblast), west Siberia, and the Far East (Tchebakova et al., 2009; Sherstyukov and Sherstyukov, 2014). Wang et al. (2017) note that recently the fire season in Canada is characterized by a higher total number of fire spread days, leading to large increases in total fire size and emissions for early season fires like the Fort McMurray megafire in Alberta, which burned both forests and peatlands and was caused by humans (Hanes et al., 2019). Lengthening the fire season means increased potential for more and larger fire emissions throughout the fire season, starting earlier in spring and lasting later into autumn. Ignition likelihood is often modelled by considering the moisture conditions of ground fuels (i.e. litter) and the organic layer (i.e. forest canopy), whereby humans are the most likely source of fire on the ground, and lightning is the most likely the source for canopy fires (Wotton et al., 2003). Veraverbeke et al. (2017) introduced a positive feedback loop between climate, lightning, fires, and northward forest expansion, whereby surface energy fluxes from forests appeared to be increasing the probability of lightning in Alaska.
Boreal fire regimes and related changes in spring albedo (relative reflectance) and the radiation balance are distinct in North American (crown-fire-dominated) and northern Eurasian (surface-fire dominated, smaller negative shortwave forcing) systems (Rogers et al., 2015). In the near future, these changes may be positive but become negative in the midterm and long term. In general, climate change accelerates forest growth at high northern latitudes due to a longer growing season. Elevated CO2 concentration decreases transpiration and increases photosynthetic rate and thus enhances forest growth (Peltola et al., 2002; Kellomäki et al., 2018). However, abiotic and biotic damages in particular may have negative effects on forest growth and dynamics (Seidl et al., 2014). For example, drought increases the risk of forest fires but also negatively impacts the growth of Norway spruce (Picea abies) and exposes trees to biotic damages. Snow damages are estimated to increase in northeastern Europe but decrease elsewhere in Europe by the end of century under the RCP4.5 and 8.5 scenarios (Groenemeijer et al., 2016). Wind damage risk is expected to increase due to the shortening of the soil frost period (Venäläinen et al., 2020), as frozen soils anchor trees in the ground, thus making them less vulnerable to uprooting. Many forest insects responsible for bug kill of trees will benefit from climate change due to established linkage of increased habitat range and increased winter temperatures (Pureswaran et al., 2018). Climate-driven bug kill increases the amount of easily burnable material in forests and can influence fire risk. For example, a large-scale bark beetle invasion could increase the amount of fuel via dead wood, increasing ignition risk and crown fire risk as well as increasing the need, danger, and cost of fuels and fire management of insect-attacked forests (Jenkins et al., 2014). According to Venäläinen et al. (2020), a warming climate is likely to increase the risk of bark beetle outbreaks and wood decay caused by Heterobasidion spp. root rot in Finland's coniferous forests. Siberian forests have already experienced a northern progression of the destructive Siberian moth (Dendrolimus sibiricus Tschetvericov) by a distance of ∼0.5∘ and a decrease in its regeneration cycle from 2 to 1 year, prompted by drought and increasing temperatures (Baranchikov and Montgomery, 2014; Kharuk et al., 2017). Moreover, the probability of forest-damaging cascading and compounding events, i.e. large-scale wind damage followed by a widespread bark beetle outbreak, may increase remarkably in the future for the high northern latitudes. Future climate conditions are expected to become more favourable for forest fires in the boreal zone, even in highly managed regions.
Under RCP8.5, Stralberg et al. (2018) estimated that by 2100, grasslands will replace much of the upland conifer, mixed forests, and deciduous forests for a large area of the boreal forest zone of northern Alberta. Shorter fire return intervals combined with climate-change-induced drought will reduce the resiliency of evergreen and broadleaf species to re-seed and/or establish after wildfires, leading to expansion of grassland ecosystems in what is now northern Canadian forests (Whitman et al., 2019). Increased grass-dominated landscapes would create a new fire regime of frequent but low-severity fires, with the likelihood of SLCF transport to the Arctic most likely in the spring months of March through May (Hall and Loboda, 2018). Grassland fires produce less energy, with smoke plumes more similar to crop residue burning, and are unlikely to breach the tropopause for consistent, year-round transport of smoke to the Arctic (Hall and Loboda, 2017), unlike the current observed deposition from boreal forest fires in the Arctic (Thomas et al., 2017). Further, Smirnov et al. (2015) found forest fires in European Russia during 2008–2012 occurred mainly in June and August, with Siberia and the Russian Far East being the main sources of BC emissions during a time when transport to the Arctic is unfavourable. In the Sakha Republic, Kirillina et al. (2020) found that from 2011 onwards, fire seasons have been 13 d longer than previously, on average, and starting from 2009 onwards, fire seasons have started earlier in April, sooner than previous years. A peak fire occurrence across a 3-month period of May to July persists in Sakha. During the 2020 extreme fire season in Siberia, high-resolution satellite data from the European Space Agencies' Sentinel-2 detected fires around still-frozen thermokarst lakes above 70∘ N (McCarty et al., 2020). This indicates that more BC from future early season burning in and near Arctic Siberia could be available for transport and thus deposition on snow and ice that accelerates melting, as well as associated climate feedback due to effect on albedo. Given this, current and future early season fires are particularly relevant because Arctic snow and sea ice coverage are much more widespread in the early burning season than late season – meaning earlier BC deposition could accelerate springtime melt to April, before the usual start of the melt season in May (Stroeve et al., 2014). Emission factors for biomass burning in grassland and steppe ecosystems are generally smaller than those of boreal forests (Akagi et al., 2011; Andreae, 2019), which potentially implies different impacts on atmospheric chemistry and SLCFs. Therefore, while boreal forest fires emit more SLCFs than grasslands and cropland fires, the springtime burning of northern grasslands, peatlands, and croplands – often human-caused – means these emissions are more likely to be transported to the Arctic during favourable transport conditions in March, April, and May than summertime forest fires.
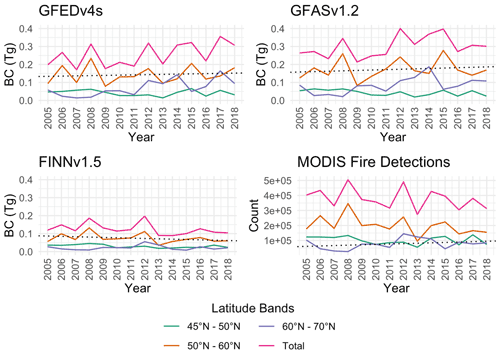
Figure 2Annual black carbon (BC) emissions in teragrams from three commonly used global fire emissions models and annual fire activity from the MODIS Collection 6 active fire product (Terra and Aqua) split by latitude ranges for the Arctic Council region, 2005–2018; note the y axis has been standardized for each model for ease of comparison; the dotted line is the positive trend for BC emissions from open biomass burning and 1 km MODIS active fire detections (Terra and Aqua) for 60 to 70∘ N.
In Sects. 4 and 5, we present new emissions work that builds on the 2015 AMAP assessment of BC and ozone (AMAP, 2015), which included 2005 biomass burning emissions from an the Global Fire Assimilation System (GFASv1.2; Kaiser et al., 2012), Global Fire Emissions Database version 2 (GFEDv2; van der Werf et al., 2006), GFEDv3 (van der Werf et al., 2010), the Global Inventory for Chemistry-Climate studies (GICC; Mieville et al., 2010), MACCity (Lamarque et al., 2010), and the Fire Inventory from NCAR (FINNv1.5; Wiedinmyer et al., 2011) for above 60∘ N. For the 2021 AMAP assessment, we focused on longitudinal biomass burning emission models for the years 2005 through 2018 using the Global Fire Emissions Database with small fires (GFEDv4s; van der Werf et al., 2017), FINNv1.5 (Wiedinmyer et al., 2011), GFASv1.2 (Kaiser et al., 2012), the Quick Fire Emissions Dataset (QFEDv2.5r1; Koster et al., 2015), and the Fire Energetics and Emissions Research (FEER; Ichoku and Ellison, 2014). These versions of GFAS, GFED, FINN, FEER, and QFED analysed rely on Moderate Resolution Imaging Spectroradiometer (MODIS) thermal anomalies, with GFEDv4s integrating the MCD64A1 burned area product with the MODIS active fire product to account for small fires (Giglio et al., 2009). For each global fire emissions model, the area of interest was defined roughly as 45 to 80∘ N (N) globally, split by latitude ranges of 45 to 50∘ N: temperate, 50 to 60∘ N: boreal, 60 to 70∘ N: low Arctic, and 70 to 80∘ N: high Arctic. Average annual emissions from open biomass burning from all sources (agriculture, boreal forest, tundra, peat, etc.) were calculated for 2005–2018 for BC, methane (CH4), carbon monoxide (CO), and fine particulate matter (PM2.5).
Since the Visible Infrared Imaging Radiometer Suite (VIIRS) provides daily, global observations of low-intensity fires (Johnston et al., 2018), a custom AMAP open biomass burning emissions inventory was developed for the year 2018 to utilize VIIRS's capabilities to detect smouldering fires which are common in peat landscapes. Suomi-NPP VIIRS active fire from day and night detections (Oliva and Schroeder, 2015) were assumed to completely burn each 375 m2 pixel. A “best-guess” land cover was created from three different land cover products, with a sample (n=30 locations) validation of land cover type performed for each country. Ultimately, the 750 m VIIRS Surface Type land cover product (Zhang et al., 2018) was used for North America, Greenland, and the Russian Federation, augmented by the revised 1 km Circumpolar Arctic Vegetation Map (Raster CAVM; Raynolds et al., 2019) for missing values in the high northern latitudes. For Norway, Sweden, and Finland, the 10 m Land Cover Map of Europe 2017 from the Sentinel-2 Global Land Cover Project (Gromny et al., 2019) was used. All land cover maps were reclassified into the International Geosphere-Biosphere Program (IGBP) classes for ease of emission calculations. Fuel loadings and combustion completeness were taken from Van Leeuwen et al. (2014), with tundra values used for Greenland. Emission factors were taken from Akagi et al. (2011), with updates from Andreae (2019).
Most fire activity and emissions occur between 50 and 60∘ N, with very few open biomass burning emissions between 70 and 80∘ N and zero satellite observations of fire above 80∘ N (Fig. 2). The latitude band of 50 to 60∘ N corresponds to the southern extents of the boreal region, an area experiencing increasing fires due to climate change (de Groot et al., 2013), and includes the largest wildfires in British Columbia's history, burning 1200 km2 in summer 2017 (Kirchmeier-Young et al., 2019). Note, however, that fire activity detected by the 1 km MODIS MCD14 Collection 6 active fire data (Giglio et al., 2016), with confidence values >50 %, has a positive trend for fires occurring between 60 and 70∘ N but not for the latitude bands of 45 and 50∘ N or 50 and 60∘ N (Fig. 2).
In the 14-year emissions estimates from GFAS, GFED, and FINN, a clear shift has occurred in the zonal distribution of fire since the mid-2000s. Fire emissions are increasing more north of 60∘ N compared to the temperate zone of 45 to 50∘ N, where large amounts of human-caused burning and wildfires throughout North America, Europe, and Eurasia occur (Fig. 2). This trend is pronounced in GFED and GFAS, with these two models showing a positive trend (note the dotted line in Fig. 2) and FINN showing a slight decrease in later years even as total MODIS active fire detections increased (bottom panels of Fig. 2). The 2005 to 2018 multi-model annual average BC emissions from all open biomass burning sources in the Arctic (60 to 80∘ N) and adjacent regions known to impact smoke transport into the Arctic (45 to 60∘ N) are 0.34 Tg. The years with the highest multi-model average are 2012, 2008, and 2015 with BC emissions of 0.45, 0.44, and 0.41 Tg, respectively. The lowest annual average BC emission years from the five global fire emissions models are 2007 and 2013, both with 0.27 Tg. The fire emissions model with the consistently highest BC emissions is QFED, with an annual average of 0.68 Tg (Fig. 3). FEER, GFAS, and GFED have more agreement, with annual BC emission averages of 0.32 (± 0.07) Tg, 0.30 (± 0.07) Tg, and 0.25 (± 0.06) Tg, respectively. FINN has the lowest annual average BC emissions of 0.130 Tg, with higher emissions in 2012 (0.20 Tg) and 2008 (0.19 Tg). The AMAP model designed specifically for the pan-Arctic, which was based on VIIRS active fire data and region-specific land cover types, produced slightly higher emission estimates than FINN (Fig. 3) for the year 2018. The AMAP model predicts BC emissions of 0.13 Tg and CH4 emissions of 1.39 Tg, compared to FINN's 0.11 Tg of BC and 1.19 Tg of CH4. Compared for 2018 only, GFED has marginally higher BC emissions than GFAS, while methane emission estimates from GFAS are substantially higher than those of GFED.
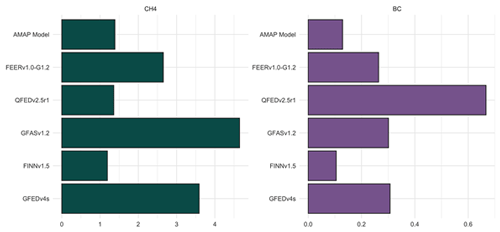
Figure 3Annual 2018 BC and CH4 emissions in teragrams from five global fire emissions models and a custom AMAP fire emissions model for north of 45∘ N.
Table 1Summary table of BC, PM2.5, and CH4 emissions in teragrams (Tg) from reported statistics on burned area from the Arctic Council members; sources for burned area include Norway (DSB, 2020), Greenland (Markuse, 2019), Finland (Ketola, 2020), Sweden (Betänkande av 2018 års skogsbrandsutredning, 2019), Canada (CIFFC, 2020), Alaska (Alaska Division of Forestry, 2020), the contiguous United States (NIFC, 2019), and the Russian Federation (Aviales, 2019). Fuel loadings and combustion completeness are from Van Leeuwen et al. (2014) for boreal forests, with tundra values used for Greenland and temperate forests for the USA/CONUS; emission factors are taken from GFED4.
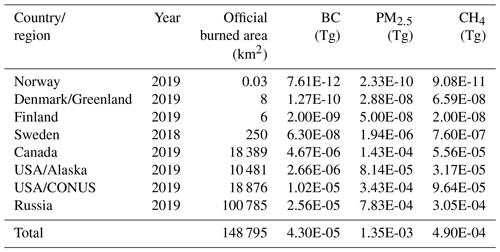
Ground-based official statistics vary greatly by country or sub-region (i.e. Alaska and Greenland) for circa 2019 (Table 1). Table S3 provides the emission variables used to calculate emissions for each country or sub-region of the pan-Arctic, reporting official burned area statistics. The Russian Federation has the highest burned area, with over 100 000 km2 burned. In 2019, open biomass burning in European Russia – comprising the Northwestern, Central, Southern, North Caucasus, and Volga federal districts – accounted for only 190 km2 of burned area (Aviales, 2019). Approximately 98.2 % of burned area in Russia occurred in the Urals, Siberia, and Far East federal districts. In general, Greenland, Fennoscandia, and European Russia are the regions with the lowest burned area and open biomass burning emissions, with all regions experiencing the most burning in 50 to 60∘ N and the second most burning in the latitudinal band of 60 to 70∘ N. Alaska and Canada account for approximately 29 000 km2 of total pan-Arctic biomass burning and 17 % of the BC emissions, while the contiguous United States (CONUS) accounted for 24 % of BC emissions. It should be noted that while Canada and the CONUS reported similar official statistics for burned area, fires in temperate zones of the CONUS tend to emit double the emissions of boreal ecosystems (Table 1) due to higher fuel loadings, emission factors, and combustion completeness (Table S3). Greenland is a novel fire regime in the Arctic, with two relatively substantial wildfires in 2017 (Evangeliou et al., 2019) and 2019 (Table 1) that accounted for more burned area and emissions than Norway or Finland. In 2019, the majority of open biomass burning and related emissions for the Arctic Council member states originated in Siberia and the Russian Far East, followed by the CONUS, Canada, and Alaska.
Focusing on a potentially novel Arctic fire regime in Greenland allows us to localize the impact of fires on BC deposition and ice and what that may hold for the future. Unusual fires were observed in western Greenland by pilots and also confirmed by satellites between 31 July and 21 August 2017, after a period of warm, dry, and sunny weather. The largest wildfire grew to approximately 22 km2 in size and was eventually extinguished by rain (Cartier, 2017). The fires burned >20 km2 of high-carbon soils – potentially peat due to smouldering and fire spread behaviour – that became vulnerable due to permafrost degradation (Daanen et al., 2011). Work by Evangeliou et al. (2019) estimated the 2017 wildfire consumed a fuel amount of about 0.12 Tg of carbon (C) and emitted about 0.00002 Tg (20 Mg) of BC and 0.0007 Tg (700 Mg) of organic carbon (OC), including 0.00014 Tg (140 Mg) of brown carbon (BrC – the portion of OC that absorbs towards shorter wavelengths). Although these fires were small compared to fires burning at the same time in North America and Eurasia, a large fraction of the BC, OC, and BrC emissions (30 %) were deposited on the Greenland ice sheet. Measurements of aerosol optical depth in western Greenland showed that the air was strongly influenced by the Canadian forest fires. Even so, the Greenland fires had an observable impact, doubling the column concentrations of BC. The spatiotemporal evolution and, in particular, the top height of the plume were also confirmed using the vertical cross section of total attenuated backscatter (at 532 nm) from Cloud-Aerosol Lidar and Infrared Pathfinder Satellite Observations (CALIOP) lidar. The maximum albedo change due to BC and BrC deposition from the Greenland fires was −0.007 at maximum, while the average instantaneous BOA (bottom of the atmosphere) radiative forcing over Greenland at noon on 31 August 2017 (post-fire) was between 0.03 and 0.04 W m−2, with locally occurring maxima up to 0.77 W m−2. Here, the BOA included the aerosol effects of both BC and BrC in the atmosphere and deposited on the snow. The albedo effect (a decrease) was very low (0.007), practically unmeasurable. The summer 2017 fires in Greenland had a small impact on the Greenland ice sheet, causing almost negligible extra radiative forcing. This was due to the comparably small size of the fires in Greenland, in a global and pan-Arctic context. However, with 30 % of the emissions deposited on the Greenland ice sheet, the 2017 Greenland wildfires were very efficient climate forcers on a per unit emission basis and adding to current BC deposition from North American boreal forest fires (Thomas et al., 2017). Thus, while the fires in 2017 were small in size on a global scale, if the expected future warming of the Arctic (IPCC, 2013) produces more and larger fires in Greenland (Keegan et al., 2014), this could indeed cause substantial albedo changes and, in turn, contribute to accelerated melting of the Greenland ice sheet.
