the Creative Commons Attribution 4.0 License.
the Creative Commons Attribution 4.0 License.
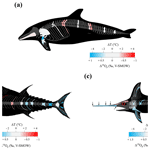
Intra-skeletal variability in phosphate oxygen isotope composition reveals regional heterothermies in marine vertebrates
Romain Amiot
Guillaume Suan
Christophe Lécuyer
François Fourel
Fabien Demaret
Arnauld Vinçon-Laugier
Sylvain Charbonnier
Peggy Vincent
Strategies used by marine vertebrates to regulate their body temperature can result in local variations, and the knowledge of these regional heterothermies is crucial for better understanding the thermophysiologies of extant and extinct organisms. In order to investigate regional heterothermies in vertebrates, we analysed the oxygen isotope composition of phosphatic skeletal elements (δ18Op) of two endothermic fishes (Thunnus thynnus and Xiphias gladius) and three dolphins (two Delphinus delphis delphis and one Cephalorhynchus commersonii kerguelensis). We observed a consistent link between δ18Op variations and temperature heterogeneities recorded by classical methods. Our δ18Op data indicate that: (i) bone hydroxylapatite of the axial skeleton of dolphins mineralise at a warmer temperature than that of the appendicular one, (ii) the skull is the warmest body region in X. gladius, and (iii) T. thynnus possesses high body temperature in the skull and visceral mass region. These results demonstrate the possibility of tracking regional heterothermies in extant marine vertebrates using the δ18Op, paving the way to direct assessment of thermophysiological specificities of both living and extinct vertebrates. From a palaeoenvironmental perspective, the significant observed δ18Op variability questions the use of some taxa or random skeletal elements for the reconstruction of palaeoceanographic parameters such as seawater temperature and δ18O.
- Article
(1312 KB) -
Supplement
(949 KB) - BibTeX
- EndNote
Within vertebrates, ectotherms (e.g. crocodylomorphs, snakes, lizards, turtles, lissamphibians, chondrichthyans and osteichthyans) rely on environmental heat sources to reach their optimal functional body temperature and use behavioural adaptations to maintain it (Carey et al., 1990; McMaster and Downs, 2013). Contrarily, endotherms (birds and mammals) produce their body heat physiologically through metabolic processes (e.g. Cannon and Nedergaard, 2004; Legendre and Davesne, 2020). Maintain a high and constant temperature throughout the body at ambient temperatures below the thermal-neutral zone can be extremely energy-consuming for endothermic homeotherms which maintain their body temperature within ± 2 ∘C (Bligh and Johnson, 1973). Consequently, many of them let the temperature of some areas of the body drop to reduce their energy need and limit heat losses (Irving and Hart, 1957; Rommel et al., 1992; Eichhorn et al., 2011). On the other hand, some ectotherms are able to produce heat locally (Carey, 1982; Block, 1986; Dickson and Graham, 2004) to improve visual acuity in cold environment (Block, 1987; Fritsches et al., 2005), swim faster or migrate over longer distances (Bernal et al., 2001; Blank et al., 2007; Watanabe et al., 2015). These two strategies lead to temperature heterogeneities called regional heterothermies which can be measured on extant organisms thanks to thermometry (Ponganis et al., 2003) and thermal imagery (Hampton et al., 1971; Tattersall et al., 2009). However, such methods suffer from several types of limitation. Indeed, in situ temperature measurements require the handling of the animal, leading to stress-induced and thus punctual rises in body temperature (Bouwknecht et al., 2007), whereas the infrared thermography is inefficient underwater. It is also difficult to apply them to large and rare living organisms, and in any case impossible to apply to extinct ones.
A possible way to track intra-individual temperature heterogeneities and thus regional heterothermies of both extant and extinct marine vertebrates could be the use of the oxygen isotope composition of phosphate (δ18Op) from bioapatite (the mineral forming the bones, teeth and scales of vertebrates). Indeed, vertebrate δ18Op values reflect both the oxygen isotope composition of their body water (δ18Obw), originating from ingested water, food and inhaled dioxygen (Telfer et al., 1970; Hui, 1981; Ortiz, 2001; Rosen and Worthy, 2018), and their body temperature due to the thermo-dependent oxygen isotope fractionation between phosphatic tissues and body fluids (δ18Obw) from which they mineralise in isotope equilibrium (Longinelli and Nuti, 1973; Kolodny et al., 1983; Longinelli, 1984; Luz et al., 1984; Lécuyer et al., 2013). Based on these considerations, it is expected that intra-skeletal δ18Op variability would highlight regional heterothermies in heterotherms. A few studies have investigated the intra-skeletal δ18Op variability in some terrestrial and semi-aquatic extant vertebrates but the relatively reduced number of samples (n<10 per individual) of these datasets considerably limits the significance of the δ18Op variability (Barrick, 1998; Stoskopf et al., 2001; Vennemann et al., 2001; Missell, 2004; Coulson et al., 2008; Clauzel et al., 2020). Some palaeontological studies were focused on the search of regional heterothermies in dinosaurs (Barrick and Showers, 1994, 1995; Barrick et al., 1996, 1998) but the observed variability in δ18Op through the skeleton was difficult to interpret without any present-day isotopic framework and concrete evidence that the isotopic method works for extant animals possessing regional heterothermies (Tomilin, 1950; Carey and Lawson, 1973; Carey, 1982).
In this study, we present new δ18Op data obtained from cephalic, axial and appendicular skeletal elements to document the δ18Op variability in selected marine vertebrates with well-documented regional heterothermies and contrasted thermoregulatory strategies. We compare the obtained δ18Op variations with available body temperature measurements obtained from classical methods and, finally, we discuss the possibility of using this proxy as a tool to identify thermoregulatory strategies and regional heterothermies of both extant and extinct marine vertebrates.
2.1 Sampled specimens
Five wild specimens belonging to four extant fully marine species were studied. They consist of five regional heterotherms, three dolphins (two specimens of Delphinus delphis delphis Linnaeus, 1758 (M.1162 and MNHN-ZM-AC-1876-275) and one specimen of Cephalorhynchus commersonii kerguelensis Robineau et al., 2007 (MNHN-ZM-AC-1983-058)) and two endothermic fishes (one specimen of Thunnus thynnus Linnaeus, 1758; one specimen of Xiphias gladius Linnaeus, 1758). All three dolphin specimens sampled in our study are adult. Dolphins specimens were found stranded on the coasts of western France, Kerguelen archipelago and Algeria (Tables S1 and S5 in the Supplement), and are curated at the Observatoire des mammifères et oiseaux marins (PELAGIS, France) and at the Museum national d'Histoire naturelle (MNHN, Paris, France), while the swordfish (X. gladius) and Atlantic bluefin tuna (T. thynnus) specimens were fished in the western Mediterranean Sea (see Tables S1 and S5). Between 24 and 44 skeletal elements per specimen covering all body regions were analysed for their δ18Op values (Figs. 1a, 2a and b). About 50 mg of each skeletal element were ground into a fine powder using either a Dremel™ diamond-head drill or a mortar and pestle. The cortical part of the bone and areas with minimal physical degradation were selected during the sampling process.
2.2 Oxygen isotope analysis of biogenic apatite phosphate
To measure oxygen isotope ratios of biogenic apatite phosphate by gas mass spectrometry techniques, samples were treated according to the wet chemistry protocol described by Crowson et al. (1991) and slightly modified by Lécuyer et al. (2013). The protocol consists of the isolation of phosphate ions (PO) from apatite as silver phosphate crystals (Ag3PO4). The Ag3PO4 crystals were filtered, dried and cleaned. For each sample, five aliquots of 300 ± 20 µg of Ag3PO4 were mixed with 400 ± 50 µg of graphite in silver foil capsules. Oxygen isotope compositions were measured using a high temperature vario PYRO cube™ elemental analyser (EA) equipped with “purge and trap” technology (Fourel et al., 2011) and interfaced in continuous flow mode to an IsoPrime™ isotopic ratio mass spectrometer (Elementar UK Ltd Cheadle, UK) at the Plateforme d'Ecologie Isotopique du Laboratoire d'Ecologie des Hydrosystèmes Naturels et Anthropisés (LEHNA, UMR5023, Université Claude Bernard Lyon 1, Lyon, France). Pyrolysis of Ag3PO4 was performed at 1450 ∘C. The measurements were calibrated against two standards: a silver phosphate precipitated from the international standards NIST SRM 120c (natural Miocene phosphorite from Florida), and from the NBS 127 (barium sulfate precipitated using seawater from Monterey Bay, California, USA). The NIST SRM 120c δ18Op value was fixed at 21.7 ‰ V-SMOW (Vienna Standard Mean Ocean Water) according to Lécuyer et al. (1993), Chenery et al. (2010) and Halas et al. (2011), and that of NBS 127 set at the certified value of 9.3 ‰ V-SMOW (see Hut, 1987; Halas and Szaran, 2001) for correction of instrumental mass fractionation during CO isotopic analysis. Silver phosphate samples precipitated from standard NIST SRM 120c were repeatedly analysed (δ18Op=21.7 ± 0.3 ‰, n=46) along with the silver phosphate samples derived from bioapatite to ensure that no isotopic fractionation occurred during the wet chemistry. A global analytical error of ± 0.3 ‰ is considered for the whole dataset because the analytical error of the samples δ18Op values is smaller or equal to that of NIST SRM 120c. Data are reported as δ18Op values normalised to V-SMOW (in ‰ δ units).
2.3 Statistical analyses
To increase sample size and statistical power for testing the intra-skeletal variability of δ18Op values, skeletal elements were grouped into several sets corresponding to different parts of the skeleton. The limit between the axial and appendicular skeleton is set at the articulation between the pectoral girdle and the stylopod for dolphins. For Atlantic bluefin tuna and swordfish, the fin rays and fin spines belonging to the fins were considered as appendicular skeleton. For the Atlantic bluefin tuna, we have distinguished anterior and posterior part of the axial skeleton at the limit between precaudal and caudal vertebrae. Since normality and homoscedasticity of the δ18Op values were not validated, we used the non-parametric Mann–Whitney–Wilcoxon to compare median values between two observational series. Statistical tests were performed using R software (R Core Team, 2017) and the level of significance was set at p value < 0.05. All the p values resulting from the statistical tests are reported in Table S4 in the Supplement.