To place current Arctic fire emissions into context, GFASv1.2 emissions (Kaiser et al., 2012) were compared to total anthropogenic emissions of BC, PM2.5, and CH4 estimated with the integrated assessment model GAINS (Greenhouse gas – Air pollution Interactions and Synergies) (Amann et al., 2011; Klimont et al., 2017). The GAINS model explicitly considers environmental policies and assesses their impact on current and future emissions (Amann et al., 2011; Klimont et al., 2017; Amann et al., 2020) and projects emissions from various anthropogenic sectors until 2050; here we compare emissions estimated for 2010, 2015, and 2020. Global GFAS data were downloaded from the European Centre for Medium-Range Weather Forecasts (ECMWF, https://apps.ecmwf.int/datasets/data/cams-gfas/, last access: 13 September 2021, login required). GFAS was chosen for this comparison because it was produced in near-real time on the global scale, unlike GFED, which is a historical product and at the time of this writing had not completed the 2020 emission estimates. GFAS also did not show consistently low emissions for the pan-Arctic region, like FINN (Fig. 2). Further, GFAS is currently used as an operational product for global and regional forecasting (Inness et al., 2019) and thus likely to be integrated into policy-making decisions on fire management. The GFAS wildfire and biomass burning emissions include all open biomass burning activity, with no differentiation between human-caused ignitions and natural sources like lightning, but attempt to remove spurious fire emissions from industrial, volcanic, and geothermal sources (Rémy et al., 2017). Data were clipped to pan-Arctic extents at 50, 60, and 65∘ N. The GFAS emissions data, referred to as wildfire emissions in this review due to inability to differentiate fire types in the emissions data, have a spatial resolution of 0.1∘, so they were aggregated to 0.5∘ for comparison with GAINS. Since the 2020 wildland fire season in the Arctic was unprecedented (Witze, 2020), with approximately 27 % of fires in Siberia burning above 65∘ N (Conard and Ponomarev, 2020), the 2020 GFAS emissions can be used to represent what potential future fire regimes by mid-century, i.e. 2050, may be like, with climate-change-driven expansion of fire seasons and likelihood for extreme fire weather and risk (see Sect. 3).
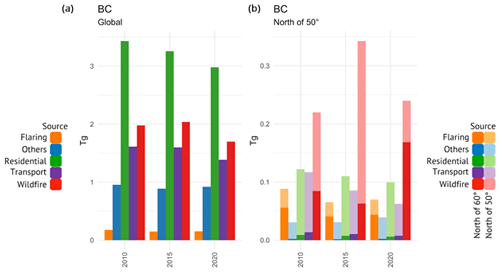
Figure 4Annual black carbon emissions for 2010, 2015, and 2020 from four anthropogenic source sectors (residential, transport, flaring, others) from GAINS and wildfires from GFAS, presented globally (a) and (b) at 50 to 60∘ N (lighter colours of the cumulative bar) and north of 60∘ N latitude (darker colours of the cumulative bar).
Figures 4, 5, and 6 present 2010, 2015, and 2020 annual BC, PM2.5, and CH4 emissions, respectively, from four main source sectors of GAINS ECLIPSEv6b (https://iiasa.ac.at/web/home/research/researchPrograms/air/Global_emissions.html, last access: 13 September 2021; Höglund-Isaksson et al., 2020) and biomass burning from GFAS at the global scale (left) and above 50 and 60∘ N. Globally, residential combustion, i.e. oil, coal, wood, used for heating, is the main anthropogenic source of BC emissions for these years and is the largest overall when compared with GFAS wildfire emissions (Fig. 4a). Bond et al. (2004) estimated BC emissions from open biomass burning from wildlands and agricultural fires to be higher than other sources, but we did not find that when comparing GAINS emissions with GFAS fire emissions at the global scale. However, in the northern latitudes, wildfires surpass the four anthropogenic sources: residential, transportation, gas flaring during oil and gas exploration and production, and the sum of all other sources, i.e. “Others”. North of 60∘ N, gas flaring is the main anthropogenic source, with comparable but still smaller emissions than GFAS wildfire emissions estimates. As Fig. 4 shows, 2020 was an extreme year for Arctic wildfires (York et al., 2020), with BC emissions above 60∘ N twice as high as in 2010 and 2015. For PM2.5, wildfires have higher emissions than the anthropogenic sectors globally, and the difference increases in the northern latitudes (Fig. 5). Globally, the agriculture sector is the main source of CH4, with fossil fuel production, distribution, and use (including flaring) and waste sectors all emitting more than wildfires (Fig. 6). Above 50∘ N, the same anthropogenic sectors are the main CH4 sources, though in 2020 wildfires emitted more methane than the others sector. A similar phenomenon occurred above 60∘ N, where across all years, wildfire emissions are higher than the other anthropogenic sectors except for the energy sector.
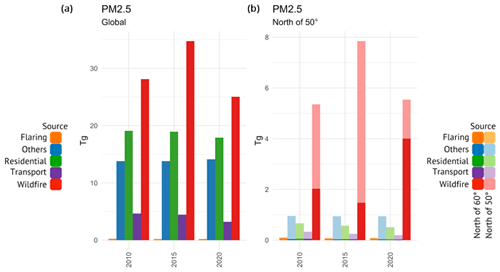
Figure 5Annual PM2.5 emissions for 2010, 2015, and 2020 from four anthropogenic source sectors (residential, transport, flaring, others) from GAINS and wildfires from GFAS, presented globally (a) and (b) at 50 to 60∘ N (lighter colours of the cumulative bar) and north of 60∘ N latitude (darker colours of the cumulative bar).
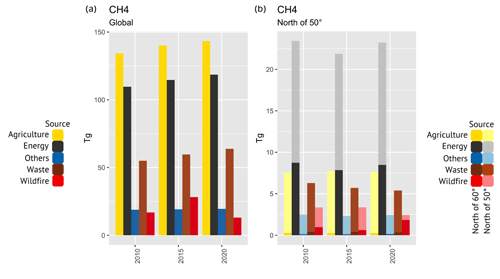
Figure 6Annual CH4 emissions for 2010, 2015, and 2020 from anthropogenic source sectors (agriculture and energy including flaring, waste, and others) from GAINS and wildfires from GFAS, presented globally (a) and (b) at 50 to 60∘ N (lighter colours of the cumulative bar) and north of 60∘ N latitude (darker colours of the cumulative bar).
Arctic shipping is often brought up as a potentially important source of BC within the Arctic in the future. According to GAINS, in 2015 shipping comprised only 0.6 % of anthropogenic BC emissions north of 60∘ N. However, according to a white paper by the International Council on Clean Transportation (ICCT; Comer et al., 2020), BC emissions from Arctic shipping increased by 85 % between 2015 and 2019. Their definition of Arctic is as described in the International Maritime Organization (IMO) Polar Code; i.e. they assessed shipping in much of the high Arctic above the Barents and Kara seas but inclusive of waters between Alaska and Russia as far south as 60∘ N. In our comparison, shipping is included in the transport sector of GAINS emissions.
Figure 7 shows the monthly BC emissions averaged from 2010, 2015, and 2020 for the globe and the three northern latitude breaks of 50, 60, and 65∘ N for the two leading sectors – wildfires and residential combustion. As with global annual emissions (Fig. 4), residential combustion is the main source sector in most months. However, in July and September the wildfire emissions are similar to residential combustion, and in August they are higher at the global scale. These two sectors show opposite temporal profiles during the year (Fig. S1 in the Supplement). Residential combustion is highest in the winter months, but wildfires grow during the spring and reach their maximum in the summer, generally falling off in September with the exception of 2020 fire emissions. In the northern latitudes, strong spring emissions in April correspond with the global signal (upper panels of Fig. 7), while the summer months comprise an even larger share of the annual emissions than in the global average.
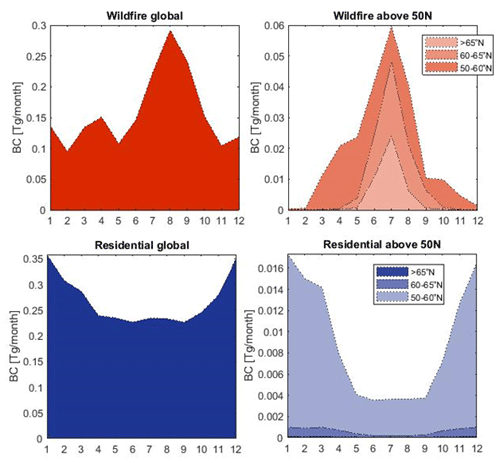
Figure 7Monthly black carbon emissions from the leading anthropogenic sector, residential heating, in GAINS and wildfires from GFAS based on global estimates (left) and by latitudinal ranges (right); emissions are averaged from the given years of 2010, 2015 and 2020 to align with the GAINS data availability.
Consistently, wildfire emissions account for more than half of all black carbon emissions north of 60 and 65∘ N (Fig. 8), representing up to 74 % and 82 % of 2020 BC emissions, respectively (Table S4). At these northern latitudes, wildfires and flaring are the main sources of black carbon, especially north of 65∘ N, with these two sectors accounting for 93 % of black carbon emissions, compared to 88 % for 60∘ N. North of 50∘ N, residential, transport, and flaring are proportionally larger than north of 60∘ N and 65∘ N, but still less than wildfire emissions (Fig. S2). North of 60∘ N, wildfire emissions have increased from 2010 to 2020, particularly above 65∘ N. Of those wildfire emissions from GFAS that were above 60∘ N, 21 % in 2010 and 27 % in 2015 occurred above 65∘ N (Table S4). However, in 2020 the percentage was 56 % (Fig. 8), indicating how extreme the 2020 wildfire year was in the Arctic.
Given the large portion of black carbon emissions from fires in comparison to anthropogenic sources as modelled by GAINS, understanding the local climate and air pollution impacts for the Arctic Council region is key. For example, the timing of fires in agricultural landscapes, boreal forest fires, and the Arctic tundra is during the early spring to early summer months (i.e. March through May for 50∘ N and May and June for 60 and 65∘ N as seen in Fig. S1) when BC transport and deposition to the Arctic is possible and critical for the cryosphere (Hall and Loboda, 2018) and air pollution (Law and Stohl, 2007), from both long-range (Thomas et al., 2017) and local sources of BC deposition (Evangeliou et al., 2019). For example, BC transport is possible as early as March into mid-May for agricultural landscapes of eastern Europe (Hall and Loboda, 2017) and peatlands, grasslands, and forests in North America (Qi and Wang, 2019) and fires in grasslands, forests, and agricultural lands most common in southern Siberia (Kukavskaya et al., 2016) and the Russian Far East (Hayasaka et al., 2020) during the spring months of March, April, and May. The boreal forest fire season starts in April and May in Canada (Tymstra et al., 2020) and Siberia (Soja et al., 2004b; Conard and Ponomarev, 2020), moving north into Alaska by early June (Partain et al., 2015). Fires and associated transport of black carbon to the Arctic in the spring months of March to June tend to be climatically important when deposition on the cryosphere can accelerate surface melting (Bond et al., 2013). In spring and summer of 2020, fires in the Arctic landscape of the northern Sakha Republic were burning as early as the beginning of May (McCarty et al., 2020), indicating a local source of black carbon. Likewise, wildfires in Greenland in July 2017 and July 2019 confirm that a local source of BC deposition on the Greenland Ice Sheet is possible (Evangeliou et al., 2019). Wildfire PM2.5 emissions are local sources of air pollution for urban and rural communities across the Arctic (Mölders and Kramm, 2018; Schmale et al., 2018), often peaking in summer months.
Fuel management, like prescribed fires and even allowing wildfires to burn under non-severe fire weather conditions, may be more effective than fire suppression and/or efforts to eliminate all fire from northern landscapes (McWethy et al., 2019), including in novel landscapes caused by warming in the Arctic. Fuel treatments in the boreal zones of Alaska were modelled to be effective for at least 14 years post-treatment, especially in shaded fuel breaks that reduce canopy cover and ladder fuels (Little et al., 2018). However, in dried and degraded peatlands of the Arctic region, fuel management will be more complicated outside the boreal forest and forest–tundra gradient, where mulching treatments that convert canopy and surface fuels to a masticated fuel bed can limit peat burn depth in black spruce (Picea mariana) stands (Wilkinson et al., 2018). Privately owned grassy tussock tundra and dwarf shrub tundra vegetation types are more likely to burn than low shrub tundra in Alaska (Hu et al., 2015), with relatively rapid vegetation re-greening within a decade after burning for shrub and tussock tundra (Rocha et al., 2012) – potentially re-establishing the shrub and tussock tundra fuelbed for repeat burns. While prescribed burning could be effective in fuel management for tussock and dwarf shrub landscapes of the tundra, prescribed burning effectiveness for peatlands is less clear. Peat fire risk and burn depth, however, are less influenced by canopy and ground vegetation and more by soil bulk density (impacting air availability in soils), the water table depth, and precipitation (Kieft et al., 2016). After the devastating 2010 fires in the Moscow region, the regional government undertook an ambitious 70 000 ha peatland rewetting project to reduce fire risk (Sirin et al., 2014), a landscape-scale process that can be monitored using existing Earth observation sensors at moderate resolution (30 m Landsat to 10 m Sentinel-2; Sirin et al., 2018). To date, the effectiveness of this campaign is unclear, but in theory it should reduce fire risk. In the larger context of CH4, Günther et al. (2020) used a radiative forcing model to determine that methane emissions from peatland rewetting are less significant in the short term when compared to the CO2 emissions from degraded or drained peatlands that increase long-term warming when rewetting is postponed. Adaptive management strategies of the timber industry in Fennoscandia could also reduce fire risk. Intensive management via ditch network maintenance and fertilization of drained peatlands will increase timber values while also rewetting the peat (Ahtikoski and Hökkä, 2019). Prescribed burning for silvicultural retention and maintaining and regenerating pure stands can also reduce fuel loadings while increasing biodiversity (Lindberg et al., 2020).
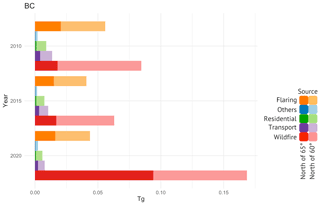
Figure 8Sectoral black carbon emissions above 60∘ N (lighter colours) and 65∘ N (darker colours) for 2010, 2015, and 2020; anthropogenic emissions are from GAINS and wildfire emissions are from GFAS.
Human ignition sources, including predicting future demographic, migration, and/or development patterns in these changing northern landscapes, will impact fire activity and related emissions (Robinne et al., 2016; Riley et al., 2019). For example, consider agricultural landscapes as one source of fire. Expanding climate-driven agricultural frontiers in the high northern latitudes under the RCP8.5 scenario for 2060–2080 could add 8.5 million square kilometre of new croplands in Canada and Russia alone, expanding wheat and maize production into areas with carbon-rich or peat soils (Hannah et al., 2020). Further, Parfenova et al. (2019) found crop growing conditions would be established in some of the permafrost zones of Siberia under RCPs 2.6 and 8.5 by 2080, favourable for wheat and maize (silage) production. These crops are commonly managed via open burning practices in the US, eastern Europe, Russia, and Canada (Kutcher and Malhi, 2010; McCarty et al., 2017; Theesfeld and Jelinek, 2017; Shiwakoti et al., 2019; Thompson and Morrison, 2020). Thus, burning of croplands, grasslands, and deciduous forests often occurs at times when transport of fire emissions to the Arctic is likely, i.e. late winter–early spring for Russia (Hall and Loboda, 2018; Qi and Wang, 2019) as well as Canada and the north central US (Viatte et al., 2015).
While open biomass burning emissions are episodic in nature when considering emissions from single extreme wildland fire events and even wildfire seasons, the spring to early summer human-caused fires are a consistent source of BC and PM2.5 that can be managed and potentially reduced. From the policy perspective, and how these events will contribute to pan-Arctic pollution, fires are important to consider for future Arctic Council collaboration and coordination among member states, Arctic Indigenous Permanent Participants, and non-Arctic observer states. For future Arctic fires, policy controls are effectively limited to fuel management, reduction of human ignitions, and wildland firefighting in the Arctic and the boreal zone (Flannigan et al., 2013). Further, wildland firefighting techniques in the boreal forest will not be appropriate for the more fragile permafrost- and peat-dominated Arctic tundra and will need to be specifically tailored, for example, to the tundra (French et al., 2015). Collaboration, cooperation, and innovation are needed for future Arctic wildland firefighting techniques, practices, and implementation, particularly in the context of potential emissions mitigation.
Here we highlight the key problems summarized from the review of scientific literature in an attempt to focus future research efforts. It is important to reduce the uncertainties below to understand Arctic fire regimes and emissions, especially given that climate change potentially introduces a new fast-moving uncertainty. Improving the understanding of the current and future Arctic and boreal fire regimes will be important for Arctic policymakers as well, given a rapidly changing Arctic and the influence of these fire regimes on climate systems, fragile Arctic ecosystems, and society (Rogers et al., 2020). Overall, a major uncertainty exists in conceptualizing and documenting what constitutes a shift in fire regimes of a certain region or even the pan-Arctic (i.e. current fire climatology versus fuel types) and what happens when a new regime is about to emerge (i.e. future projections of climatic and ecological conditions). Specific recommendations are made in each subsection to propose next steps.
7.1 Spatial and temporal modelling of future fire landscapes and regimes
Modelling future fire landscapes and regimes, in terms of coupled fire–climate–land use–ecology models, remains uncertain. Future Arctic fire regimes will be influenced by shifting vegetation types (Tchebakova et al., 2009; Sizov et al., 2021), with both climate change and subsequent fire seasons, i.e. fire disturbance, determining the species and locations of future vegetation on Arctic and boreal landscapes (Foster et al., 2019). For example, fire and the thawing of permafrost are considered to be the principal mechanisms that will shape new vegetation physiognomies for Siberia (Polikarpov et al., 1998; Tchebakova et al., 2010). It is important to note that moisture from summertime thaw of the active layer of permafrost provides necessary moisture for forest growth in the dry environment of interior Siberia, otherwise only steppe could exist without this additional moisture (Shumilova, 1962). In the dry climate in interior Siberia, frequent fires eliminate any of the dark conifer undergrowth that may have become established in suitable sites within the permafrost zone. The fire return interval in the light conifer (larch, Larix spp., and Scots pine, Pinus sylvestris) middle taiga in central Siberia is 20–30 years (Furyaev et al., 2001), compared to 200–300 years in dark conifer (Siberian pine, Pinus sibirica, and fir, Abies sibirica) forests in southern Siberia, including mountain taiga. Slowly growing dark conifers are not adapted to frequent fires and typically die; additionally, they are not light-tolerant, so they are not likely to be the first species to succeed following fire events. On the other hand, Larix dahurica is evolutionarily adapted to fire and successfully regenerates when cones open following fire events. For east Siberia, Polikarpov et al. (1998) speculated that post-fire succession would mean that dark conifers would be replaced by Scots pine in southern dry climates and by larch on cold soils in a warmer climate. Dark conifers, which survive in specific climatic zones, would shift northwards and eastwards following permafrost retreat, and light-needled tree species (e.g. Pinus sylvestris and Larix sibirica) would follow them, expanding from the south. In the transition zone between dark-needled and light-needled tree species, birch and mixed light conifer–hardwood subtaiga and forest–steppe would dominate, likely reducing fire risk. In the southern tundra of Yamalo-Nenets Autonomous Okrug in northwest Siberia, a transition from dry dwarf shrub to woodlands (<50 % of area is covered by trees) has been documented in previously burned areas (Sizov et al., 2021).
Total area of Siberian forests is predicted to decrease and shift northwards, with forest–steppe and steppe ecosystems predicted to dominate 50 % of Siberia by 2080 under RCP8.5 (Parfenova et al., 2019), meaning agriculture in Siberia would likely benefit from climate warming. About 50 %–85 % of central Siberia was predicted to be climatically suitable for agriculture (Tchebakova et al., 2011), although potential croplands would be limited by availability of suitable soils. Crop production may increase by 2-fold. The introduction of new agricultural crops could likely be less costly than afforestation with new tree species climatypes. Farming may be a preferred land use choice in the future where forests would fail due to climate change, with regional business and economy authorities determining what specific measures may be undertaken to support forestry, agriculture, or mixed agriculture and forestry practices in order to optimize economic loss or gain effects of climate change. Therefore, understanding how climate change and ongoing fire disturbance in the boreal and Arctic will impact species distribution, and thus fuel availability, remains complex (Shuman et al., 2017), and more work in coupled fire–climate–ecology models, with considerations for permafrost and human-driven land use and ignition in emerging agricultural systems, for the Arctic and boreal is needed.
7.2 Peatlands
Peat smouldering can emit large quantities of smoke, contributing to hazardous air quality (Hu et al., 2018). Current global fire emissions inventories underestimate peat fires, as forest fuel types currently drive fuel maps and profiles (Liu et al., 2020). Boreal zone peatland fires are not well quantified in terms of fuel loadings (Van Leuwen et al., 2014). High uncertainty in emission factors for boreal peat fires (Hu et al., 2018) has led to improved laboratory-derived emission factors from sampled peat from Russia and Alaska (Watson et al., 2019). Recent laboratory work on fire mechanisms of organic soils and how peat fires spread improves the understanding of these processes (for example, Huang et al., 2017, 2015; Prat-Guitart, 2016; Huang et al., 2019; Christensen et al., 2020; Santoso et al., 2021; Yuan et al., 2021), though a need for pan-Arctic field observations persists. Burn depth is also not well captured outside of localized spatial scales, like sampling plots, given lack of Earth observation sensing capabilities and pre-fire and post-fire soil surveys (Rogers et al., 2014), which can lead to emissions underestimations.
With a warming climate, there is a risk of increasing peatland and “legacy carbon” fires (Ingram et al., 2019) in boreal forests, particularly in stands younger than 60 years where drying limits the resilience of the carbon-rich soils (Walker et al., 2019), and in drying fen watersheds near large settlements, like the costliest wildfire in Canada's history – the May 2016 Horse River–Fort McMurray fire (Elmes et al., 2018). Future emission estimates from peat fires will need to be informed by where and in what condition these carbon-rich soils reside, particularly as predicted moderate and severe drought in boreal peatlands in western Canada are expected to increase fire size by over 500 % (Thompson et al., 2019). Current Earth system models do not typically characterize well or include peat fires and related feedbacks (Lasslop et al., 2019; Loisel et al., 2020), further limiting our ability to predict future emissions from peatland burning. Mapping pan-Arctic peatlands has proved challenging (Yu et al., 2010; Xu et al., 2018), with recent improvements linking permafrost to peat storage (Hugelius et al., 2020). Further, difficulties in estimating and/or accounting for water table depth and moisture content of peat when modelling depth of burn and associated emissions during smouldering are a key observational uncertainty (Kiely et al., 2019). Future fuel data will need to account for how the complexities of the boreal and Arctic peat topography will impact rate of post-fire peat soil accumulation (Ingram et al., 2019), with some landscapes remaining resilient with other marginal peat areas with severe smouldering and fewer sediment inputs becoming sources of legacy carbon emissions, thus driving future fuel availability. Current Earth system models underestimate evaporative water loss and overestimate current and future water availability for boreal peatland systems under RCP4.5 and 8.5 warming scenarios when compared to current climatic conditions, perhaps underestimating fire risk, activity, and emissions in peat systems (Helbig et al., 2020).
Climate mitigation efforts, like restoration or rewetting of peatlands, do not eliminate the role of fire as a management tool (Davies et al., 2016) nor the risk of wildland fire in peat landscapes. Thus, estimates of future fire emissions will need to assimilate complexities associated with peat fuel conditions and loadings. For example, restoration of peat is not a linear process, with previous results in Canada showing 1 to 2 decades needed for restoration and rewetting of degraded peatlands that have residual peat and vegetation to “seed” the sites (Nugent et al., 2019). Until these restored peatlands have sufficient moisture and vegetation cover, they are still susceptible to fire risk. Burn depth in peat can be limited in naturally wet and rewetted peatlands if the surface maintains a high moisture content via hydrological and vegetation processes (Granath et al., 2016). Maintaining these needed hydrological processes is difficult for degraded, unmanaged peatlands. In Alberta, wildland peat sites lacking constant sources of water and depositional inputs experienced severe burning on margins (Ingram et al., 2019), while Wilkinson et al. (2019) found forested peatland margins were extremely vulnerable to peat smouldering combustion, especially in previously burned areas with >60 years since fire. Ronkainen et al. (2013) expect a warmer climate to lower water tables via evapotranspiration for unmanaged peatlands in Finland, thus increasing wildfire risk. Producing more complete estimates of fuel loadings for peatlands across the Arctic region can follow methodologies set by Johnston et al. (2015) to augment the dynamic boreal, taiga, and tundra fuel loadings, e.g. Innes (2013) and Ivanova et al. (2019).
7.3 Permafrost
Approximately half of all peatlands in the Northern Hemisphere are coincidental with permafrost (Hugelius et al., 2020), with many discontinuous permafrost sites dominated by peatlands in Canada (Estop-Aragonés et al., 2018; Gibson et al., 2018), Russia (Hugelius et al., 2014), and Sweden (Chang et al., 2019). In the flat west Siberian terrain, Kotlyakov and Khromova (2002) and Malevsky-Malevich et al. (2001) show no continuous or discontinuous permafrost below 65∘ N, which influences the viable vegetation for the tundra and sparse Larix sibirica taiga. Current climate models may be missing the link between melting ground ice, sometimes referred to as thermokarst processes, and potential permafrost degradation of the currently stable and carbon-rich northeast Siberian Arctic lowlands (NESAL). Nitzbon et al. (2020) indicate that we can expect a 3-fold increase in permafrost thaw in the NESAL region under RCP4.5 (a stabilization scenario) by 2100 when thermokarst processes are combined with increased temperature projections in numerical modelling, potentially increasing the amount of peat fuels in an already high-fire-activity region. Combining current peatland distribution maps with newer modelled datasets of predicted mid-century and late-century permafrost extent and geohazard indices under climate-forcing scenarios (Karjalainen et al., 2019) can reduce uncertainties to determine (1) increased peat fire risk and locations due to permafrost thaw and (2) decreased capability to deploy ground-level wildland firefighting, thus limiting ability to control future peat fires and fire emissions in the pan-Arctic. Further, permafrost thawing changes hydrology (e.g. greater river discharge or disappearing lakes) and geomorphology (solifluction and thermokarst processes) across broad expanses of the contemporary permafrost zone. In a warmer and drier climate, many locations in the Arctic may be affected by solifluction, with thermokarst modified by frequent catastrophic fires and deeper active layer thaw. As a whole, retreating permafrost should cause a reduction in the area of forests and their replacement by steppe on well-drained, tilted geomorphology (Lawrence and Slater, 2005) or by bogs on poorly drained plains (Tchebakova et al., 2009).
Permafrost areas, especially at their southern distributions, are being disturbed by wildfires (Holloway et al., 2020). In Alaska and northwestern Canada, the impacts of wildfire disturbances on permafrost have been well quantified. For instance, post-fire permafrost change in Alaska showed surface warming greater in boreal sites than tundra, with surface temperatures higher for previously burned sites than at unburned sites, even after vegetation recovered for 1 to 4 decades (Jiang et al., 2017). In the North Slope of Alaska, recent evidence suggests that a transition from grasses to shrubbier conditions is occurring after tundra fires (Jones et al., 2013). Though the vast majority of fires in the continuous and discontinuous permafrost zones occur in deciduous needleleaf forests (Loranty et al., 2016), knowledge gaps on post-fire permafrost resiliency exist for larch-dominated forests (Larix spp.) in Siberia. For instance, recent work in the Sakha Republic found that a 36 km2 wildfire in an open larch with shrub and moss lichen landscape northwest of the Batagaika megaslump resulted in approximately 3.5 million cubic metres of thawed permafrost 5 years later (Yanagiya and Furuya, 2020). Likewise, uncertainties persist for post-fire permafrost resiliency in the boreal forests of eastern Canadian, like Quebec and Labrador (Holloway et al., 2020). As with peatlands, improved geospatial products advance our understanding of the potential for impacts of wildfires across large spatial scales (Hugelius et al., 2020).
7.4 Satellite-based fire emissions
Fire regimes for the boreal regions are often described by impacts on and from fire emissions (Rogers et al., 2020), with many modelling emissions in the high northern latitudes using Earth observations. Uncertainties in emission models are driven by availability and quality of fire activity data from satellite- and ground-based sources, as well as incomplete knowledge of fuels and emission factors. Current global fire emission inventories rely on satellite-derived fire activity from active fire detections, burned area mapping, and fire radiative power (Liu et al., 2020). A comparison of four satellite-based global fire emissions databases over North America – GFED, FINN, GFAS, QFED – found that assumed portions of dry matter in fuels and not emission factors were creating biomass burning aerosol estimates that differ by factors of 4 to 7, essentially limiting the ability to accurately quantify the impact of smoke on climate and air quality (Carter et al., 2020). Given the international scientific community's reliance on two main fire emissions factor sources (Akagi et al., 2011; Andraea, 2019, as an update to Andraea and Merlet, 2001), information available for a robust uncertainty analysis for this variable is limited (Pan et al., 2020).
Satellite-based observations of fire in the Arctic and boreal regions underestimate open burning in agricultural landscapes, surface fires in boreal forests, and smouldering peat fires. For example, current emission inventories based on satellite-derived products of burned area, like GFEDv4, underestimate human-caused burning in agricultural landscapes and mixed forests in Eurasia between 50 and 65∘ N by approximately 2100 km2 annually (Zhu et al., 2017), indicating that actual burned area from anthropogenic ignitions in the Eurasian boreal zone is currently underestimated by as much as 16 %. Surface fires under forest canopies dominate fire regimes in much of northern Eurasia, but these fires are not well quantified in current satellite-based burned area products (Rogers et al., 2015; Duncan et al., 2020) and thus emission inventories. Smouldering fires in carbon-rich humus and peat landscapes will be difficult to detect, as smouldering combustion occurs at much lower temperatures than flaming combustion: 500 to 700 ∘C versus 1500 to 1800 ∘C, respectively (Rein et al., 2008). As previously mentioned, daily, global observations of low-intensity fire from existing satellite systems are currently limited to VIIRS (Johnston et al., 2018), as it was designed to detect smaller and cooler fires than MODIS. For this review, the versions of GFAS, GFED, FINN, FEER, and QFED analysed rely on MODIS thermal anomalies, unlike the custom AMAP fire emissions which used VIIRS only. Smouldering fires in the Arctic can be mapped via regionally tuned algorithms designed to ingest daily active fire detections from multispectral VIIRS (Waigl et al., 2017) and hyperspectral Hyperion (Waigl et al., 2019) sensors. In general, satellite and drone detections (Burke et al., 2019) of smouldering peat fires are difficult because ground fires are low temperature and can burn underground and re-emerge in new locations (Rein, 2016), with additional existing detection constraints from coarse-resolution (>1 km) global satellite sensors, canopy cover, and cloud cover (Johnston et al., 2018).
A further complication is that peat fires can smoulder for months, years, and even decades (Hu et al., 2018), burning laterally and vertically below the surface, appearing to be extinguished, but releasing smoke at the surface in a different location from the original ignition site. This phenomenon is referred to as holdover, overwintered, and/or zombie fires and makes it difficult to allocate as a single – but complex – fire event from cumulative satellite active fire and burned area pixels. For example, in April 2020, the Alaska Division of Forestry was monitoring several active smoldering peat fires from the ∼ 5 km2 Deshka Landing Fire of August 2019 that had overwintered near Willow, Alaska, despite heavy snow melt (Alaska Wildland Fire Information, 2020). Preliminary results by Scholten and Veraverbeke (2020), indicate that overwintering fires are more likely to be holdovers from high-severity fires, emerging more frequently in lowland black-spruce-dominated boreal forests. McCarty et al. (2020) hypothesize that some of the earliest fires along still-frozen thermokarst lakes of the Sakha Republic in May 2020 may be holdover fires, as the drivers and extent of early season human-caused ignitions are still not well documented in the scientific literature for much of the Arctic.
7.5 Lack of agreement between official statistics and satellite observations
Earth observations from satellite products are powerful tools for forecasting (Pickell et al., 2017), improving rapid response post-fire modelling (Miller et al., 2017) and quantifying fire in the boreal and Arctic regions (Hislop et al., 2020). Consistently, however, there has been little correlation between satellite-derived and official estimates of burned area (Fusco et al., 2019). Loepfe et al. (2012) found that multiple satellite fire products had high correlation with official reports of burned areas for Sweden but little to no correlation with official statistics for Finland. Agreement of burned area within Siberian forests between official Russian statistics and four satellite-based burned area products was less than 10 % (Kukavskaya et al., 2013). Average official satellite-derived Russian burned area estimates differ by a mean of 48 % for 2002–2015 in comparison to the Loboda et al. (2017) regionally tuned product, which only differs by a mean of 18 % in comparison to official burned area statistics for Alaska and Canada. One reason for these differences could be that regional-to-global scale algorithms may not have the sensitivity necessary to define surface fire, which is the dominant fire type in Siberia in normal fire years. Also, North American and Nordic countries have long-term ground-based boreal burned area records that span 50 years or more, which aids in calibrating current satellite data records and analysing relationships between fire regimes, vegetation, weather, and climate. Accurate long-term fire records do not exist for much of Russia, primarily because fire was not historically recorded in the remote “unprotected territories” (Sofronov et al., 1998; Soja et al., 2004). Consequently, understanding of the balance between surface-to-crown fire and the ecosystem-dependent areas that burn in Siberia is limited, which adversely affects fire emissions estimates. The Global Wildfire Information System (GWIS; https://gwis.jrc.ec.europa.eu/, last access: 13 September 2021), a joint programme between the Group on Earth Observations (GEO; https://www.earthobservations.org/geoss_wp.php, last access: 13 September 2021), Copernicus (https://www.copernicus.eu/en/services/emergency, last access: 13 September 2021), and NASA (https://www.nasa.gov/, last access: 13 September 2021), uses the MODIS MOD64A1 Collection 6 Burned Area product (Giglio et al., 2018) to create country-level burned area statistics. GWIS satellite-derived burned area overestimates open biomass burning in both Norway and Finland by 199 % and 129 %, respectively, when compared to official statistics (Table 3). However, GWIS underestimates open biomass burning in Sweden by 48 %. The work of the SLCF EG was unable to determine exact reasons for why this mismatch occurs, though previous work has shown that satellite-based fire observations are more likely to align with official records as fire sizes increase (Fusco et al., 2019). Both Norway and Finland reported the lowest fire activity and burned area (Table 1). Future open biomass burning emissions will need improved satellite fire detection methodologies for the Arctic and boreal regions and shorter latency in ground reports and statistics from official agencies. Further, verifying and relating satellite detections of fires to ground-level verification will require a concerted effort and likely lead to a better understanding of how and why these two fire data sources do not presently align.
Prevention and management of pan-Arctic fires are limited to reduction of human-caused ignitions and management of landscape fuels (Flannigan et al., 2013). The impact of humans on fire risk is dependent on local- to national-scale actions that may increase fire and emissions via deforestation, transportation networks, energy extraction, and agricultural open burning as well as decrease fire and fire emissions via active suppression. On a practical level, people are the main ignition sources for fires in the Arctic region, while lightning ignitions tend to lead to larger fires. In interior Alaska, where lightning-caused fires account for 95 % of total burned area (Veraverbeke et al., 2017), 52 % of total ignitions were human in origin but occurred in areas of high fire suppression, resulting in only 5 % of total burned area from 1990–2016 (Calef et al., 2017). Archard et al. (2008) estimated 65 % of all forest fires in the Russian Federation were caused by human ignition, and a more recent study found approximately half of all fires in the Sakha Republic are caused by anthropogenic activities (Kirillina et al., 2020). Throughout boreal Canada, anthropogenic factors increase fire probability (Parisien et al., 2016), with humans igniting most fires close to roads while lightning-caused fires are responsible for the majority of burned area in the more remote locations (Gralewicz et al., 2012). Blouin et al. (2016) found that 45 % of wildfires in Alberta were started by lightning but were responsible for 71 % of burned area. In Finland, lightning-caused fires account for less than 15 % of forest fires (Larjavaara et al., 2005). Machines used for forestry operations in stony areas of Sweden account for 7 %–10 % of total annual ignitions and 40 % of total burned area (Sjöström et al., 2019). For the 19 European countries reporting fires and ignition sources to the European Forest Fire Information System (EFFIS; https://effis.jrc.ec.europa.eu/, last access: 13 September 2021), de Rigo et al. (2017) determined only 4 % of fires were from natural sources, with half of the fire records lacking a verified cause.
Indigenous fire management (IFM) and understanding Indigenous use of fire, as well as fire risk and response to fire events (Mottershead et al., 2020), are needed in a changing Arctic environment. IFM is more frequently being deployed in fire-prone and/or fire-adapted areas (Nikolakis et al., 2020), which accounts for much of the boreal but not necessarily Arctic ecosystems. Cogos et al. (2019) documented historical place names in northern Sweden (e.g. roavve and roavvi) related to historical Saami practices of burning pine heath landscapes to improve long-term foraging of reindeer. Approximately 1 out of every 10 people in the Arctic are Indigenous (Nordregio, 2019), compromising an estimated 15 % of the population of Alaska, 53 % of the Northern Territories of Canada, and 98 % of Greenland, for a total of 1.13 million Indigenous people in the pan-Arctic (Young and Bjerregaard, 2019). Arctic communities are demanding more leadership roles in climate research and applications (Stone, 2020). Research- and experiential-driven recommendations on how to incorporate traditional Indigenous knowledge into Arctic Council working group efforts, including (1) use of participatory methodology, (2) use of Indigenous methodologies, (3) recognition that traditional ecological knowledge is local, (4) application to policy, and (5) cross-cultural understanding (Sidorova, 2020), align well with community- and landscape-driven fire science methodologies needed to predict future fire risk (Bowman et al., 2020; Johnston et al., 2020) and to answer many of the fire regime and emission, including ignition and fuel type, uncertainties raised in this review. Who better to ask – and to lead – than the people who live there?