The δ18Op values of D. delphis delphis, C. commersonii kerguelensis, T. thynnus and X. gladius are reported in Tables S2 and S3 in the Supplement. A synthesis is provided in Table 1. Intra-skeletal δ18Op variability is represented in Fig. 1a for the North Atlantic D. delphis delphis and in Fig. 2a and b for osteichthyans. The δ18Op values range from 17.4 ‰ to 19.2 ‰ for the North Atlantic D. delphis delphis, from 20.0 ‰ to 22.5 ‰ for T. thynnus and from 20.0 ‰ to 22.8 ‰ for X. gladius. The results of the two others Delphinidae studied are available in Table 1 and Fig. S1 in the Supplement. Intra-bone variability was measured by paired samples on vertebrae in dolphins and osteichthyans and on fin rays in osteichthyans and is systematically lower than inter-bone variability (Table 1). In dolphins, the maximum intra-bone δ18Op variability (0.5 ‰) is three times smaller than the inter-bone δ18Op variability (1.5 ‰; Table 1). In osteichthyans, the intra-bone δ18Op variability can reach 1.1 ‰ in T. thynnus and 0.4 ‰ in X. gladius but still remains lower to the inter-bone variability (2.5 ‰ for T. thynnus and 2.8 ‰ for X. gladius). As expected, the intra-bone and the inter-bone δ18Op variability is higher in endothermic fishes than dolphins (Table 1). For dolphins, δ18Op values from the axial skeleton are significantly lower than those of the appendicular ones (p values < 0.05; Fig. 1b and Fig. S2 in the Supplement). Teeth δ18Op values of dolphins are higher than those from axial skeletal (Table 1). Nonetheless, the significance of these differences cannot be tested due to the small number of teeth and skull samples (n=1 to 3). In T. thynnus, the highest mean value of 21.6 ± 0.2 ‰ (1 SD, n=6) is recorded in the posterior part of the axial skeleton, whereas the lowest values (Table 1) are recorded in the skull (20.6 ± 0.5 ‰, 1 SD, n=5) and teeth (20.1 ‰, n=1). The skull δ18Op values are significantly lower than those of all the other body parts except from those of the anterior part of the axial skeleton (p value > 0.05; Fig. 2c). The δ18Op values of the skeletal elements belonging to the anterior part of the axial skeleton are significantly lower than those belonging to the posterior part of the axial skeleton (p value < 0.05; Fig. 2c). The mean δ18Op value of X. gladius whole skeleton is 22.0 ± 0.5 ‰ (1 SD, n=33), with the highest mean δ18Op value corresponding to the rostrum (22.3 ± 0.3 ‰, 1 SD, n=5) and the minimum mean value in the skull (20.7 ± 0.6 ‰, n=3; Table 1). No significant differences in δ18Op values are observed between either axial skeleton and fins or axial skeleton and rostrum, but the δ18Op values are significantly different between fins and rostrum (p value < 0.05; Fig. 2c). Despite the small number of samples from the skull (n=3), the δ18Op values from this body region are lower than all the other ones.
Table 1Summary of the mean oxygen isotopic composition (‰, V-SMOW) of dolphins and osteichthyans.
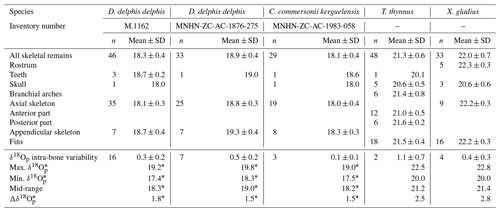
* Teeth are not taken into account in this calculation.
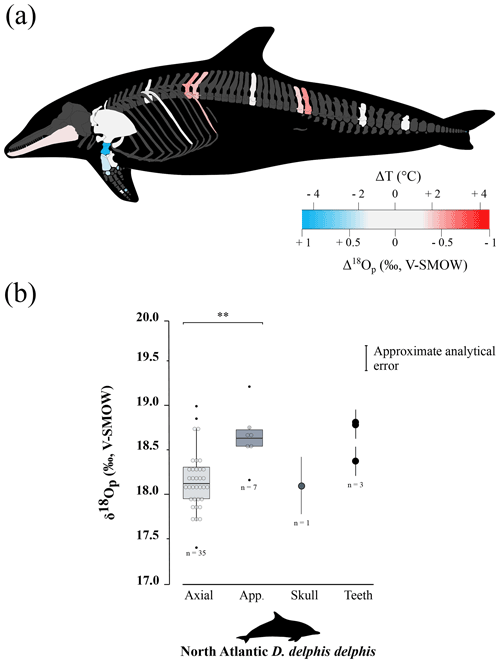
Figure 1(a) Oxygen isotope variability within the skeleton of a North Atlantic D. delphis delphis (M.1162). Bone Δ18Op corresponds to the difference between bone δ18Op value and an average value of the skeleton expressed as its mid-range value ((δ18Omax−δ18O). For paired skeletal elements as well as vertebrae centra and neural spines, the mean value is used. (b) Boxplots showing the δ18Op values of skeletal regions for a North Atlantic D. delphis delphis. Asterisks indicate the significance of the observed differences between pairs of groups ( for p < 0.01). Outliers are plotted as small black circles. Abbreviation: App. (appendicular skeleton).
To sum up, phosphate oxygen isotope compositions reveal variations for all studied specimens: the appendicular skeleton in dolphins is significantly 18O-enriched compared to the axial skeleton. Swordfish has the lowest δ18Op values in the skull and Atlantic bluefin tuna has the lowest δ18Op values in the skull and skeletal elements positioned near the visceral mass.
4.1 Sources of intra-skeletal δ18Op variability
The measured intra-skeletal δ18Op variability may result from two main factors identified as the difference in temperature of bone mineralisation across the skeleton as well as changing isotopic compositions of animal body water. We found significant δ18Op differences (∼ 0.5 ‰) between axial and appendicular bones in dolphins that possess the same mineralisation process, strongly suggesting a dominant temperature control (Fig. 1b). By contrast, the differences in δ18Op recorded between bones and teeth of dolphins (Table 1; Fig. 1b and Fig. S2) also previously observed by Barrick et al. (1992) and Amiot et al. (2008), cannot be exclusively attributed to variable body temperature because these elements mineralise at distinct times during ontogeny and possess different rates of remodelling (Myrick, 1991; Ungar, 2010). Indeed, young dolphins breast-feed during the first 12 to 18 months of their life and ingest maternal milk that is 18O-enriched compared to environmental water (Wright and Schwarcz, 1998). Furthermore, odontocetes possess only one generation of teeth that grow at very slow rate each year until they reach their adult size. It is thus expected that the oxygen isotope composition of teeth is influenced by the 18O-enriched maternal milk unlike bones, which are continuously remodelled, thus erasing the isotopic signal of the early animal's development. Due to the small size of the available teeth, we have sampled and analysed the whole teeth; the δ18Op values therefore integrate the early stages of the animal's development during which it was breast-feed. For osteichthyans such as tuna and billfishes, mineralisation timing should affect δ18Op minimally because all skeletal elements are remodelled (Rosenthal, 1963; Meunier and Huysseune, 1992; Atkins et al., 2014) and teeth are continuously renewed in fishes (Witten and Huysseune, 2009; Tucker and Fraser, 2014). The differences in δ18Op values between skeletal elements with comparable timing of mineralisation and remodelling rates can therefore be confidently attributed to differences in body temperature (Fig. 2c). Besides, all studied vertebrates are nektonic predators that feed on fishes and invertebrates (Young and Cockcroft, 1994; Kastelein et al., 2000), which in turn possess δ18Obw values similar to that of their surrounding water (Picard et al., 1998; Pucéat et al., 2003) but vary depending on the geographical area where they live. The food being the main source of water in dolphins (Telfer et al., 1970; Hui, 1981; Ortiz, 2001; Rosen and Worthy, 2018), the consumption of prey coming from different water masses should cause variations in their δ18Obw. Nevertheless, the seasonal changes in δ18Osw of the water masses in which the sampled marine vertebrates fed are relatively small (± 0.4 ‰; Table S5) and cannot fully explain the inter-bone δ18Op variability reported herein in dolphins and osteichthyans (respectively 1.5 ‰ and 2.5 ‰).
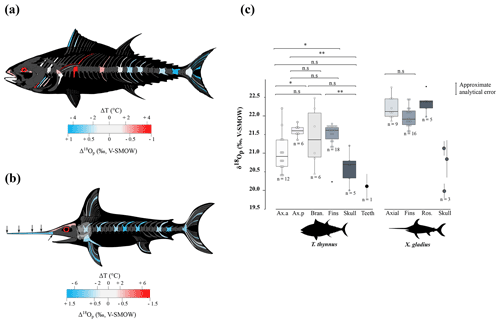
Figure 2Oxygen isotope variability within the skeleton of T. thynnus (a) and X. gladius (b). For each specimen, bone Δ18Op corresponds to the difference between bone δ18Op value and an average value of the skeleton expressed as its mid-range value ((δ18Omax−δ18O). For paired skeletal elements, as well as vertebrae centra and neural spines and fin spines and rays, the mean value is used. The arrows represented on the swordfish's skull represents the precise location of the sampling. (c) Boxplots showing the δ18Op values of skeletal regions for T. thynnus and X. gladius. Asterisks indicate the significance of the observed differences between pairs of groups: ns (not significant) for p > 0.05, * for p < 0.05, for p < 0.01 (highly significant difference). Outliers are plotted as small black circles. Abbreviations: Ax.a (axial anterior), Ax.p (axial posterior), Bran. (branchial arches), Ros. (rostrum).