Since the mid-2000s, emissions from open biomass burning have increased above 60∘ N, with fires above 66∘ N occurring earlier in the year and burning later into the growing season, indicative of a changing Arctic fire regime. Compared to anthropogenic sources in the GAINS model, biomass burning already accounts for more BC and PM2.5 emissions than anthropogenic sources north of 60∘ N, including flaring from associated gas from oil and natural gas extraction. Increased length in fire seasons is coupled with prediction of increased fire severity, with predictions of essentially physically unmanageable crown fires in the boreal as soon as 2050 (Wotton et al., 2017). Future emissions from fires are difficult to predict, and here more work is needed. For example, emissions from functionally uncontrollable fires in boreal forests are not well quantified due to uncertainties in combustion efficiency observations and estimates (Xu et al., 2020). Improving our understanding of the future of Arctic fires and fire emissions will also allow us to better predict future Earth system processes – both at high latitudes and globally.
In contributing to the AMAP 2021 assessment of SLCFs, this review was driven by policy questions identified by member states of the Arctic Council (Table S2) and builds on the 2011 (AMAP, 2011) and 2015 (AMAP, 2015) reports, which included some analysis and discussion of natural, “semi-natural” (i.e. human-caused ignitions in wildland landscapes), and agricultural field burning. We did not perform a systematic review of the fire research literature (Robinne et al., 2020), and the existing literature cited was not assessed for limitations or errors. Further, while the authors attempted to cite published literature and official fire statistics for the seven Arctic Council states experiencing open biomass burning (excluding Iceland), we know that bias may still be present in the over 200 peer-reviewed sources of literature and data chosen for this review (Johnston et al., 2020). This review is a starting point, a foundation for future pan-Arctic research agendas for fire monitoring and needed systematic reviews (Haddaway et al., 2020) of future fire risk, fire emissions, and fire prevention and management in the Arctic – all needed to accurately describe future Arctic fire regimes.
Future Arctic fire regimes will likely be driven by climate change impacts on fuels, including the interactions between peat and permafrost, fire weather, and ignition sources as well as the complexities of climate and fire disturbance changing vegetation types (Tchebakova et al., 2009; Shuman et al., 2017). The consensus of current literature is that climate change and human activity will increase fire risk in the Arctic, via increased lightning strikes; thawing of permafrost; transitions to grasses, taiga, and dry peat; and more human-caused ignitions. In eastern Canada, the northward expansion of deciduous forests will likely decrease fire risk, which may also be true for portions of southern Siberia and Fennoscandia. Human- and lightning-caused fires are likely to increase given expansion of energy extraction, transportation networks, tourism, and climate change. Further, Arctic landscapes are complex, with high levels of localized heterogeneity due to polygonal tundra landforms (Lara et al., 2020), complex and endemic vegetation types and communities (Raynolds et al., 2019), and topography (Morin et al., 2016). Future fire emissions studies will need to integrate multiple datasets to accurately quantify Arctic fire regimes (Masrur et al., 2018), including climate; permafrost conditions; aboveground, surface, and peat fuels; topography; land use; Indigenous and local fire management; seasonality of burns; and ignition sources.
Human activity and communities in the Arctic will need to adapt to increasing fire risk. To prepare for these 21st century changes to the Arctic fire regime, evidence-based fire monitoring and management – including prevention strategies – must incorporate Indigenous and local knowledge in the Arctic. This will require increasing transdisciplinary research (Sidorova, 2020) to understand and predict fire in the north, how humans are and must adapt to a new fire-prone landscape in the Anthropocene (Bowman et al., 2020), and pan-Arctic collaboration and cooperation. Understanding ecological landscape changes, predicted to substantially increase across Asian Russia, is crucial for developing viable strategies for long-term economic and social development in preparation for climate migration and strategic adaptation planning (Parfenova et al., 2019).
The Arctic Council's role as an agent of change in the region is promising, as it has moved its role from policy informing to policy making (Barry et al., 2020). Given the extreme fire season of 2020, an Arctic Council-led initiative for pan-Arctic fire monitoring, prevention, and management is strongly needed for a rapidly changing Arctic (McCarty et al., 2020). Such efforts have started, including the Arctic Wildland Fire Ecology Mapping and Monitoring Project (Arctic FIRE; https://www.caff.is/arcticfire, last access: 13 September 2021) led by the Gwich'in Council International, an Indigenous permanent participant, via the Conservation of Arctic Flora and Fauna (CAFF) working group of the Arctic Council, as well as other Arctic Council activities. Potentially expanding existing efforts or coordinating with new initiatives to incorporate the five other Indigenous Permanent Participants, as well as more efforts from the science and disaster response agencies of the eight member states and the expertise of other Arctic Council working groups, could create the type of community- and Arctic-centric science needed for pan-Arctic fire policies and to increase the capacity for the Indigenous peoples of the Arctic to monitor and protect their Arctic homelands (Wilson, 2020) from fire risk and to adapt to the changing Arctic fire regime.
The GFEDv4s, FINNv1.5, GFASv1.2, QFEDv2.5r1, and FEERv1.0-G1.2 fire emissions data for 2005 through 2018 were downloaded from https://globalfires.earthengine.app/view/firecam (Liu, 2020). The AMAP SLCF EG 2018 pan-Arctic fire emissions database can be downloaded at https://doi.org/10.5281/zenodo.4648723 (Fain and McCarty, 2021), and R code used to compute it can be downloaded at https://github.com/fainjj (last access: 13 September 2021). The 2020 global GFAS emissions data were downloaded from https://apps.ecmwf.int/datasets/data/cams-gfas/ (Copernicus Atmosphere Monitoring Service Information, 2020). Neither the European Commission nor ECMWF is responsible for any use that may be made of the information it contains. GAINS global emission data can be accessed at https://iiasa.ac.at/web/home/research/researchPrograms/air/Global_emissions.html (Klimont and Heyes, 2019).
The supplement related to this article is available online at: https://doi.org/10.5194/bg-18-5053-2021-supplement.
JLM coordinated the review, designed the fire emission models comparison, co-led the creation of the custom AMAP fire emissions model with JJF, archived the literature for the review, wrote the manuscript, and led the revision. JA, VVP, ZK, SE, AV, SRA, NE, NMT, EIP, AJS, and KK provided major efforts in paper design, organization, and revision. VVP, JJF, ZK, and JLM led the GAINS and GFAS analysis. JJF, VVP, SE, and JLM created the figures and supplemental materials, with input from the remaining authors. All authors contributed to interpretation and verification of the review, as well as contributing to the writing of the manuscript.
The authors declare that they have no conflict of interest.
Publisher's note: Copernicus Publications remains neutral with regard to jurisdictional claims in published maps and institutional affiliations.
This article is part of the special issue “Arctic climate, air quality, and health impacts from short-lived climate forcers (SLCFs): contributions from the AMAP Expert Group (ACP/BG inter-journal SI)”. It is not associated with a conference.
This paper was developed as part of the Arctic Monitoring Assessment Programme (AMAP), AMAP 2021 Assessment: Arctic climate, air quality, and health impacts from short-lived climate forcers (SLCFs). The authors would like to thank the Arctic Monitoring and Assessment Programme Secretariat for providing additional input and review. Clayton (“C.J.”) Mescher (meschecj@miamioh.edu) is acknowledged for his contributions in designing and creating components of original scientific artwork used in this paper. The authors would also like to thank the three anonymous reviewers, whose reviews improved this paper.
This research has been supported by Miami University, Ministry for Foreign Affairs of Finland (IBA Forest Fires, decision PC0TQ4BT-53); Business Finland (BC Footprint; grant no. 1462/31/2019); the ACRoBEAR project, funded by the Belmont Forum Climate, Environment and Health (CEH) Collaborative Research Action and the UK Natural Environment Research Council (grant no. NE/T013672/1); the Arctic Monitoring and Assessment Programme (AMAP); the Russian Foundation for Basic Research (RFBR grant no. 19-45-240004); a joint project of the Government of Krasnoyarsk Territory and Russian Foundation for Basic Research (GKT KRFS and RFBR grant no. 20-05-00540); NASA's Weather and Data Analysis programme; and the Climate Adaptation Research Fund from Environment and Climate Change Canada. Portions of this publication were produced with the financial support of the European Union via the EU-funded Action on Black Carbon in the Arctic. Its contents are the sole responsibility of Jessica L. McCarty, Ville-Veikko Paunu, Zbigniew Klimont, and Justin J. Fain and do not necessarily reflect the views of the European Union.
This paper was edited by Sandy Harrison and reviewed by three anonymous referees.
Abatzoglou, J. T. and Williams, A. P.: Impact of anthropogenic climate change on wildfire across western US forests, P. Natl. Acad. Sci. USA, 113, 11770–11775, https://doi.org/10.1073/pnas.1607171113, 2016.
Ahtikoski, A. and Hökkä, H: Intensive forest management – does it pay off financially on drained peatlands?, Can. J. For. Res., 49, 1101–1113, https://doi.org/10.1139/cjfr-2019-0007, 2019.
Akagi, S. K., Yokelson, R. J., Wiedinmyer, C., Alvarado, M. J., Reid, J. S., Karl, T., Crounse, J. D., and Wennberg, P. O.: Emission factors for open and domestic biomass burning for use in atmospheric models, Atmos. Chem. Phys., 11, 4039–4072, https://doi.org/10.5194/acp-11-4039-2011, 2011.
Alaska Division of Forestry: 2019 EOY handout, available at: http://forestry.alaska.gov/Assets/pdfs/firestats/2019 Alaska Fire Statistics.pdf (last access: 13 September 2021), 2020.
Alaska Wildland Fire Information: Despite heavy snow melt, Deshka Landing hot spots still smoldering, available at: https://akfireinfo.com/2020/04/24/despite-heavy-snow-melt-deshka-landing-hot-spots-still-smoldering/ (last access: 13 September 2021), 2020.
Alexander, H. D. and Mack, M. C.: Gap regeneration within mature deciduous forests of Interior Alaska: Implications for future forest change, Forest Ecol. Manage., 396, 35–43, https://doi.org/10.1016/j.foreco.2017.04.005, 2017.
Amann, M., Bertok, I., Borken-Kleefeld, J., Cofala, J., Heyes, C., Höglund-Isaksson, L., Klimont, Z., Nguyen, B., Posch, M., Rafaj, P., and Sandler, R.: Cost-effective control of air quality and greenhouse gases in Europe: Modeling and policy applications, Environ. Model Softw., 26, 1489–1501, https://doi.org/10.1016/j.envsoft.2011.07.012, 2011.
Amann, M., Kiesewetter, G., Schöpp, W., Klimont, Z., Winiwarter, W., Cofala, J., Rafaj, P., Höglund-Isaksson, L., Gomez-Sabriana, A., Heyes, C., and Purohit, P.: Reducing global air pollution: the scope for further policy interventions, Philos. T. R. Soc. A., 378, 20190331, https://doi.org/10.1098/rsta.2019.0331, 2020.
AMAP: AMAP Assessment Report: Arctic Pollution Issues, Arctic Monitoring and Assessment Programme (AMAP), Oslo, Norway, xii+859 pp., available at: https://www.amap.no/documents/doc/amap-assessment-report-arctic-pollution-issues/68 (last access: 13 September 2021), 1998.
AMAP: Assessment 2011: The Impact of Black Carbon on Arctic Climate, Arctic Monitoring and Assessment Programme (AMAP), Oslo, Norway, Technical Report no. 4, available at: https://www.amap.no/documents/download/977/inline (last access: 13 September 2021), 2011.
AMAP: Assessment 2015: Black carbon and ozone as Arctic climate forcers. Arctic Monitoring and Assessment Programme (AMAP), Oslo, Norway, available at: http://hdl.handle.net/11374/1607, 2015.
AMAP: Assessment 2021: Impacts of short-lived climate forcers on Arctic climate, air quality, and human health, Arctic Monitoring and Assessment Programme (AMAP), Tromsø, Norway, available at: https://www.amap.no/documents/doc/impacts-of-short-lived-climate-forcers-on-arctic-climate-air-quality-and-human-health.-summary-for-policy-makers/3512, last access: 13 September 2021.
Andreae, M. O. and Merlet, P.: Emission of trace gases and aerosols from biomass burning, Global Biogeochem. Cy., 15, 955–966, https://doi.org/10.1029/2000GB001382, 2001.
Andreae, M. O.: Emission of trace gases and aerosols from biomass burning – an updated assessment, Atmos. Chem. Phys., 19, 8523–8546, https://doi.org/10.5194/acp-19-8523-2019, 2019.
Achard, F., Eva, H. D., Mollicone, D., and Beuchle, R.: The effect of climate anomalies and human ignition factor on wildfires in Russian boreal forests, Philos. T. R. Soc. B, 363, 2329–2337, https://doi.org/10.1098/rstb.2007.2203, 2008.
Aviales: Information about the forest fire situation on the territory of the constituent entities of the Russian Federation as of 12/31/2019, available at: https://bit.ly/3nolcSK (last access: 13 September 2021), 2019 (in Russian).
Baranchikov, Y. N. and Montgomery, M. E.: Chapter XXXVI – Siberian Moth, in: The use of classical biological control to preserve forests in North America, edited by: Van Driesche, R. and Reardon, R. C., United States Department of Agriculture, Forest Service, Forest Health Technology Enterprise Team, Morgantown, WV, USA, 383–391, 2014.
Barry, T., Daviðsdóttir, B., Einarsson, N., and Young, O. R.: The Arctic Council: an agent of change?, Glob. Environ. Change, 63, 102099, https://doi.org/10.1016/j.gloenvcha.2020.102099, 2020.
Betänkande av 2018 års skogsbrandsutredning: Skogsbränderna sommaren 2018 [Forest fires in summer 2018, in Swedish], Statens offentliga utredningar (SOU) 2019, Stockholm, 1–334, 2019.
Bieniek, P. A., Bhatt, U. S., York, A., Walsh, J. E., Lader, R., Strader, H., Ziel, R., Jandt, R. R., and Thoman, R. L.: Lightning variability in dynamically downscaled simulations of Alaska's present and future summer climate, J, Appl, Meteorol, Climatol,, 59, 1139–1152, https://doi.org/10.1175/JAMC-D-19-0209.1, 2020.
Bintanja, R. and Andry, O.: Towards a rain-dominated Arctic, Nat. Clim. Change, 7, 263–267, https://doi.org/10.1038/nclimate3240, 2017.
Blyakharchuk, T. A., Tchebakova, N. M., Parfenova, E. I., and Soja, A. J.: Potential influence of the late Holocene climate on settled farming versus nomadic cattle herding in the Minusinsk Hollow, south-central Siberia, Environ. Res. Lett., 9, 065004, https://doi.org/10.1088/1748-9326/9/6/065004, 2014.
Blouin, K. D., Flannigan, M. D., Wang, X., and Kochtubajda, B.: Ensemble lightning prediction models for the province of Alberta, Canada, Int. J. Wildland Fire, 25, 421–432, https://doi.org/10.1071/WF15111, 2016.
Boike, J., Grau, T., Heim, B., Günther, F., Langer, M., Muster, S., Gouttevin, I., and Lange, S.: Satellite-derived changes in the permafrost landscape of central Yakutia, 2000–2011: Wetting, drying, and fires, Glob. Planet. Change, 139, 116, https://doi.org/10.1016/j.gloplacha.2016.01.001, 2016.
Bond, T. C., Streets, D. G., Yarber, K. F., Nelson, S. M., Woo, J. H., and Klimont, Z.: A technology-based global inventory of black and organic carbon emissions from combustion, J. Geophys. Res.-Atmos,, 109, 203, https://doi.org/10.1029/2003JD003697, 2004.
Bond, T. C., Doherty, S. J., Fahey, D. W., Forster, P. M., Berntsen, T., DeAngelo, B. J., Flanner, M. G., Ghan, S., Kärcher, B., Koch, D., and Kinne, S.: Bounding the role of black carbon in the climate system: A scientific assessment, J. Geophys. Res.-Atmos., 118, 5380–5552, https://doi.org/10.1002/jgrd.50171, 2013.
Boulanger, Y., Gauthier, S., Gray, D. R., Le Goff, H., Lefort, P., and Morissette, J.: Fire regime zonation under current and future climate over eastern Canada, Ecol. Appl., 23, 904–923, https://doi.org/10.1890/12-0698.1, 2013.
Boulanger, Y., Gauthier, S., and Burton, P. J.: A refinement of models projecting future Canadian fire regimes using homogeneous fire regime zones, Can. J. Forest Res., 44, 365–376, https://doi.org/10.1139/cjfr-2013-0372, 2014.
Bowman, D. M., Kolden, C. A., Abatzoglou, J. T., Johnston, F. H., van der Werf, G. R., and Flannigan, M.: Vegetation fires in the Anthropocene, Nat. Rev. Earth Environ., 1, 500–515, https://doi.org/10.1038/s43017-020-0085-3, 2020.
Box, J. E., Colgan, W. T., Christensen, T. R., Schmidt, N. M., Lund, M., Parmentier, F. J. W., Brown, R., Bhatt, U. S., Euskirchen, E. S., Romanovsky, V. E., and Walsh, J. E.: Key indicators of Arctic climate change: 1971–2017, Environ. Res. Lett., 14, 045010, https://doi.org/10.1088/1748-9326/aafc1b, 2019.
Burke, C., Wich, S., Kusin, K., McAree, O., Harrison, M.E., Ripoll, B., Ermiasi, Y., Mulero-Pázmány, M., and Longmore, S.: Thermal-Drones as a Safe and Reliable Method for Detecting Subterranean Peat Fires, Drones, 3, 23, https://doi.org/10.3390/drones3010023, 2019.
Calef, M. P., Varvak, A., and McGuire, A. D.: Differences in human versus lightning fires between urban and rural areas of the boreal forest in interior Alaska, Forests, 8, 422, https://doi.org/10.3390/f8110422, 2017.
Carter, T. S., Heald, C. L., Jimenez, J. L., Campuzano-Jost, P., Kondo, Y., Moteki, N., Schwarz, J. P., Wiedinmyer, C., Darmenov, A. S., da Silva, A. M., and Kaiser, J. W.: How emissions uncertainty influences the distribution and radiative impacts of smoke from fires in North America, Atmos. Chem. Phys., 20, 2073–2097, https://doi.org/10.5194/acp-20-2073-2020, 2020.
Cartier, K. M. S.: Southern Greenland wildfire extinguished, Eos, 98, https://doi.org/10.1029/2017EO080905, 2017.
Chang, K. Y., Riley, W. J., Crill, P. M., Grant, R. F., Rich, V. I., and Saleska, S. R.: Large carbon cycle sensitivities to climate across a permafrost thaw gradient in subarctic Sweden, The Cryosphere, 13, 647– 663, https://doi.org/10.5194/tc-13-647-2019, 2019.
Chernokulsky, A. and Esau, I: Cloud cover and cloud types in the Eurasian Arctic in 1936–2012, Int. J. Climatol., 39, 5771–5790, https://doi.org/10.1002/joc.6187, 2019.
Christensen, E. G., Fernandez-Anez, N., and Rein, G.: Influence of soil conditions on the multidimensional spread of smouldering combustion in shallow layers, Combust Flame, 214, 361–370, https://doi.org/10.1016/j.combustflame.2019.11.001, 2020.
CIFFC: Canadian Interagency Forest Fire Centre: Fire Hectares by Year, available at: https://ciffc.net/en/ext/hectares-by-year (last access: 13 September 2021), 2020.