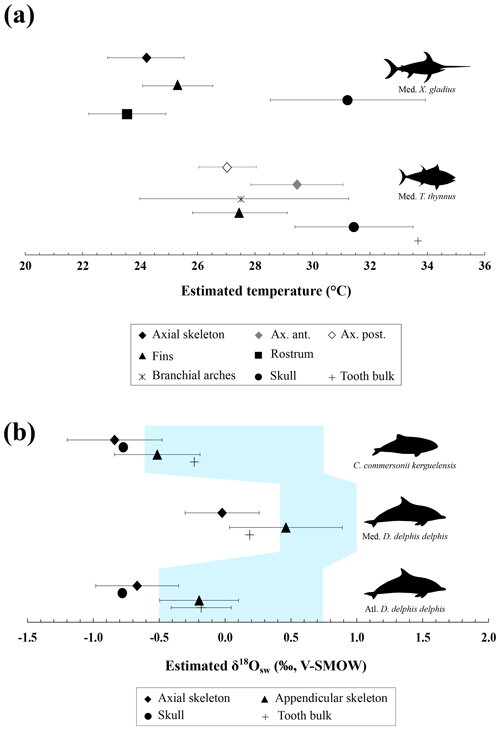
Figure 3(a) Mean estimated hydroxylapatite mineralisation temperature from the phosphate–water oxygen fractionation equation published by Lécuyer et al. (2013), where body water oxygen isotope composition (δ18Obw) for osteichthyans is assumed to be equal to the δ18Osw value. Temperature estimates were done with the mean annual oxygen isotope composition of the Mediterranean Sea (δ18Osw = 1.5 ± 0.4 ‰; Table S5). Error bars correspond to 1 SD. (b) Mean estimated δ18Osw from the phosphate–water oxygen fractionation equation for cetaceans published by Ciner et al. (2016). Error bars correspond to 1 SD and the shaded blue form corresponds to the real measured δ18Osw values (LeGrande and Schmidt, 2006). Abbreviations: Med. (Mediterranean Sea), Atl. (Atlantic Ocean).
Therefore, the link between δ18Op values and the intra-individual body temperature differences previously documented among the studied vertebrates strongly suggest that the recorded isotopic variability is mainly due to differences in mineralisation temperature rather than different timing of mineralisation.
4.2 δ18Op variations linked to regional heterothermies
4.2.1 Marine mammals
Intra-skeletal δ18Op variability of dolphins (mapped in Fig. 1a, and Fig. S1) shows an isotopic enrichment in the appendicular skeleton relative to the axial one. This indicates a lower mineralisation temperature in the appendicular skeleton. This observation is consistent with the thermoregulatory strategies used by cetaceans having a trunk at a nearly constant temperature of 36 ± 2 ∘C (Morrison, 1962; Hampton et al., 1971; Yeates and Houser, 2008), in agreement with their high metabolic activity (Williams et al., 2001), a thick layer of blubber (Lockyer, 1986; Hashimoto et al., 2015) and counter-current heat exchangers which limit heat losses at the extremities (Scholander and Schevill, 1955). Counter-current heat exchangers, defined by a particular spatial arrangement of the cardiovascular system, causes cooling of the blood from the arteries in contact with the veins and results in body temperature proximodistal gradient (Irving and Hart, 1957). The little information available for dolphins mentioned body temperature variation of 9 ∘C in the limbs whereas trunk body temperature remains constant (Tomilin, 1950).
The temperature differences between limb and trunk in the sampled dolphins can be calculated using differences in their δ18Op values and the phosphate–water temperature scale published by Lécuyer et al. (2013):
Assuming only slight seasonal changes in marine mammal δ18Obw, we calculated differences in mineralisation temperature between limbs and trunk of 2 ± 0.5 ∘C for D. delphis delphis, and 1 ± 0.5 ∘C for C. commersonii kerguelensis. In other words, our data show that the mineralisation temperature of the bone is about 2 ∘C lower in the limbs than in the rest of the skeleton in D. delphis delphis and 1 ∘C in C. commersonii kerguelensis. The estimated temperature differences are lower than those recorded by classical methods (respectively 1 and 9 ∘C; Tomilin, 1950). This difference could be explained by the time average recorded in the bones. The time record being long, in the order of several years (Rosenthal, 1963; Riccialdelli et al., 2010; Browning et al., 2014), the estimates inferred from bone δ18Op represent a long-term average value than precise temperature at a specific time and probably mitigate these temperature differences.
4.2.2 Endothermic fishes
Locally high body temperatures have been recorded in several species of tuna (Carey and Lawson, 1973; Graham and Dickson, 2001) and billfishes (Carey, 1982) using classical methods. Heat in tuna is generated in viscera (Carey et al., 1984), red swimming and extraocular muscles (Guppy et al., 1979); this heat is then retained by counter-current heat exchangers (Block and Finnerty, 1994; Dickson and Graham, 2004). Unlike most teleosts, tuna have red muscles positioned close to the spine, limiting heat transfer from the body to the surrounding aquatic medium (Graham and Dickson, 2004). Our δ18Op values and their variations across the body are in agreement with the temperature heterogeneities previously measured by other techniques (e.g. Carey and Teal, 1966; Carey et al., 1971, 1984; Graham and Dickson, 2001), with in particular the lowest δ18Op values measured in the skull and vertebrae near the visceral mass (Table 1 and Fig. 2c). Estimated temperature heterogeneities of tuna assuming slight seasonal changes in δ18Obw are of 2 ± 0.5 ∘C between fins and the visceral mass region and 4 ± 0.5 ∘C between fins and skull (Fig. 3a). These results are consistent with in situ body temperature measurements which indicate a strong thermal gradient ranging from 4 to 20 ∘C but most of the time between 5 and 10 ∘C between core temperature and environmental water depending on both the red muscle activity of the tuna and the temperature of the surrounding water (Carey and Teal, 1966; Carey et al., 1971; Carey and Lawson, 1973; Carey et al., 1984). The δ18Op values of the teeth indicate that they mineralised at a significantly higher temperature than the fins and the posterior part of the axial skeleton. This is the result of the high efficiency of the rete mirabile present near the gills which limits the heat losses associated with ram ventilation (Graham and Dickson, 2001). However, the absolute temperature differences inferred from the two methods are difficult to compare as for dolphins. The high δ18Op variability observed in branchial arches can be explained by variable thermal exchanges between hot blood and cold environmental water.
Swordfish warm the brain and eyes through a unique heater organ associated with the rectus eye muscle (Carey, 1982; Block, 1987) linked to a system of counter-current exchangers and buried in a thick adipose mass that stores the heat produced (Block, 1986, 1991). This mechanism allows the swordfish brain temperature to be 5 to 30 ∘C warmer than the surrounding water while the rest of its body remains close to water temperature (Carey, 1982, 1990; Schwab, 2002; Stoehr et al., 2018). Our δ18Op values and the use of the Eq. (1) (Fig. 3a) indicate that the skull temperature is approximately 7 ± 0.5 ∘C warmer than the rest of the body which is consistent with the in situ temperature measurements (Carey, 1982, 1990; Fritsches et al., 2005).
4.3 Implications for extant and extinct marine vertebrates
The proposed oxygen isotope thermometry complements conventional approaches and thermal imaging methods. The use of oxygen isotopes represents a valuable alternative method to access temperature heterogeneities over the body in marine vertebrates for which loggers are difficult to install and operate. Unlike techniques involving surgical implants (Carey and Teal, 1966; Ponganis et al., 2008), isotopic method does not require the handling of living animals, that can punctually increase their body temperature due to stress (Bouwknecht et al., 2007). Despite the need of already dead specimens from collections or museums, these results open up new perspectives for thermophysiological studies both on extant organisms that are difficult to monitor (e.g. whales) or which are rare (abyssal organisms), but also on extinct marine vertebrates for which only the skeleton is available (e.g. Steller's sea cow, extinct cetaceans and marine reptiles such as ichthyosaurs, plesiosaurs, …). Beyond these (palaeo-)biological implications, our results also highlight a major issue concerning the use of random skeletal elements of marine vertebrates (e.g. chondrichthyans and osteichthyans or cetacean bones and teeth) for the reconstruction of palaeoceanographic parameters based on the oxygen isotope composition of bioapatite (e.g. seawater temperatures and δ18Osw values). Intra-skeletal variability resulting from regional heterothermies can lead to overestimating seawater temperature or overestimating δ18Osw values when applying existing fractionation equations that have been established assuming an isotopic homogeneity of the skeleton, to isolated skeletal elements (Fig. 3a, b). For example, the maximum δ18Op difference of 2.8 ‰ measured between two bones of the swordfish can result in an overestimation of 10 ∘C of seawater temperature when applying the phosphate–water temperature scale of Lécuyer et al. (2013) (Fig. 3a). Along the same lines, the maximum δ18Op difference of 1.8 ‰ measured between two bones of the North Atlantic short-beaked common dolphin can result in an δ18Osw overestimation of 1.7 ‰ when applying the fractionation equation published by Ciner et al. (2016): δ18Ow = 0.95317 (± 0.03293) δ18Op - 17.971 (± 0.605), r=0.97253 (Fig. 3b). It is noteworthy that existing fractionation equations available for chondrichthyans and osteichthyans or cetaceans were established mixing various skeletal elements including axial or appendicular bones and teeth (Longinelli and Nuti, 1973; Kolodny et al., 1983; Yoshida and Miyazaki, 1991; Lécuyer et al., 2013; Ciner et al., 2016). In order to perform accurate palaeoceanographic reconstructions, existing fractionation equations will therefore need to be updated to take into account regional heterothermies.
Detailed intra-skeletal δ18Op mapping allows regional heterothermies in marine vertebrates to be documented. Calculated δ18Op-derived temperatures are consistent with temperature heterogeneities recorded by classical methods (Tomilin, 1950; Carey, 1982; Graham and Dickson, 2001). This opens up new perspectives on the determination of the thermoregulatory strategies of present-day marine vertebrates for which conventional methods of body temperature measurements are difficult to apply. This also allows the investigation of the thermophysiology of extinct vertebrates because the oxygen isotope composition of hydroxylapatite phosphate can be preserved in the fossil record. However, these results highlight the need to update the existing fractionation equations established for chondrichthyans and osteichthyans or cetaceans as they do not consider the significant intra-skeletal δ18Op variability caused by regional heterothermies.
Stable oxygen isotope compositions are provided in Excel tables as electronic Supplement. Information about Atlantic bluefin tuna are also mentioned in Supplement.
The supplement related to this article is available online at: https://doi.org/10.5194/bg-19-2671-2022-supplement.