Cogos, S., Östlund, L. and Roturier, S.: Forest fire and indigenous Sami land use: place names, fire dynamics, and ecosystem change in Northern Scandinavia, Human Ecol., 47, 51–64, https://doi.org/10.1007/s10745-019-0056-9, 2019.
Comer, B., Osipova, L., Georgeff, E., and Mao, X.: The International Maritime Organization's proposed Arctic heavy fuel oil ban: Likely implications and opportunities for improvement, International Council on Clean Transportation, available at: https://theicct.org/sites/default/files/publications/Arctic-HFO-ban-sept2020.pdf (last access: 13 September 2021), 2020.
Conard, S. G. and Ivanova, G. A.: Wildfire in Russian boreal forests – Potential impacts of fire regime characteristics on emissions and global carbon balance estimates, Environ. Pollut., 98, 305, https://doi.org/10.1016/S0269-7491(97)00140-1, 1997.
Conard, S. G. and Ponomarev, E.: Fire in the North, Wildfire Magazine, available at: https://www.iawfonline.org/article/fire-in-the-north-the-2020-siberian-fire-season/ (last access: 13 September 2021), 2020.
Copernicus Atmosphere Monitoring Service Information: CAMS GFAS, ECMWF [data set], available at: https://apps.ecmwf.int/datasets/data/cams-gfas/ (last access 13 September 2021), 2020.
Daanen, R. P., Ingeman-Nielsen, T., Marchenko, S. S., Romanovsky, V. E., Foged, N., Stendel, M., Christensen, J. H., and Hornbech Svendsen, K.: Permafrost degradation risk zone assessment using simulation models, The Cryosphere, 5, 1043–1056, https://doi.org/10.5194/tc-5-1043-2011, 2011.
Davies, G. M., Kettridge, N., Stoof, C. R., Gray, A., Ascoli, D., Fernandes, P. M., Marrs, R., Allen, K. A., Doerr, S. H., Clay, G. D., and McMorrow, J.: The role of fire in UK peatland and moorland management: the need for informed, unbiased debate, Philos. T. R. Soc. Lond. B, 371, 20150342, https://doi.org/10.1098/rstb.2015.0342, 2016.
de Groot, W. J., Flannigan, M. D., and Stocks, B. J.: Climate change and wildfires, González-Cabán, Armando, tech. coord. Proceedings of the fourth international symposium on fire economics, planning, and policy: climate change and wildfires, available at: https://www.fs.fed.us/psw/publications/documents/psw_gtr245/psw_gtr245_001.pdf (last access: 13 September 2021), 2013.
de Rigo, D., Libertà, G., Houston Durrant, T., Artés Vivancos, T., and San-Miguel-Ayanz, J.: Forest fire danger extremes in Europe under climate change: variability and uncertainty, Publication Office of the European Union, Luxembourg, https://doi.org/10.2760/13180, 2017.
Dronin, N. and Kirilenko, A.: Climate change, food stress, and security in Russia, Reg. Environ. Change, 11, 167–178, https://doi.org/10.1007/s10113-010-0165-x, 2011.
DSB: Direktoratet for samfunnssikkerhet og beredskap, Personal communication, March 2020, availabe at: https://www.dsb.no/ (last access: 13 September 2021), 2020.
Duncan, B. N., Ott, L. E., Abshire, J. B., Brucker, L., Carroll, M. L., Carton, J., Comiso, J. C., Dinnat, P., Forbes, E. P., Gonsamo, B. C., Gregg, A., Hall, W. W., Ialongo, D. K., Jandt, I., Kahn, R., Karpechko, R. A., Kawa, A., Kato, S. R., Kumpula, T., Kyrölä, E., Loboda, T. V., McDonald, K. C., Montesano, P. M., Nassar, R., Neigh, C. S. R., Parkinson, C. L., Poulter, B., Pulliainen, J., Rautiainen, K., Rogers, B. M., Rousseaux, C. S., Soja, A. J., Steiner, N., Tamminen, J., Taylor, P. C., Tzortziou, M. A., Virta, H., Wang, J. S., Watts, J. D., Winker, D. M., and Wu, D. L.: Space-Based Observations for Understanding Changes in the Arctic-Boreal Zone, Rev. Geophys., 58, e2019RG000652, https://doi.org/10.1029/2019RG000652, 2020.
Elmes, M. C., Thompson, D. K., Sherwood, J. H., and Price, J. S.: Hydrometeorological conditions preceding wildfire, and the subsequent burning of a fen watershed in Fort McMurray, Alberta, Canada, Nat. Hazards Earth Syst. Sci., 18, 157–170, https://doi.org/10.5194/nhess-18-157-2018, 2018.
Estop-Aragonés, C., Czimczik, C. I., Heffernan, L., Gibson, C., Walker, J. C., Xu, X., and Olefeldt, D.: Respiration of aged soil carbon during fall in permafrost peatlands enhanced by active layer deepening following wildfire but limited following thermokarst, Environ. Res. Lett., 13, 085002, https://doi.org/10.1088/1748-9326/aad5f0, 2018.
Evangeliou, N., Kylling, A., Eckhardt, S., Myroniuk, V., Stebel, K., Paugam, R., Zibtsev, S., and Stohl, A.: Open fires in Greenland in summer 2017: transport, deposition and radiative effects of BC, OC and BrC emissions, Atmos. Chem. Phys., 19, 1393–1411, https://doi.org/10.5194/acp-19-1393-2019, 2019.
Fain, J. and McCarty, J.: AMAP SLCF EG Pan-Arctic Fire Emissions Model, version 1.0.0 Data Set, Zenodo [data set], https://doi.org/10.5281/zenodo.4648723, 2021.
Fisher, J. A., Jacob, D. J., Purdy, M. T., Kopacz, M., Le Sager, P., Carouge, C., Holmes, C. D., Yantosca, R. M., Batchelor, R. L., Strong, K., Diskin, G. S., Fuelberg, H. E., Holloway, J. S., Hyer, E. J., McMillan, W. W., Warner, J., Streets, D. G., Zhang, Q., Wang, Y., and Wu, S.: Source attribution and interannual variability of Arctic pollution in spring constrained by aircraft (ARCTAS, ARCPAC) and satellite (AIRS) observations of carbon monoxide, Atmos. Chem. Phys., 10, 977–996, https://doi.org/10.5194/acp-10-977-2010, 2010.
Flannigan, M., Cantin, A. S., De Groot, W. J., Wotton, M., Newbery, A., and Gowman, L. M.: Global wildland fire season severity in the 21st century, Forest Ecol. Manag., 294, 54–61, https://doi.org/10.1016/j.foreco.2012.10.022, 2013.
Foster, A. C., Armstrong, A. H., Shuman, J. K., Shugart, H. H., Rogers, B. M., Mack, M. C., Goetz, S. J., and Ranson, K. J.: Importance of tree-and species-level interactions with wildfire, climate, and soils in interior Alaska: Implications for forest change under a warming climate, Ecol. Model., 409, 108765, https://doi.org/10.1016/j.ecolmodel.2019.108765, 2019.
French, N. H., Jenkins, L. K., Loboda, T. V., Flannigan, M., Jandt, R., Bourgeau-Chavez, L. L., and Whitley, M.: Fire in arctic tundra of Alaska: past fire activity, future fire potential, and significance for land management and ecology, Int. J. Wildland Fire, 24, 1045–1061, https://doi.org/10.1071/wf14167, 2015.
Furyaev, V. V.: Pyrological regimes and dynamics of the southern taiga forests in Siberia, in: Fire in ecosystems of boreal Eurasia, edited by: Goldammer, J. G. and Furyaev, V. V., Springer, Dordrecht, the Netherlands, 168–185, https://doi.org/10.1007/978-94-015-8737-2_12, 1996.
Furyaev, V. V., Vaganov, E. A., Tchebakova, N. M., and Valendik, E. N.: Effects of fire and climate on successions and structural changes in the Siberian boreal forest, Eurasian J. Forest Res., 2, 1–15, 2001.
Fusco, E. J., Finn, J. T., Abatzoglou, J. T., Balch, J. K., Dadashi, S., and Bradley, B. A.: Detection rates and biases of fire observations from MODIS and agency reports in the conterminous United States, Remote Sens. Environ., 220, 30–40, https://doi.org/10.1016/j.rse.2018.10.028, 2019.
Gibson, C. M., Chasmer, L. E., Thompson, D. K., Quinton, W. L., Flannigan, M. D., and Olefeldt, D.: Wildfire as a major driver of recent permafrost thaw in boreal peatlands, Nat. Commun., 9, 1–9, https://doi.org/10.1038/s41467-018-05457-1 , 2018.
Giglio, L., Loboda, T., Roy, D. P., Quayle, B., and Justice, C. O.: An active-fire based burned area mapping algorithm for the MODIS sensor, Remote Sens. Environ., 113, 408–420, https://doi.org/10.1016/j.rse.2008.10.006, 2009.
Giglio, L., Schroeder, W.m and Justice, C.O.: The collection 6 MODIS active fire detection algorithm and fire products, Remote Sens. Environ, 178, 31–41, https://doi.org/10.1016/j.rse.2016.02.054, 2016.
Giglio, L., Boschetti, L., Roy, D. P., Humber, M. L., and Justice, C. O.: The Collection 6 MODIS burned area mapping algorithm and product, Remote Sens. Environ., 217, 72–85, https://doi.org/10.1016/j.rse.2018.08.005, 2018.
Gralewicz, N. J., Nelson, T. A., and Wulder, M. A.: Factors influencing national scale wildfire susceptibility in Canada, Forest Ecol. Manag., 265, 20–29, https://doi.org/10.1016/j.foreco.2011.10.031, 2012.
Granath, G., Moore, P. A., Lukenbach, M. C., and Waddington, J. M.: Mitigating wildfire carbon loss in managed northern peatlands through restoration, Sci. Rep., 6, 1–9, https://doi.org/10.1038/srep28498, 2016.
Granström, A. and Niklasson, M.: Potentials and limitations for human control over historic fire regimes in the boreal forest, Philos. T. R. Soc. Lond. B, 363, 2351–2356, https://doi.org/10.1098/rstb.2007.2205, 2008.
Groenemeijer, P., Vajda, A., Lehtonen, I., Kämäräinen, M., Venäläinen, A., Gregow, H., Becker, N., Nissen, K., Ulbrich, U., Paprotny, D., and Morales Napoles, O.: Present and future probability of meteorological and hydrological hazards in Europe, Final report of Deliverable 2.5 for the Risk Analysis of Infrastructure Networks in response to extreme weather (RAIN) project, available at: http://rain-project.eu/wp-content/uploads/2016/09/D2.5_REPORT_final.pdf (last access: 13 September 2021), 2016.
Gromny, E., Lewiński, S., Rybicki, M., Malinowski, R., Krupiński, M., Nowakowski, A., and Jenerowicz, M.: Creation of training dataset for Sentinel-2 land cover classification, in: Photonics Applications in Astronomy, Communications, Industry, and High-Energy Physics Experiments 2019, Vol. 11176, p. 111763D, International Society for Optics and Photonics, available at: http://s2glc.cbk.waw.pl/ (last access: 13 September 2021), 2019.
Günther, A., Barthelmes, A., Huth, V., Joosten, H., Jurasinski, G., Koebsch, F., and Couwenberg, J.: Prompt rewetting of drained peatlands reduces climate warming despite methane emissions, Nat. Commun., 11, 1644, https://doi.org/10.1038/s41467-020-15499-z, 2020.
Haddaway, N. R., Bethel, A., Dicks, L. V., Koricheva, J., Macura, B., Petrokofsky, G., Pullin, A. S., Savilaakso, S., and Stewart, G. B.: Eight problems with literature reviews and how to fix them, Nat. Ecol. Evol., 4, 1582, https://doi.org/10.1038/s41559-020-01295-x, 2020.
Hall, J. V. and Loboda, T. V.: Quantifying the Potential for Low-Level Transport of Black Carbon Emissions from Cropland Burning in Russia to the Snow-Covered Arctic, Front. Earth Sci., 5, 109, https://doi.org/10.3389/feart.2017.00109, 2017.
Hall, J. and Loboda, T.: Quantifying the variability of potential black carbon transport from cropland burning in Russia driven by atmospheric blocking events, Environ. Res. Lett., 13, 055010, https://doi.org/10.1088/1748-9326/aabf65, 2018.
Hanes, C. C., Wang, X., Jain, P., Parisien, M. A., Little, J. M., and Flannigan, M. D.: Fire-regime changes in Canada over the last half century, Can. J. Forest Res., 49, 256, https://doi.org/10.1139/cjfr-2018-0293, 2019.
Hannah, L., Roehrdanz, P. R., KC, K. B., Fraser, E. D., Donatti, C. I., Saenz, L., Wright, T. M., Hijmans, R. J., Mulligan, M., Berg, A., and van Soesbergen, A.: The environmental consequences of climate-driven agricultural frontiers, PLoS One, 15, e0228305, https://doi.org/10.1371/journal.pone.0228305, 2020.
Hayasaka, H., Sokolova, G. V., Ostroukhov, A., and Naito, D: Classification of Active Fires and Weather Conditions in the Lower Amur River Basin, Rem. Sens., 12, 3204, https://doi.org/10.3390/rs12193204, 2020.
Helbig, M., Waddington, J. M., Alekseychik, P., Amiro, B. D., Aurela, M., Barr, A. G., Black, T. A., Blanken, P. D., Carey, S. K., Chen, J., and Chi, J.: Increasing contribution of peatlands to boreal evapotranspiration in a warming climate, Nat. Clim. Change, 10, 555, https://doi.org/10.1038/s41558-020-0763-7, 2020.
Hislop, S., Haywood, A., Jones, S., Soto-Berelov, M., Skidmore, A., and Nguyen, T. H.: A satellite data driven approach to monitoring and reporting fire disturbance and recovery across boreal and temperate forests, Int. J. Appl. Earth Obs., 87, 102034, https://doi.org/10.1016/j.jag.2019.102034, 2020.
Höglund-Isaksson, L., Gómez-Sanabria, A., Klimont, Z., Rafaj, P., and Schöpp, W.: Technical potentials and costs for reducing global anthropogenic methane emissions in the 2050 timeframe – results from the GAINS model, Environ. Res. Commun., 2, 025004, https://doi.org/10.1088/2515-7620/ab7457, 2020.
Holloway, J. E., Lewkowicz, A. G., Douglas, T. A., Li, X., Turetsky, M. R., Baltzer, J. L., and Jin, H.: Impact of wildfire on permafrost landscapes: A review of recent advances and future prospects, Permafrost Periglac., 31, 371–382, https://doi.org/10.1002/ppp.2048, 2020.
Hu, F. S., Higuera, P. E., Duffy, P., Chipman, M. L., Rocha, A. V., Young, A. M., Kelly, R., and Dietze, M. C.: Arctic tundra fires: natural variability and responses to climate change, Front. Ecol. Environ., 13, 369–377, https://doi.org/10.1890/150063,2015.
Hu, Y., Fernandez-Anez, N., Smith, T. E., and Rein, G.: Review of emissions from smouldering peat fires and their contribution to regional haze episodes, Int. J. Wildland Fire, 27, 293, https://doi.org/10.1071/wf17084, 2018.
Huang, X. and Rein, G.: Computational study of critical moisture and depth of burn in peat fires, Int. J. Wildland Fire, 24, 798–808, https://doi.org/10.1071/WF14178, 2015.
Huang, X. and Rein, G.: Downward spread of smouldering peat fire: the role of moisture, density and oxygen supply, Int. J. Wildland Fire., 26, 907–918, https://doi.org/10.1071/WF16198, 2017.
Huang, X. and Rein, G.: Upward-and-downward spread of smoldering peat fire, Proc. Combust Inst., 37, 4025–4033, https://doi.org/10.1016/j.proci.2018.05.125, 2019.
Hugelius, G., Strauss, J., Zubrzycki, S., Harden, J. W., Schuur, E. A. G., Ping, C.-L., Schirrmeister, L., Grosse, G., Michaelson, G. J., Koven, C. D., O'Donnell, J. A., Elberling, B., Mishra, U., Camill, P., Yu, Z., Palmtag, J., and Kuhry, P.: Estimated stocks of circumpolar permafrost carbon with quantified uncertainty ranges and identified data gaps, Biogeosciences, 11, 6573–6593, https://doi.org/10.5194/bg-11-6573-2014, 2014.
Hugelius, G., Loisel, J., Chadburn, S., Jackson, R. B., Jones, M., MacDonald, G., Marushchak, M., Olefeldt, D., Packalen, M., Siewert, M. B., and Treat, C.: Large stocks of peatland carbon and nitrogen are vulnerable to permafrost thaw, P. Natl. Acad. Sci., 117, 20438, https://doi.org/10.1073/pnas.1916387117, 2020.
Ichoku, C. and Ellison, L.: Global top-down smoke-aerosol emissions estimation using satellite fire radiative power measurements, Atmos. Chem. Phys., 14, 6643–6667, https://doi.org/10.5194/acp-14-6643-2014, 2014.
Ingram, R. C., Moore, P. A., Wilkinson, S., Petrone, R. M., and Waddington, J. M.: Postfire soil carbon accumulation does not recover boreal peatland combustion loss in some hydrogeological settings, J. Geophys. Res.-Biogeo., 124, 775, https://doi.org/10.1029/2018jg004716, 2019.
Innes, R. J.: Fire regimes of Alaskan tundra communities, U.S. Department of Agriculture, Forest Service, Rocky Mountain Research Station, Fire Sciences Laboratory (Producer), available at: https://www.fs.fed.us/database/feis/fire_regimes/AK_tundra/all.html (last access: 13 September 2021), 2013.
Inness, A., Ades, M., Agustí-Panareda, A., Barré, J., Benedictow, A., Blechschmidt, A.-M., Dominguez, J. J., Engelen, R., Eskes, H., Flemming, J., Huijnen, V., Jones, L., Kipling, Z., Massart, S., Parrington, M., Peuch, V.-H., Razinger, M., Remy, S., Schulz, M., and Suttie, M.: The CAMS reanalysis of atmospheric composition, Atmos. Chem. Phys., 19, 3515–3556, https://doi.org/10.5194/acp-19-3515-2019, 2019.
Ioffe, G. and Nefedova, T.: Marginal farmland in European Russia, Eurasian Geogr. Econ., 45, 45–49, https://doi.org/10.2747/1538-7216.45.1.45, 2004.
IPCC: Climate Change 2013: The Physical Science Basis. Contribution to the Fifth Assessment Report of the Intergovernmental Panel on Climate Change, edited by: Stocker, T. F., Qin, D., Plattner, G.-K., Tignor, M., Allen, S. K., Boschung, J., Nauels, A., Xia, Y., Bex, V. and Midgley, P. M., available at: https://www.ipcc.ch/report/ar5/wg1/ (last access: 13 September 2021), 2013.
Ivanova, G. A., Kukavskaya, E. A., Ivanov, V. A., Conard, S. G., and McRae, D. J.: Fuel characteristics, loads and consumption in Scots pine forests of central Siberia, J. Forest Res., 31, 2507, https://doi.org/10.1007/s11676-019-01038-0, 2019
Jain, P., Tye, M. R., Paimazumder, D., and Flannigan, M.: Downscaling fire weather extremes from historical and projected climate models, Climatic Change, 163, 1–28, https://doi.org/10.1007/s10584-020-02865-5, 2020.
Jenkins, M. J., Runyon, J. B., Fettig, C. J., Page, W. G., and Bentz, B. J.: Interactions among the mountain pine beetle, fires, and fuels, Forest Sci., 60, 489–501, https://doi.org/10.5849/forsci.13-017, 2014.
Jiang, Y., Rocha, A. V., O'Donnell, J. A., Drysdale, J. A., Rastetter, E. B., Shaver, G. R., and Zhuang, Q.: Contrasting soil thermal responses to fire in Alaskan tundra and boreal forest, J. Geophys. Res.-Earth. Surf., 120, 363, https://doi.org/10.1002/2014jf003180, 2015.
Johnston, D. C., Turetsky, M. R., Benscoter, B. W., and Wotton, B. M.: Fuel load, structure, and potential fire behaviour in black spruce bogs, Can. J. Forest Res., 45, 888, https://doi.org/10.1139/cjfr-2014-0334, 2015.
Johnston, J. M., Johnston, L. M., Wooster, M. J., Brookes, A., McFayden, C., and Cantin, A. S.: Satellite detection limitations of sub-canopy smouldering wildfires in the North American Boreal Forest, Fire, 1, 28, https://doi.org/10.3390/fire1020028, 2018.
Johnston, L.M., Wang, X., Erni, S., Taylor, S.W., McFayden, C.B., Oliver, J.A., Stockdale, C., Christianson, A., Boulanger, Y., Gauthier, S., and Arseneault, D.: Wildland fire risk research in Canada, Environ. Rev., 28, 164, https://doi.org/10.1139/er-2019-0046, 2020.
Jones, B. M., Breen, A. L., Gaglioti, B. V., Mann, D. H., Rocha, A. V., Grosse, G., Arp, C. D., Kunz, M. L., and Walker, D. A.: Identification of unrecognized tundra fire events on the north slope of Alaska, J. Geophys. Res.-Biogeo., 118, 1334, https://doi.org/10.1002/jgrg.20113, 2013.
Jones, B. M., Grosse, G., Arp, C. D., Miller, E., Liu, L., Hayes, D. J., and Larsen, C. F.: Recent Arctic tundra fire initiates widespread thermokarst development, Sci. Rep., 5, 15865, https://doi.org/10.1038/srep15865, 2015.
Kaiser, J. W., Heil, A., Andreae, M. O., Benedetti, A., Chubarova, N., Jones, L., Morcrette, J. J., Razinger, M., Schultz, M. G., Suttie, M., and Van Der Werf, G. R.: Biomass burning emissions estimated with a global fire assimilation system based on observed fire radiative power, Biogeosciences, 9, 527–554, https://doi.org/10.5194/bg-9-527-2012, 2012.
Karjalainen, O., Aalto, J., Luoto, M., Westermann, S., Romanovsky, V. E., Nelson, F. E., Etzelmüller, B., and Hjort, J.: Circumpolar permafrost maps and geohazard indices for near-future infrastructure risk assessments, Sci. Data, 6, 190037, https://doi.org/10.1038/sdata.2019.37 , 2019.
Keegan, K. M., Albert, M. R., McConnell, J. R., and Baker, I.: Climate change and forest fires synergistically drive widespread melt events of the Greenland Ice Sheet, P. Natl. Acad. Sci. USA, 111, 7964, https://doi.org/10.1073/pnas.1405397111, 2014.
Kellomäki, S., Strandman, H., Heinonen, T., Asikainen, A., Venäläinen, A., and Peltola, H.: Temporal and spatial change in diameter growth of boreal Scots pine, Norway spruce, and birch under recent-generation (CMIP5) global climate model projections for the 21st century, Forests, 9, 118, https://doi.org/10.3390/f9030118, 2018.
Ketola, J.: Forest fire activity and burned area for Finland, Emergency Services Academy, Personal communication to Henrik Lindberg, based on rescue service database PRONTO, available at: https://prontonet.fi/Pronto3/online3/OnlineTilastot.htm (last access: 13 September 2021), 2020.
Kicklighter, D. W., Cai, Y., Zhuang, Q., Parfenova, E. I., Paltsev, S., Sokolov, A. P., Melillo, J. M., Reilly, J. M., Tchebakova, N. M., and Lu, X.: Potential influence of climate-induced vegetation shifts on future land use and associated land carbon fluxes in Northern Eurasia, Environ. Res. Lett., 9, 035004, https://doi.org/10.1088/1748-9326/9/3/035004, 2014.
Klimont, Z., Kupiainen, K., Heyes, C., Purohit, P., Cofala, J., Rafaj, P., Borken-Kleefeld, J., and Schöpp, W.: Global anthropogenic emissions of particulate matter including black carbon, Atmos. Chem. Phys., 17, 8681, https://doi.org/10.5194/acp-17-8681-2017, 2017.
Kharuk, V. I., Im, S. T., Ranson, K. J., and Yagunov, M. N.: Climate-Induced Northerly Expansion of Siberian Silkmoth Range, Forests, 8, 301, https://doi.org/10.3390/f8080301, 2017.
Kharuk, V. I., Ponomarev, E. I., Ivanova, G. A., Dvinskaya, M. L., Coogan, S. C., and Flannigan, M. D.: Wildfires in the Siberian taiga, Ambio, 1, 1–22, https://doi.org/10.1007/s13280-020-01490-x, 2021.
Kieft, J., Smith, T., Someshwar, S., and Boer, R.: Towards Anticipatory Management of Peat Fires to Enhance Local Resilience and Reduce Natural Capital Depletion, Ecosystem-Based Disaster Risk Reduction and Adaptation in Practice, Springer, 2016.
Kiely, L., Spracklen, D. V., Wiedinmyer, C., Conibear, L., Reddington, C. L., Archer-Nicholls, S., Lowe, D., Arnold, S. R., Knote, C., Khan, M. F., Latif, M. T., Kuwata, M., Budisulistiorini, S. H., and Syaufina, L.: New estimate of particulate emissions from Indonesian peat fires in 2015, Atmos. Chem. Phys., 19, 11105–11121, https://doi.org/10.5194/acp-19-11105-2019f, 2019.
King, M., Altdorff, D., Li, P., Galagedara, L., Holden, J., and Unc, A.: Northward shift of the agricultural climate zone under 21st-century global climate change, Sci. Rep., 8, 7904, https://doi.org/10.1038/s41598-018-26321-8, 2018.
Kirchmeier-Young, M. C., Gillett, N. P., Zwiers, F. W., Cannon, A. J., and Anslow, F. S.: Attribution of the Influence of Human-Induced Climate Change on an Extreme Fire Season, Earths Future, 7, 2–10, https://doi.org/10.1029/2018ef001050, 2019.
Kirillina, K., Shvetsov, E. G., Protopopova, V. V., Thiesmeyer, L., and Yan, W.: Consideration of anthropogenic factors in boreal forest fire regime changes during rapid socio-economic development: case study of forestry districts with increasing burnt area in the Sakha Republic, Russia, Environ. Res. Lett., 15, 035009, https://doi.org/10.1088/1748-9326/ab6c6e, 2020.
Klimont, Z. and Heyes, C.: GAINS Global emission fields of air pollutants and GHGs, IIASA [data set], available at: https://iiasa.ac.at/web/home/research/researchPrograms/air/Global_emissions.html, (last access: 13 September 2021), 2019.
Knorr, W., Dentener, F., Hantson, S., Jiang, L., Klimont, Z., and Arneth, A.: Air quality impacts of European wildfire emissions in a changing climate, Atmos. Chem. Phys., 16, 5685–5703, https://doi.org/10.5194/acp-16-5685-2016, 2016.
Koster, R. D., Darmenov, A. S., and da Silva, A. M.: The Quick Fire Emissions Dataset (QFED): Documentation of Versions 2.1, 2.2 and 2.4, Technical Report Series on Global Modeling and Data Assimilation, available at: https://ntrs.nasa.gov/search.jsp?R=20180005253 (last access: 13 September 2021), 2015.
Kotlyakov, V. and Khromova, T.: Land Resources of Russia – Maps of Permafrost and Ground Ice, Boulder, Colorado USA: National Snow and Ice Data Center, available at: https://nsidc.org/data/GGD600/versions/1 (last access: 13 September 2021), 2002.
Krause, A., Kloster, S., Wilkenskjeld, S., and Paeth, H.: The sensitivity of global wildfires to simulated past, present, and future lightning frequency, J. Geophys. Res.-Biogeo., 119, 312, https://doi.org/10.1002/2013jg002502, 2014.
Krawchuk, M. A. and Moritz, M. A.: Constraints on global fire activity vary across a resource gradient, Ecology, 92, 121–132, 2011.
Krawchuk, M. A., Cumming, S. G., Flannigan, M. D., and Wein, R. W.: Biotic and abiotic regulation of lightning fire initiation in the mixedwood boreal forest, Ecology, 87, 458–468, https://doi.org/10.1890/05-1021, 2006.
Krawchuk, M. A., Moritz, M. A., Parisien, M. A., Van Dorn, J., and Hayhoe, K.: Global pyrogeography: the current and future distribution of wildfire, PLOS ONE, 4, e5102, https://doi.org/10.1371/journal.pone.0005102, 2009.
Kukavskaya, E. A., Soja, A. J., Petkov, A. P., Ponomarev, E. I., Ivanova, G. A., and Conard, S. G.: Fire emissions estimates in Siberia: evaluation of uncertainties in area burned, land cover, and fuel consumption, Can. J. Forest Res., 43, 493, https://doi.org/10.1139/cjfr-2012-0367, 2013.
Kukavskaya, E. A., Buryak, L. V., Shvetsov, E. G., Conard, S. G., and Kalenskaya, O. P.: The impact of increasing fire frequency on forest transformations in southern Siberia, Forest Ecol. Manag., 382, 225, https://doi.org/10.1016/j.foreco.2016.10.015, 2016.
Kutcher, H. R. and Malhi, S. S.: Residue burning and tillage effects on diseases and yield of barley (Hordeum vulgare) and canola (Brassica napus), Soil Till. Res., 109, 153–160, https://doi.org/10.1016/j.still.2010.06.001, 2010.
Lamarque, J.-F., Bond, T. C., Eyring, V., Granier, C., Heil, A., Klimont, Z., Lee, D., Liousse, C., Mieville, A., Owen, B., Schultz, M. G., Shindell, D., Smith, S. J., Stehfest, E., Van Aardenne, J., Cooper, O. R., Kainuma, M., Mahowald, N., McConnell, J. R., Naik, V., Riahi, K., and van Vuuren, D. P.: Historical (1850–2000) gridded anthropogenic and biomass burning emissions of reactive gases and aerosols: methodology and application, Atmos. Chem. Phys., 10, 7017–7039, https://doi.org/10.5194/acp-10-7017-2010, 2010.
Lara, M. J., McGuire, A. D., Euskirchen, E. S., Genet, H., Yi, S., Rutter, R., Iversen, C., Sloan, V., and Wullschleger, S. D.: Local-scale Arctic tundra heterogeneity affects regional-scale carbon dynamics, Nat. Commun., 11, 4925, https://doi.org/10.1038/s41467-020-18768-z, 2020.
Larjavaara, M., Kuuluvainen, T., and Rita, H.: Spatial distribution of lightning-ignited forest fires in Finland, Forest Ecol. Manag., 208, 177, https://doi.org/10.1016/j.foreco.2004.12.005, 2005.
Lasslop, G., Coppola, A. I., Voulgarakis, A., Yue, C., and Veraverbeke, S.: Influence of Fire on the Carbon Cycle and Climate, Curr. Clim. Change Rep., 5, 112–123, https://doi.org/10.1007/s40641-019-00128-9, 2019.
Law, K. S. and Stohl, A.: Arctic air pollution: Origins and impacts, Science, 315, 1537, https://doi.org/10.1126/science.1137695, 2007.
Lawrence, D. M. and Slater, A. G.: A projection of severe near-surface permafrost degradation during the 21st century, Geophys. Res. Lett, 32, L24401, https://doi.org/10.1029/2005GL025080, 2005.
Lehtonen, I., Venäläinen, A., Kämäräinen, M., Peltola, H., and Gregow, H.: Risk of large-scale fires in boreal forests of Finland under changing climate, Nat. Hazards Earth Syst. Sci., 16, 239–253, https://doi.org/10.5194/nhess-16-239-2016, 2016.
Lidskog, R., Johansson, J., and Sjödin, D.: Wildfires, responsibility and trust: public understanding of Sweden's largest wildfire, Scand. J. Forest Res., 34, 319, https://doi.org/10.1080/02827581.2019.1598483, 2019.
Lindberg, H., Punttila, P., and Vanha-Majamaa, I.: The challenge of combining variable retention and prescribed burning in Finland, Ecol. Proc., 9, 4, https://doi.org/10.1186/s13717-019-0207-3, 2020.
Little, J. M., Jandt, R. R., Drury, S., Molina, A., and Lane, B.: Evaluating the effectiveness of fuel treatments in Alaska-Final Report to the Joint Fire Science Program, JFSP Project No. 14-5-01-27, University of Alaska-Fairbanks, available at: https://www.fs.fed.us/psw/pubs/58856 (last access: 13 September 2021), 2018.
Liu, T.: Fire Inventories: Regional Evaluation, Comparison, and Metrics (FIRECAM) Tool, Earth Engine Apps [data], available at: https://globalfires.earthengine.app/view/firecam (last access: 13 September 2021), 2020.
Liu, T., Mickley, L. J., Marlier, M. E., DeFries, R. S., Khan, M. F., Latif, M. T., and Karambelas, A.: Diagnosing spatial biases and uncertainties in global fire emissions inventories: Indonesia as regional case study, Remote Sens. Environ., 237, 111557, https://doi.org/10.31223/osf.io/nh57j, 2020.
Loboda, T. V., Hall, J. V., Hall, A. H., and Shevade, V. S.: ABoVE: Cumulative Annual Burned Area, Circumpolar High Northern Latitudes, 2001–2015, available at: https://daac.ornl.gov/cgi-bin/dsviewer.pl?ds_id=1526 (last access: 13 September 2021), ORNL DAAC, Oak Ridge, TN, USA, https://doi.org/10.3334/ORNLDAAC/1526, 2017.
Loepfe, L., Lloret, F., and Román-Cuesta, R. M.: Comparison of burnt area estimates derived from satellite products and national statistics in Europe, Int. J. Remote Sens., 33, 3653, https://doi.org/10.1080/01431161.2011.631950, 2012.
Loisel, J., Gallego-Sala, A. V., Amesbury, M. J., Magnan, G., Anshari, G., Beilman, D. W., Benavides, J. C., Blewett, J., Camill, P., Charman, D. J., and Chawchai, S.: Expert assessment of future vulnerability of the global peatland carbon sink, Nat. Clim. Change, 11, 70–77, https://doi.org/10.1038/s41558-020-00944-0, 2021.
Loranty, M. M., Lieberman-Cribbin, W., Berner, L. T., Natali, S. M., Goetz, S. J., Alexander, H. D., and Kholodov, A. L.: Spatial variation in vegetation productivity trends, fire disturbance, and soil carbon across arctic-boreal permafrost ecosystems, Environ. Res. Lett., 11, 095008, https://doi.org/10.1088/1748-9326/11/9/095008, 2016.
Malevsky-Malevich, S. P., Molkentin, E. K., Nadyozhina, E. D., and Shklyarevich, O. B.: Numerical simulation of permafrost parameters distribution in Russia, Cold. Reg. Sci. Technol., 32, 1–11, https://doi.org/10.1016/s0165-232x(01)00018-0, 2001.
Markuse, P.: Before/After Comparison of the July/August 2019 Greenland Wildfire: Analysis from Sentinel-2, available at: https://pierre-markuse.net/2019/08/19/before-after-comparison-of-the-july-august-2019-greenland-wildfire/ (last access: 13 September 2021), 2019.
Masrur, A., Petrov, A. N., and DeGroote, J.: Circumpolar spatio-temporal patterns and contributing climatic factors of wildfire activity in the Arctic tundra from 2001–2015, Environ. Res. Lett., 13, 014019, https://doi.org/10.1088/1748-9326/aa9a76, 2018.
McCarty, J. L., Krylov, A., Prishchepov, A. V., Banach, D. M., Tyukavina, A., Potapov, P., and Turubanova, S.: Agricultural fires in European Russia, Belarus, and Lithuania and their impact on air quality, 2002–2012, In Land-Cover and Land-Use Changes in Eastern Europe after the Collapse of the Soviet Union in 1991, Springer, 2017.
McCarty, J. L., Smith, T. E., and Turetsky, M. R.: Arctic fires re-emerging, Nat. Geosci., 13, 658, https://doi.org/10.1038/s41561-020-00645-5, 2020.
McGwinn, K.: Hikers warned as Greenland wildfire burns out of control, Arctic Today, available at: https://www.arctictoday.com/hikers-warned-as-greenland-wildfire-burns-out-of-control/ (last access: 13 September 2021), 2019.
McWethy, D. B., Schoennagel, T., Higuera, P. E., Krawchuk, M., Harvey, B. J., Metcalf, E. C., Schultz, C., Miller, C., Metcalf, A.L., Buma, B. and Virapongse, A.: Rethinking resilience to wildfire, Nat. Sustain, 2, 797, https://doi.org/10.1038/s41893-019-0353-8, 2019.
Mekonnen, Z. A., Riley, W. J., Randerson, J. T., Grant, R. F., and Rogers. B. M.: Expansion of high-latitude deciduous forests driven by interactions between climate warming and fire, Nat. Plants, 5, 952, https://doi.org/10.1038/s41477-019-0495-8, 2019.
Melekhov, I. S.: Forest Science: “Forest Industry” Textbook, Moscow, 1980 (in Russian).
Michaelides, R. J., Schaefer, K., Zebker, H. A., Parsekian, A., Liu, L., Chen, J., Natali, S., Ludwig, S. and Schaefer, S.R.: Inference of the impact of wildfire on permafrost and active layer thickness in a discontinuous permafrost region using the remotely sensed active layer thickness (ReSALT) algorithm, Environ. Res. Lett., 14, 035007, https://doi.org/10.1088/1748-9326/aaf932, 2019.
Mieville, A., Granier, C., Liousse, C., Guillaume, B., Mouillot, F., Lamarque, J. F., Grégoire, J. M., and Pétron, G.: Emissions of gases and particles from biomass burning during the 20th century using satellite data and an historical reconstruction, Atmos. Environ., 44, 1469, https://doi.org/10.1016/j.atmosenv.2010.01.011, 2010.
Miller, M. E., Billmire, M., Bourgeau-Chavez, L., Elliot, W. J., Robichaud, P. R., and MacDonald, L.: Rapid response tools and datasets for post-fire modeling in Boreal and Arctic Environments, Spring 2017 AFSC Remote Sensing Workshop: Opportunities to Apply Remote Sensing in Boreal/Arctic Wildfire Management and Science, available at: https://digitalcommons.mtu.edu/mtri_p/290 (last access: 13 September 2021), 2017.