PV, GS, RA and NS conceived and designed the study. Material preparation and data collection were performed by NS, RA, FD, AVL, SC and PV. Material analysis were performed by NS, RA, FF and CL. The first draft of the manuscript was written by NS, RA and PV, and all authors commented on previous versions of the manuscript. All authors read and approved the final manuscript.
The contact author has declared that neither they nor their co-authors have any competing interests.
Publisher’s note: Copernicus Publications remains neutral with regard to jurisdictional claims in published maps and institutional affiliations.
The authors thank Christine Lefèvre (MNHN) and Willy Dabin (PELAGIS) for providing materials. Finally, we thank editor Steven Bouillon and the two anonymous referees for helpful comments and suggestions.
This research has been supported by the Agence Nationale de la Recherche (grant no. ANR-18-CE31-0020 “Oxymore”).
This paper was edited by Steven Bouillon and reviewed by two anonymous referees.
Amiot, R., Göhlich, U. B., Lécuyer, C., De Muizon, C., Cappetta, H., Fourel, F., Héran, M.-A., and Martineau, F.: Oxygen isotope compositions of phosphate from Middle Miocene–Early Pliocene marine vertebrates of Peru, Palaeogeogr. Palaeocl., 264, 85–92, 2008.
Atkins, A., Dean, M. N., Habegger, M.-L., Motta, P. J., Ofer, L., Repp, F., Shipov, A., Weiner, S., Currey, J. D., and Shahar, R.: Remodeling in bone without osteocytes: billfish challenge bone structure–function paradigms, P. Natl. Acad. Sci. USA, 111, 16047–16052, 2014.
Barrick, R. E.: Isotope paleobiology of the vertebrates: ecology, physiology, and diagenesis, Paleontol. Soc. Pap., 4, 101–137, 1998.
Barrick, R. E. and Showers, W. J.: Thermophysiology of Tyrannosaurus rex: evidence from oxygen isotopes, Science, 265, 222–224, 1994.
Barrick, R. E. and Showers, W. J.: Oxygen isotope variability in juvenile dinosaurs (Hypacrosaurus): evidence for thermoregulation, Paleobiology, 21, 552–560, 1995.
Barrick, R. E., Fischer, A. G., Kolodny, Y., Luz, B., and Bohaska, D.: Cetacean bone oxygen isotopes as proxies for Miocene ocean composition and glaciation, Palaios, 7, 521–531, 1992.
Barrick, R. E., Showers, W. J., and Fischer, A. G.: Comparison of thermoregulation of four ornithischian dinosaurs and a varanid lizard from the Cretaceous Two Medicine Formation: evidence from oxygen isotopes, Palaios, 11, 295–305, 1996.
Barrick, R. E., Stoskopf, M. K., Marcot, J. D., Russell, D. A., and Showers, W. J.: The thermoregulatory functions of the Triceratops frill and horns: heat flow measured with oxygen isotopes, J. Vertebr. Paleontol., 18, 746–750, 1998.
Bernal, D., Dickson, K. A., Shadwick, R. E., and Graham, J. B.: Analysis of the evolutionary convergence for high performance swimming in lamnid sharks and tunas, Comp. Biochem. Physiol. A, 129, 695–726, 2001.
Blank, J. M., Morrissette, J. M., Farwell, C. J., Price, M., Schallert, R. J., and Block, B. A.: Temperature effects on metabolic rate of juvenile Pacific bluefin tuna Thunnus orientalis, J. Exp. Biol., 210, 4254–4261, 2007.
Bligh, J. and Johnson, K. G.: Glossary of terms for thermal physiology, J. Appl. Physiol., 35, 941–961, 1973.
Block, B. A.: Structure of the brain and eye heater tissue in marlins, sailfish, and spearfishes, J. Morphol., 190, 169–189, 1986.
Block, B. A.: Billfish brain and eye heater: a new look at nonshivering heat production, Physiology, 2, 208–213, 1987.
Block, B. A.: Evolutionary novelties: how fish have built a heater out of muscle, Am. Zool., 31, 726–742, 1991.
Block, B. A. and Finnerty, J. R.: Endothermy in fishes: a phylogenetic analysis of constraints, predispositions, and selection pressures, Environ. Biol. Fishes, 40, 283–302, 1994.
Bouwknecht, J. A., Olivier, B., and Paylor, R. E.: The stress-induced hyperthermia paradigm as a physiological animal model for anxiety: a review of pharmacological and genetic studies in the mouse, Neurosci. Biobehav. Rev., 31, 41–59, 2007.
Browning, N. E., Dold, C., I-Fan, J., and Worthy, G. A.: Isotope turnover rates and diet–tissue discrimination in skin of ex situ bottlenose dolphins (Tursiops truncatus), J. Exp. Biol., 217, 214–221, 2014.
Cannon, B. and Nedergaard, J. A. N.: Brown adipose tissue: function and physiological significance, Physiol. Rev., 84, 277–359, 2004.
Carey, F. G.: A brain heater in the swordfish, Science, 216, 1327–1329, 1982.
Carey, F. G.: Further observations on the biology of the swordfish, Plan. Future Billfishes Natl. Coalit. Mar. Conserv. Inc Savannah Ga., edited by: Stroud, R. H., 103–122, 1990.