Mölders, N. and Kramm, G.: Climatology of Air Quality in Arctic Cities – Inventory and Assessment, Open J. Air Pollut., 7, 48–93, https://doi.org/10.4236/ojap.2018.71004, 2018.
Molinari, C., Lehsten, V., Blarquez, O., Carcaillet, C., Davis, B. A., Kaplan, J. O., Clear, J., and Bradshaw, R. H.: The climate, the fuel and the land use: Long-term regional variability of biomass burning in boreal forests, Glob. Change Biol., 24, 4929–4945, https://doi.org/10.1111/gcb.14380, 2018.
Monks, S. A., Arnold, S. R., and Chipperfield, M. P.: Evidence for El Niño-Southern Oscillation (ENSO) influence on Arctic CO interannual variability through biomass burning emissions, Geophys. Res. Lett., 39, L14804, https://doi.org/10.1029/2012GL052512, 2012.
Montesano, P. M., Neigh, C. S., Macander, M., Feng, M., and Noojipady, P.: The bioclimatic extent and pattern of the cold edge of the boreal forest: the circumpolar taiga-tundra ecotone, Environ. Res. Lett., 15, 105019, https://doi.org/10.1088/1748-9326/abb2c7, 2020.
Morin, P., Porter, C., Cloutier, M., Howat, I., Noh, M.J., Willis, M., Bates, B., Willamson, C., and Peterman, K.: ArcticDEM: a publicly available, high resolution elevation model of the Arctic, available at: https://livingatlas2.arcgis.com/arcticdemexplorer/ (last access: 13 September 2021), 2016.
Mottershead, K. D., McGee, T. K., and Christianson, A.: Evacuating a First Nation Due to Wildfire Smoke: The Case of Dene Tha' First Nation, Int. J. Disaster Risk. Sci., 11, 274, https://doi.org/10.1007/s13753-020-00281-y, 2020.
NIFC: National Interagency Fire Center: Total Wildland Fires and Acres (1926–2019), available at: https://www.nifc.gov/fireInfo/fireInfo_stats_totalFires.html (last access: 13 September 2021), 2019.
Nikolakis, W., Roberts, E., Hotte, N. and Ross, R.M.: Goal setting and Indigenous fire management: a holistic perspective, Int. J. Wildland Fire, 29, 974, https://doi.org/10.1071/WF20007, 2020.
Nitzbon, J., Westermann, S., Langer, M., Martin, L. C., Strauss, J., Laboor, S., and Boike, J.: Fast response of cold ice-rich permafrost in northeast Siberia to a warming climate, Nat. Commun., 11, 2201, https://doi.org/10.1038/s41467-020-15725-8, 2020.
Nordregio.: Indigenous population in the Arctic, available at: https://nordregio.org/maps/indigenous-population-in-the-arctic/ (last access: 13 September 2021), 2019.
Nugent, K. A., Strachan, I. B., Roulet, N. T., Strack, M., Frolking, S., and Helbig, M.: Prompt active restoration of peatlands substantially reduces climate impact, Environ. Res. Lett., 14, 124030, https://doi.org/10.1088/1748-9326/ab56e6, 2019.
Oliva, P. and Schroeder, W.: Assessment of VIIRS 375 m active fire detection product for direct burned area mapping, Remote Sens. Environ., 160, 144, https://doi.org/10.1016/j.rse.2015.01.010, 2015.
O'Neill, H. B., Burn, C. R., Allard, M., Arenson, L. U., Bunn, M. I., Connon, R. F., Kokelj, S. A., Kokelj, S. V., LeBlanc, A.-M., Morse, P. D., and Smith, S. L.: Permafrost thaw and northern development, Nat. Clim. Chang., 10, 722–723, https://doi.org/10.1038/s41558-020-0862-5, 2020.
Päätalo, M.-L.: Factors influencing occurrence and impacts of fires in northern European forests, Silva Fenn., 32, 185–202, https://doi.org/10.14214/sf.695, 1998.
Pan, X., Ichoku, C., Chin, M., Bian, H., Darmenov, A., Colarco, P., Ellison, L., Kucsera, T., da Silva, A., Wang, J., Oda, T., and Cui, G.: Six global biomass burning emission datasets: intercomparison and application in one global aerosol model, Atmos. Chem. Phys., 20, 969–994, https://doi.org/10.5194/acp-20-969-2020, 2020.
Parfenova, E., Tchebakova, N., and Soja, A.: Assessing landscape potential for human sustainability and `attractiveness' across Asian Russia in a warmer 21st century, Environ. Res. Lett., 14, 065004, https://doi.org/10.1088/1748-9326/ab10a8, 2019.
Parisien, M. A., Miller, C., Parks, S. A., DeLancey, E. R., Robinne, F. N., and Flannigan, M. D.: The spatially varying influence of humans on fire probability in North America, Environ. Res. Lett., 11, 075005, https://doi.org/10.1088/1748-9326/11/7/075005, 2016.
Parisien, M. A., Barber, Q. E., Hirsch, K. G., Stockdale, C. A., Erni, S., Wang, X., Arseneault, D., and Parks, S. A.: Fire deficit increases wildfire risk for many communities in the Canadian boreal forest, Nat. Commun., 11, 2121, https://doi.org/10.1038/s41467-020-15961-y, 2020.
Partain Jr., J. L., Alden, S., Strader, H., Bhatt, U. S., Bieniek, P. A., Brettschneider, B. R., Walsh, J. E., Lader, R. T., Olsson, P. Q., Rupp, T. S., and Thoman Jr., R. L.:. An assessment of the role of anthropogenic climate change in the Alaska fire season of 2015 [in “Explaining Extremes of 2015 from a Climate Perspective”], B. Am. Meteorol. Soc., 97, S14–S18, https://doi.org/10.1175/bams-d-16-0149.1, 2016.
Peltola, H., Kilpeläinen, A., and Kellomäki, S.: Diameter growth of Scots pine (Pinus sylvestris) trees grown at elevated temperature and carbon dioxide concentration under boreal conditions, Tree Physiol., 22, 963–972, https://doi.org/10.1093/treephys/22.14.963, 2002.
Pickell, P. D., Coops, N. C., Ferster, C. J., Bater, C. W., Blouin, K. D., Flannigan, M. D., and Zhang, J.: An early warning system to forecast the close of the spring burning window from satellite-observed greenness, Sci. Rep., 7, 1–10, https://doi.org/10.1038/s41598-017-14730-0, 2017.
Pimentel, R. and Arheimer, B.: Hydrological impacts of a wildfire in a Boreal region: The Västmanland fire 2014 (Sweden), Sci. Total Environ. 756, 143519, https://doi.org/10.1016/j.scitotenv.2020.143519, 2021.
Polikarpov, N. P., Andreeva, N. M., Nazimova, D. I., Sirotinina, A. V., and Sofronov, M. A.: Formation composition of the forest zones in Siberia as a reflection of forest-forming tree species interrelations, Russ. J. Forest Sci., 5, 3–11, 1998.
Prat-Guitart, N., Rein, G., Hadden, R. M., Belcher, C. M., and Yearsley, J. M.: Propagation probability and spread rates of self-sustained smouldering fires under controlled moisture content and bulk density conditions, Int. J. Wildland Fire, 25, 456–465, https://doi.org/10.1071/WF15103, 2016.
Prishchepov, A. V., Schierhorn, F., Dronin, N., Ponkina, E. V., and Müller, D.: 800 Years of Agricultural Land-use Change in Asian (Eastern) Russia, in: KULUNDA: Climate Smart Agriculture, Innovations in Landscape Research, edited by: Frühauf, M., Guggenberger, G., Meinel, T., Theesfeld, I., Lentz, S., Springer, Cham, Switzerland, 67–87, https://doi.org/10.1007/978-3-030-15927-6_6, 2020.
Púčik, T., Groenemeijer, P., Rädler, A. T., Tijssen, L., Nikulin, G., Prein, A. F., van Meijgaard, E., Fealy, R., Jacob, D., and Teichmann, C.: Future changes in European severe convection environments in a regional climate model ensemble, J. Clim., 30, 6771, https://doi.org/10.1175/jcli-d-16-0777.1, 2017.
Pureswaran, D. S., Roques, A., and Battisti, A.: Forest insects and climate change, Curr. Forest Rep., 4, 35–50, https://doi.org/10.1007/s40725-018-0075-6, 2018.
Qi, L. and Wang, S.: Sources of black carbon in the atmosphere and in snow in the Arctic, Sci. Total Environ., 691, 442–454, https://doi.org/10.1016/j.scitotenv.2019.07.073, 2019.
Raynolds, M. K., Walker, D. A., Balser, A., Bay, C., Campbell, M., Cherosov, M. M., Daniëls, F. J. A., Eidesen, P. B., Ermokhina, K. A., Frost, G. V., Jedrzejek, B., Jorgenson, M. T., Kennedy, B. E., Kholod, S. S., Lavrinenko, I. A., Lavrinenko, O. V., Magnússon, B., Matveyeva, N. V., Metúsalemsson, S., Nilsen, L., Olthof, I., Pospelov, I. N., Pospelova, E. B., Pouliot, D., Razzhivin, V., Schaepman-Strub, G., Šibík, J., Telyatnikov, M. Y., and Troeva, E.: A raster version of the Circumpolar Arctic Vegetation Map (CAVM), Remote Sens. Environ., 232, 111297, https://doi.org/10.1016/j.rse.2019.111297, 2019.
Rein, G.: Smoldering combustion, in: SFPE Handbook of Fire Protection Engineering, edited by: Hurley, M. J., Gottuk, D., Hall, J. R., Harada, K., Kuligowski, E., Puchovsky, M., Torero, J., Watts, J. M., and Wieczoreks, C., Springer New York, New York, New York, 581–603, https://doi.org/10.1007/978-1-4939-2565-0_19, 2016.
Rein, G., Cleaver, N., Ashton, C., Pironi, P., and Torero, J. L.: The severity of smouldering peat fires and damage to the forest soil, Catena, 74, 304–309, https://doi.org/10.1016/j.catena.2008.05.008, 2008.
Rémy, S., Veira, A., Paugam, R., Sofiev, M., Kaiser, J. W., Marenco, F., Burton, S. P., Benedetti, A., Engelen, R. J., Ferrare, R., and Hair, J. W.: Two global data sets of daily fire emission injection heights since 2003, Atmos. Chem. Phys., 17, 2921–2942, https://doi.org/10.5194/acp-17-2921-2017, 2017.
Riley, K. L., Williams, A. P., Urbanski, S. P., Calkin, D. E., Short, K. C., and O'Connor, C. D.: Will Landscape Fire Increase in the Future? A Systems Approach to Climate, Fire, Fuel, and Human Drivers, Curr. Pollut. Rep., 5, 9–24, https://doi.org/10.1007/s40726-019-0103-6, 2019.
Robinne, F. N., Parisien, M. A., and Flannigan, M.: Anthropogenic influence on wildfire activity in Alberta, Canada, Int. J. Wildland Fire, 25, 1131–1143, https://doi.org/10.1071/wf16058, 2016.
Robinne, F. N., Hallema, D. W., Bladon, K. D., and Buttle, J. M: Wildfire impacts on hydrologic ecosystem services in North American high-latitude forests: A scoping review, J. Hydrol., 581, 124360, https://doi.org/10.1016/j.jhydrol.2019.124360, 2020.
Rocha, A. V., Loranty, M. M., Higuera, P. E., Mack, M. C., Hu, F. S., Jones, B. M., Breen, A. L., Rastetter, E. B., Goetz, S. J., and Shaver, G. R.: The footprint of Alaskan tundra fires during the past half-century: implications for surface properties and radiative forcing, Environ. Res. Lett., 7, 044039, https://doi.org/10.1088/1748-9326/7/4/044039, 2012.
Rogers, B. M., Veraverbeke, S., Azzari, G., Czimczik, C. I., Holden, S. R., Mouteva, G. O., Sedano, F., Treseder, K. K., and Randerson, J. T.: Quantifying fire-wide carbon emissions in interior Alaska using field measurements and Landsat imagery, J. Geophys. Res.-Biogeo., 119, 1608, https://doi.org/10.1002/2014JG002657, 2014.
Rogers, B. M., Soja, A. J., Goulden, M. L., and Randerson, J. T.: Influence of tree species on continental differences in boreal fires and climate feedbacks, Nat. Geosci., 8, 228, https://doi.org/10.1038/ngeo2352, 2015.
Rogers, B. M., Balch, J. K., Goetz, S. J., Lehmann, C. E., and Turetsky, M.: Focus on changing fire regimes: interactions with climate, ecosystems, and society, Environ. Res. Lett., 15, 030201, https://doi.org/10.1088/1748-9326/ab6d3a, 2020.
Ronkainen, T., Väliranta, M., and Tuittila, E.-S.: Fire pattern in a drainage-affected boreal bog, Boreal Environ. Res., 18, 309–316, 2013.
Santoso, M. A., Cui, W., Amin, H. M., Christensen, E. G., Nugroho, Y. S., and Rein, G.: Laboratory study on the suppression of smouldering peat wildfires: effects of flow rate and wetting agent, Int. J. Wildland Fire, 30, 378–390, https://doi.org/10.1071/WF20117, 2021.
Schmale, J., Arnold, S. R., Law, K. S., Thorp, T., Anenberg, S., Simpson, W. R., Mao, J., and Pratt, K. A.: Local Arctic air pollution: A neglected but serious problem, Earth's Future, 6, 1385, https://doi.org/10.1029/2018EF000952, 2018.
Scholten, R. and Veraverbeke, S.: Alaska Fire Science Consortium: Spatiotemporal patterns of overwintering fire in Alaska, available at: https://akfireconsortium.files.wordpress.com/2020/03/fsh_2020mar25_holdoverfires-1.pdf (last access: 13 September 2021), 2020.
Seidl, R., Schelhaas, M. J., Rammer, W., and Verkerk, P. J.: Increasing forest disturbances in Europe and their impact on carbon storage, Nat. Clim. Change, 4, 806–810, https://doi.org/10.1038/nclimate2318, 2014.
Sherstyukov, B. G. and Sherstyukov, A. B.: Assessment of increase in forest fire risk in Russia till the late 21st century based on scenario experiments with fifth-generation climate models, Russ. Meteorol. Hydro+, 39, 292, https://doi.org/10.3103/s1068373914050021, 2014.
Shiwakoti, S., Zheljazkov, V. D., Gollany, H. T., Kleber, M., Xing, B., and Astatkie, T.:. Micronutrients in the Soil and Wheat: Impact of 84 Years of Organic or Synthetic Fertilization and Crop Residue Management, Agronomy, 9, 464, https://doi.org/10.3390/agronomy9080464, 2019.
Shugart, H. H., Leemans, R., and Bonan, G. B.: A Systems Analysis of the Global Boreal Forest, Cambridge University Press, New York, USA, 1–565, https://doi.org/10.1017/CBO9780511565489.022, 1992.
Shuman, J. K., Foster, A. C., Shugart, H. H., Hoffman-Hall, A., Krylov, A., Loboda, T., Ershov, D., and Sochilova, E.: Fire disturbance and climate change: implications for Russian forests, Environ. Res. Lett., 12, 035003, https://doi.org/10.1088/1748-9326/aa5eed, 2017.
Shumilova, L. V.: Botanical Geography of Siberia, Tomsk University Press, Tomsk, USSR, 1962.
Shvidenko, A. Z. and Nilsson, S.: Extent, distribution, and ecological role of fire in Russian forests, in: Fire, climate change, and carbon cycling in the boreal forest, edited by: Kasischke E. S. and Stocks B. J., Springer, New York, NY, 132–150, https://doi.org/10.1007/978-0-387-21629-4_16, 2000.
Sidorova, E. J.: The incorporation of Traditional Ecological Knowledge in the Arctic Council: Lip service?, Polar Rec., 56, 1–12, https://doi.org/10.1017/S0032247420000273, 2020.
Silva, J. S. and Harrison, S. P.: Humans, Climate and Land Cover as Controls on European Fire Regimes, in: Towards integrated fire management-Outcomes of the European Project Fire Paradox, edited by: Silva, J. S., Rego, F. C., Fernandes, P., and Rigolot, E., European Forest Institute, Joensuu, Finald, 49–59, 2010.
Sirin, A., Maslov, A., Medvedeva, M., Vozbrannaya, A., Valyaeva, N., Tsyganova, O., Glukhova, T., and Makarov, D.: Multispectral Remote Sensing Data as a Tool for Assessing the Need and the Effectiveness for Peatland Restoration, In Proceedings of the 9th European Conference on Ecological Restoration, edited by: Tolvanen, A. and Hekkala, A. M., Finnish Forest Research Institute, Oulu, Finland, p. 133, 2014.
Sirin, A., Medvedeva, M., Maslov, A., and Vozbrannaya, A.: Assessing the Land and Vegetation Cover of Abandoned Fire Hazardous and Rewetted Peatlands: Comparing Different Multispectral Satellite Data, Land, 7, 71, https://doi.org/10.3390/land7020071, 2018.
Sizov, O., Ezhova, E., Tsymbarovich, P., Soromotin, A., Prihod'ko, N., Petäjä, T., Zilitinkevich, S., Kulmala, M., Bäck, J., and Köster, K.: Fire and vegetation dynamics in northwest Siberia during the last 60 years based on high-resolution remote sensing, Biogeosciences, 18, 207–228, https://doi.org/10.5194/bg-18-207-2021, 2021.
Sjöström, J., Plathner, F. V., and Granström, A.: Wildfire ignition from forestry machines in boreal Sweden, Int. J. Wildland Fire, 28, 666, https://doi.org/10.1071/wf18229, 2019.
Smirnov, N. S., Korotkov, V. N., and Romanovskaya, A. A.: Black carbon emissions from wildfires on forest lands of the Russian Federation in 2007–2012, Russ. Meteorol. Hydro+, 40, 435, https://doi.org/10.3103/s1068373915070018, 2015.
Sofronov, M. A. and Volokitina A. V.: Wildfire Ecology in Continuous Permafrost Zone, in: Permafrost Ecosystems, Ecological Studies (Analysis and Synthesis), Vol. 209, edited by: Osawa, A., Zyryanova, O., Matsuura, Y., Kajimoto, T., and Wein, R., Springer, Dordrecht, the Netherlands, 59–82, https://doi.org/10.1007/978-1-4020-9693-8_4, 2010.
Sofronov, M. A., Volokitina, A. V., and Shvidenko, A. Z.: Wildland fires in the north of Central Siberia, Commonw. For. Rev., 77, 124–127, 1998.
Sofronov, M. A., Volokitina, A., Kajimoto, T., Matsuura, Y., and Uemura, S.: Zonal peculiarities of forest vegetation controlled by fires in northern Siberia, Euras. J. Forest Res., 1, 51–57, 2000.
Soja, A. J., Cofer, W. R., Shugart, H. H., Sukhinin, A. I., Stackhouse, P. W., McRae, D. J., and Conard, S. G.: Estimating fire emissions and disparities in boreal Siberia (1998–2002), J. Geophys. Res.-Atmos., 109, D14S06, https://doi.org/10.1029/2004JD004570, 2004a.
Soja, A. J., Sukhinin, A. I., Cahoon Jr., D. R., Shugart, H. H., and Stackhouse Jr., P. W.: AVHRR-derived fire frequency, distribution and area burned in Siberia, Int. J. Remote Sens., 25, 1939, https://https://doi.org/10.1080/01431160310001609725, 2004b.
Soja, A. J., Shugart, H. H., Sukhinin, A., Conard, S., and Stackhouse Jr., P. W.: Satellite-Derived Mean Fire Return Intervals As Indicators Of Change In Siberia (1995–2002), Mitig. Adapt. Strat. Glob. Change, 11, 75–96, https://doi.org/10.1007/s11027-006-1009-3, 2006.
Sommers, W. T., Loehman, R. A., and Hardy, C. C.: Wildland fire emissions, carbon, and climate: Science overview and knowledge needs, Forest Ecol. Manag., 317, 1–8, https://doi.org/10.1016/j.foreco.2013.12.014, 2014.
Stralberg, D., Wang, X., Parisien, M. A., Robinne, F. N., Sólymos, P., Mahon, C. L., Nielsen, S. E., and Bayne, E. M.: Wildfire-mediated vegetation change in boreal forests of Alberta, Canada, Ecosphere, 9, e02156, https://doi.org/10.1002/ecs2.2156, 2018.
Stone, R.: As the Arctic thaws, Indigenous Alaskans demand a voice in climate change research, Sci. Mag., https://doi.org/10.1126/science.abe7149, 2020.
Stroeve, J. C., Markus, T., Boisvert, L., Miller, J., and Barrett, A.: Changes in Arctic melt season and implications for sea ice loss, Geophys. Res. Lett., 41, 1216–1225, https://doi.org/10.1002/2013GL058951, 2014.
Tchebakova, N. M., Parfenova, E., and Soja, A. J.: The effects of climate, permafrost and fire on vegetation change in Siberia in a changing climate, Environ. Res. Lett., 4, 045013, https://doi.org/10.1088/1748-9326/4/4/045013, 2009.
Tchebakova, N. M., Rehfeldt, G. E., and Parfenova, E.: From Vegetation Zones to Climatypes: Effects of Climate Warming on Siberian Ecosystems, in: Permafrost Ecosystems, Ecological Studies (Analysis and Synthesis), edited by: Osawa, A., Zyryanova, O., Matsuura, Y., Kajimoto, T., and Wein, R., Springer, Dordrecht, Germany, 427–446, https://doi.org/10.1007/978-1-4020-9693-8_22, 2010.
Tchebakova, N. M., Parfenova, E. I., Lysanova, G. I., and Soja, A. J.: Agroclimatic potential across central Siberia in an altered twenty-first century, Environ. Res. Lett., 6, 045207, https://doi.org/10.1088/1748-9326/6/4/045207, 2011.
Tchebakova, N. M., Chuprova, V. V., Parfenova, E. I., Soja, A. J., and Lysanova, G. I.: Evaluating the agroclimatic potential of Central Siberia, in: Novel Methods for Monitoring and Managing Land and Water Resources in Siberia, edited by: Mueller, L., Sheudshen, A., and Eulenstein, F., Springer, Cham, https://doi.org/10.1007/978-3-319-24409-9_10, 2016.
Terrier, A., Girardin, M. P., Périé, C., Legendre, P., and Bergeron, Y.: Potential changes in forest composition could reduce impacts of climate change on boreal wildfires, Ecol. Appl., 23, 21–35, https://doi.org/10.1890/12-0425.1, 2013.
Teufel, B. and Sushama, L.: Abrupt changes across the Arctic permafrost region endanger northern development, Nat. Clim. Change, 9, 858, https://doi.org/10.1038/s41558-019-0614-6, 2019.
Theesfeld, I. and Jelinek, L.: A misfit in policy to protect Russia's black soil region, An institutional analytical lens applied to the ban on burning of crop residues, Land Use Policy, 67, 517, https://doi.org/10.1016/j.landusepol.2017.06.018, 2017.
Thomas, J. L., Polashenski, C. M., Soja, A. J., Marelle, L., Casey, K. A., Choi, H. D., Raut, J. C., Wiedinmyer, C., Emmons, L. K., Fast, J. D., and Pelon, J.: Quantifying black carbon deposition over the Greenland ice sheet from forest fires in Canada, Geophys. Res. Lett., 44, 7965, https://doi.org/10.1002/2017gl073701, 2017.
Thompson, D. K. and Morrison, K.: A classification scheme to determine wildfires from the satellite record in the cool grasslands of southern Canada: considerations for fire occurrence modelling and warning criteria, Nat. Hazards Earth Syst. Sci., 20, 3439–3454, https://doi.org/10.5194/nhess-20-3439-2020, 2020.
Thompson, D. K., Simpson, B. N., Whitman, E., Barber, Q. E., and Parisien, M. A.: Peatland hydrological dynamics as a driver of landscape connectivity and fire activity in the boreal plain of Canada, Forests, 10, 534, https://doi.org/10.3390/f10070534, 2019.
Turetsky, M., Benscoter, B., Page, S., Rein, G., Van Der Werf, G. R., and Watts, A.: Global vulnerability of peatlands to fire and carbon loss, Nat. Geosci., 8, 11–14, https://doi.org/10.1038/ngeo2325, 2015.
Tymstra, C., Stocks, B. J., Cai, X., and Flannigan, M. D.: Wildfire management in Canada: Review, challenges and opportunities, Prog. Disaster Scie., 5, 100045, https://doi.org/10.1016/j.pdisas.2019.100045, 2020.
van der Werf, G. R., Randerson, J. T., Giglio, L., Collatz, G. J., Kasibhatla, P. S., and Arellano Jr., A. F.: Interannual variability in global biomass burning emissions from 1997 to 2004, Atmos. Chem. Phys., 6, 3423–3441, https://doi.org/10.5194/acp-6-3423-2006, 2006.
van der Werf, G. R., Randerson, J. T., Giglio, L., Collatz, G. J., Mu, M., Kasibhatla, P. S., Morton, D. C., DeFries, R. S., Jin, Y., and van Leeuwen, T. T.: Global fire emissions and the contribution of deforestation, savanna, forest, agricultural, and peat fires (1997–2009), Atmos. Chem. Phys., 10, 11707–11735, https://doi.org/10.5194/acp-10-11707-2010, 2010.
van der Werf, G. R., Randerson, J. T., Giglio, L., van Leeuwen, T. T., Chen, Y., Rogers, B. M., Mu, M., van Marle, M. J. E., Morton, D. C., Collatz, G. J., Yokelson, R. J., and Kasibhatla, P. S.: Global fire emissions estimates during 1997–2016, Earth Syst. Sci. Data, 9, 697–720, https://doi.org/10.5194/essd-9-697-2017, 2017.
Van Leeuwen, T. T., van der Werf, G. R., Hoffmann, A. A., Detmers, R. G., Rücker, G., French, N. H. F., Archibald, S., Carvalho Jr., J. A., Cook, G. D., de Groot, W. J., Hély, C., Kasischke, E. S., Kloster, S., McCarty, J. L., Pettinari, M. L., Savadogo, P., Alvarado, E. C., Boschetti, L., Manuri, S., Meyer, C. P., Siegert, F., Trollope, L. A., and Trollope, W. S. W.: Biomass burning fuel consumption rates: a field measurement database, Biogeosciences, 11, 7305–7329, https://doi.org/10.5194/bg-11-7305-2014, 2014.
Veira, A., Lasslop, G., and Kloster, S.: Wildfires in a warmer climate: emission fluxes, emission heights, and black carbon concentrations in 2090–2099, J. Geophys. Res.-Atmos., 121, 3195, https://doi.org/10.1002/2015jd024142, 2016.
Venäläinen, A., Lehtonen, I., Laapas, M., Ruosteenoja, K., Tikkanen, O. P., Viiri, H., Ikonen, V. P., and Peltola, H.: Climate change induces multiple risks to boreal forests and forestry in Finland: A literature review, Glob Change Biol., 26, 4178, https://doi.org/10.1111/gcb.15183, 2020.
Veraverbeke, S., Rogers, B. M., Goulden, M. L., Jandt, R. R., Miller, C. E., Wiggins, E. B., and Randerson, J. T.: Lightning as a major driver of recent large fire years in North American boreal forests, Nat. Clim. Change, 7, 529, https://doi.org/10.1038/nclimate3329, 2017.
Viatte, C., Strong, K., Hannigan, J., Nussbaumer, E., Emmons, L. K., Conway, S., Paton-Walsh, C., Hartley, J., Benmergui, J., and Lin, J.: Identifying fire plumes in the Arctic with tropospheric FTIR measurements and transport models, Atmos. Chem. Phys., 15, 2227–2246, https://doi.org/10.5194/acp-15-2227-2015, 2015.
Voulgarakis, A., Marlier, M. E., Faluvegi, G., Shindell, D. T., Tsigaridis, K., and Mangeon, S.: Interannual variability of tropospheric trace gases and aerosols: the role of biomass burning emissions, J. Geophys. Res.-Atmos., 120, 7157–7173, https://doi.org/10.1002/2014JD022926, 2015.
Waigl, C. F., Stuefer, M., Prakash, A., and Ichoku, C.: Detecting high and low-intensity fires in Alaska using VIIRS I-band data: An improved operational approach for high latitudes, Remote Sens. Environ., 199, 389, https://doi.org/10.1016/j.rse.2017.07.003, 2017.
Waigl, C. F., Prakash, A., Stuefer, M., Verbyla, D., and Dennison, P.: Fire detection and temperature retrieval using EO-1 Hyperion data over selected Alaskan boreal forest fires, Int. J. Appl. Earth Obs., 81, 72–84, https://doi.org/10.1016/j.jag.2019.03.004, 2019.
Walker, X. J., Baltzer, J. L., Cumming, S. G., Day, N. J., Ebert, C., Goetz, S., Johnstone, J. F., Potter, S., Rogers, B. M., Schuur, E. A., and Turetsky, M. R.: Increasing wildfires threaten historic carbon sink of boreal forest soils, Nature, 572, 520–523, https://doi.org/10.1038/s41586-019-1474-y, 2019.
Walker, X. J., Rogers, B. M., Veraverbeke, S., Johnstone, J. F., Baltzer, J. L., Barrett, K., Bourgeau-Chavez, L., Day, N. J., de Groot, W. J., Dieleman, C. M. and Goetz, S.: Fuel availability not fire weather controls boreal wildfire severity and carbon emissions, Nat. Clim. Change, 10, 1130, https://doi.org/10.1038/s41558-020-00920-8, 2020.
Walsh, J. E., Ballinger, T. J., Euskirchen, E. S., Hanna, E., Mård, J., Overland, J. E., Tangen, H., and Vihma, T.: Extreme weather and climate events in northern areas: A review, Earth-Sci. Rev., 209, 103324, https://doi.org/10.1016/j.earscirev.2020.103324, 2020.
Wang, X., Parisien, M. A., Taylor, S. W., Candau, J. N., Stralberg, D., Marshall, G. A., Little, J. M., and Flannigan, M. D.: Projected changes in daily fire spread across Canada over the next century, Environ. Res. Lett., 12, 025005, https://doi.org/10.1088/1748-9326/aa5835, 2017.
Wang, J. A., Sulla-Menashe, D., Woodcock, C. E., Sonnentag, O., Keeling, R. F., and Friedl, M. A.: Extensive land cover change across Arctic–Boreal Northwestern North America from disturbance and climate forcing, Glob. Change Biol., 26, 807, https://doi.org/10.1111/gcb.14804, 2019.
Watson, J. G., Cao, J., Chen, L.-W. A., Wang, Q., Tian, J., Wang, X., Gronstal, S., Ho, S. S. H., Watts, A. C., and Chow, J. C.: Gaseous, PM2.5 mass, and speciated emission factors from laboratory chamber peat combustion, Atmos. Chem. Phys., 19, 14173–14193, https://doi.org/10.5194/acp-19-14173-2019, 2019.
Wein, R. W.: Frequency and characteristics of arctic tundra fires, Arctic, 29, 213, https://doi.org/10.14430/arctic2806, 1976.
Whitman, E., Parisien, M. A., Thompson, D. K., and Flannigan, M. D.: Short-interval wildfire and drought overwhelm boreal forest resilience, Sci. Rep., 9, 18796, https://doi.org/10.1038/s41598-019-55036-7, 2019.
Wiedinmyer, C., Akagi, S. K., Yokelson, R. J., Emmons, L. K., Al-Saadi, J. A., Orlando, J. J., and Soja, A. J.: The Fire INventory from NCAR (FINN): a high resolution global model to estimate the emissions from open burning, Geosci. Model Dev., 4, 625–641, https://doi.org/10.5194/gmd-4-625-2011, 2011.
Wilkinson, S. L., Moore, P. A., Thompson, D. K., Wotton, B. M., Hvenegaard, S., Schroeder, D., and Waddington, J. M.: The effects of black spruce fuel management on surface fuel condition and peat burn severity in an experimental fire, Can. J. For. Res., 48, 1433, https://doi.org/10.1139/cjfr-2018-0217, 2018.
Wilkinson, S. L., Moore, P. A., and Waddington, J. M.: Assessing Drivers of Cross-Scale Variability in Peat Smoldering Combustion Vulnerability in Forested Boreal Peatlands, Front. Forest Glob. Change, 2, 1–11, https://doi.org/10.3389/ffgc.2019.00084, 2019.
Wilson, G. N.: Indigenous Internationalism in the Arctic, in: The Palgrave Handbook of Arctic Policy and Politics, edited by: Coates, K.S., and Holroyd, C., Palgrave Macmillan, Cham, Switzerland, 27–40, https://doi.org/10.1007/978-3-030-20557-7_3, 2020.
Witze, A.: The Arctic is burning like never before-and that's bad news for climate change, Nature, 585, 336–337, https://doi.org/10.1038/d41586-020-02568-y, 2020.
Wotton, B. M., Martell, D. L., and Logan, K. A.: Climate change and people-caused forest fire occurrence in Ontario, Climatic Change, 60, 275, https://doi.org/10.1023/A:1026075919710, 2003.
Wotton, B. M., Flannigan, M. D., and Marshall, G. A.: Potential climate change impacts on fire intensity and key wildfire suppression thresholds in Canada, Environ. Res. Lett., 12, 095003, https://doi.org/10.1088/1748-9326/aa7e6e, 2017.
Wrona, F. J., Johansson, M., Culp, J. M., Jenkins, A., Mård, J., Myers‐Smith, I. H., Prowse, T. D., Vincent, W. F., and Wookey, P. A.: Transitions in Arctic ecosystems: Ecological implications of a changing hydrological regime, J. Geophys. Res.-Biogeo., 121, 650–675, https://doi.org/10.1002/2015JG003133, 2016.
Xu, J., Morris, P. J., Liu, J., and Holden, J.: PEATMAP: Refining estimates of global peatland distribution based on a meta-analysis, Catena, 160, 134–140, https://doi.org/10.1016/j.catena.2017.09.010, 2018.
Xu, W., He, H. S., Hawbaker, T. J., Zhu, Z., and Henne, P. D.: Estimating burn severity and carbon emissions from a historic megafire in boreal forests of China, Sci. Total Environ., 716, 136534, https://doi.org/10.1016/j.scitotenv.2020.136534, 2020.
Yanagiya, K. and Furuya, M.: Post-wildfire surface deformation near Batagay, Eastern Siberia, detected by L-band and C-band InSAR, J. Geophys. Res., 125, e2019JF005473, https://doi.org/10.1029/2019JF005473, 2020.
York, A., Bhatt, U. S., Gargulinski, E., Garbinski, Z., Jain, P., Soja, A., Thoman, R. L., and Ziel, R: Wildland Fire in High Northern Latitudes, in: Arctic Report Card 2020, edited by: Thoman, R. L., Richter-Menge, J., and Druckenmiller, M. L., https://doi.org/10.25923/2gef-3964, 2020.
Young, A. M., Higuera, P. E., Duffy, P. A., and Hu, F. S.: Climatic thresholds shape northern high-latitude fire regimes and imply vulnerability to future climate change, Ecogeg, 40, 606, https://doi.org/10.1111/ecog.02205, 2016.
Young, T. K. and Bjerregaard, P.: Towards estimating the indigenous population in circumpolar regions, Int. J. Circumpolar Health, 78, 1653749, https://doi.org/10.1080/22423982.2019.1653749, 2019.
Yu, Z., Loisel, J., Brosseau, D. P., Beilman, D. W., and Hunt, S. J.: Global peatland dynamics since the Last Glacial Maximum, Geophys. Res. Lett., 37, L13402, https://doi.org/10.1029/2010gl043584, 2010.
Yuan, H., Richter, F., and Rein, G.: A multi-step reaction scheme to simulate self-heating ignition of coal: Effects of oxygen adsorption and smouldering combustion, Proc. Combust. Inst., 38, 4717–4725, https://doi.org/10.1016/j.proci.2020.07.016, 2021.
Zhang, R., Huang, C., Zhan, X., Jin, H., and Song, X. P.: Development of S-NPP VIIRS global surface type classification map using support vector machines, Int. J. Digit. Earth, 11, 212, https://doi.org/10.1080/17538947.2017.1315462, 2018.
Zhu, C., Kobayashi, H., Kanaya, Y., and Saito, M.: Size-dependent validation of MODIS MCD64A1 burned area over six vegetation types in boreal Eurasia: Large underestimation in croplands, Sci. Rep., 7, 4181, https://doi.org/10.1038/s41598-017-03739-0, 2017.
The Arctic Council membership comprises the eight member states: Canada, the Kingdom of Denmark, Finland, Iceland, Norway, the Russian Federation, Sweden, and the United States of America, as well as six Permanent Participants representing Arctic Indigenous peoples, including the Aleut International Association, the Arctic Athabaskan Council, the Gwich'in Council International, the Inuit Circumpolar Council, the Russian Association of Indigenous Peoples of the North, and the Saami Council.
- Abstract
- Introduction
- Drivers of Arctic fire regimes
- Future Arctic fire activity
- Arctic fire emissions
- Relevance of fire sources in global and Arctic emissions
- Fire management in the Arctic
- Knowledge gaps and associated uncertainties
- People and future Arctic fire regimes
- Conclusions
- Code and data availability
- Author contributions
- Competing interests
- Disclaimer
- Special issue statement
- Acknowledgements
- Financial support
- Review statement
- References
- Supplement
- Article
(1652 KB) - Full-text XML
- Abstract
- Introduction
- Drivers of Arctic fire regimes
- Future Arctic fire activity
- Arctic fire emissions
- Relevance of fire sources in global and Arctic emissions
- Fire management in the Arctic
- Knowledge gaps and associated uncertainties
- People and future Arctic fire regimes
- Conclusions
- Code and data availability
- Author contributions
- Competing interests
- Disclaimer
- Special issue statement
- Acknowledgements
- Financial support
- Review statement
- References
- Supplement