Carey, F. G. and Lawson, K. D.: Temperature regulation in free-swimming bluefin tuna, Comp. Biochem. Physiol. A Physiol., 44, 375–392, 1973.
Carey, F. G. and Teal, J. M.: Heat conservation in tuna fish muscle, P. Natl. Acad. Sci. USA, 56, 1464, https://doi.org/10.1073/pnas.56.5.1464, 1966.
Carey, F. G., Teal, J. M., Kanwisher, J. W., Lawson, K. D., and Beckett, J. S.: Warm-bodied fish, Am. Zool., 11, 137–143, 1971.
Carey, F. G., Kanwisher, J. W., and Stevens, E. D.: Bluefin tuna warm their viscera during digestion, J. Exp. Biol., 109, 1–20, 1984.
Carey, F. G., Scharold, J. V., and Kalmijn, A. J.: Movements of blue sharks (Prionace glauca) in depth and course, Mar. Biol., 106, 329–342, 1990.
Chenery, C., Müldner, G., Evans, J., Eckardt, H., and Lewis, M.: Strontium and stable isotope evidence for diet and mobility in Roman Gloucester, UK, J. Archaeol. Sci., 37, 150–163, 2010.
Ciner, B., Wang, Y., and Parker, W.: Oxygen isotopic variations in modern cetacean teeth and bones: implications for ecological, paleoecological, and paleoclimatic studies, Sci. Bull., 61, 92–104, 2016.
Clauzel, T., Richardin, P., Ricard, J., Le Béchennec, Y., Amiot, R., Fourel, F., Phouybanhdyt, B., Vinçon-Laugier, A., Flandrois, J.-P., and Lécuyer, C.: The Gauls experienced the Roman Warm Period: Oxygen isotope study of the Gallic site of Thézy-Glimont, Picardie, France, J. Archaeol. Sci. Rep., 34, 102595, https://doi.org/10.1016/j.jasrep.2020.102595, 2020.
Coulson, A. B., Kohn, M. J., Shirley, M., Joyce, W. G., and Barrick, R. E.: Phosphate–oxygen isotopes from marine turtle bones: Ecologic and paleoclimatic applications, Palaeogeogr. Palaeocl., 264, 78–84, 2008.
Crowson, R. A., Showers, W. J., Wright, E. K., and Hoering, T. C.: Preparation of phosphate samples for oxygen isotope analysis, Anal. Chem., 63, 2397–2400, 1991.
Dickson, K. A. and Graham, J. B.: Evolution and consequences of endothermy in fishes, Physiol. Biochem. Zool., 77, 998–1018, 2004.
Eichhorn, G., Groscolas, R., Le Glaunec, G., Parisel, C., Arnold, L., Medina, P., and Handrich, Y.: Heterothermy in growing king penguins, Nat. Commun., 2, 1–7, 2011.
Fourel, F., Martineau, F., Lécuyer, C., Kupka, H.-J., Lange, L., Ojeimi, C., and Seed, M.: 18O 16O ratio measurements of inorganic and organic materials by elemental analysis–pyrolysis–isotope ratio mass spectrometry continuous-flow techniques, Rapid Commun. Mass Spectrom., 25, 2691–2696, 2011.
Fritsches, K. A., Brill, R. W., and Warrant, E. J.: Warm eyes provide superior vision in swordfishes, Curr. Biol., 15, 55–58, 2005.
Graham, J. B. and Dickson, K. A.: Anatomical and physiological specializations for endothermy, Fish Physiol., 19, 121–165, 2001.
Graham, J. B. and Dickson, K. A.: Tuna comparative physiology, J. Exp. Biol., 207, 4015–4024, 2004.
Guppy, M., Hulbert, W. C., and Hochachka, P. W.: Metabolic sources of heat and power in tuna muscles: II. Enzyme and metabolite profiles, J. Exp. Biol., 82, 303–320, 1979.
Halas, S. and Szaran, J.: Improved thermal decomposition of sulfates to SO2 and mass spectrometric determination of δ34S of IAEA SO-5, IAEA SO-6 and NBS-127 sulfate standards, Rapid Commun. Mass Spectrom., 15, 1618–1620, 2001.
Halas, S., Skrzypek, G., Meier-Augenstein, W., Pelc, A., and Kemp, H. F.: Inter-laboratory calibration of new silver orthophosphate comparison materials for the stable oxygen isotope analysis of phosphates, Rapid Commun. Mass Spectrom., 25, 579–584, 2011.
Hampton, I. F. G., Whittow, G. C., Szekerczes, J., and Rutherford, S.: Heat transfer and body temperature in the Atlantic bottlenose dolphin, Tursiops truncatus, Int. J. Biometeorol., 15, 247–253, 1971.
Hashimoto, O., Ohtsuki, H., Kakizaki, T., Amou, K., Sato, R., Doi, S., Kobayashi, S., Matsuda, A., Sugiyama, M., and Funaba, M.: Brown adipose tissue in cetacean blubber, PLoS ONE, 10, e0116734, https://doi.org/10.1371/journal.pone.0116734, 2015.
Hui, C. A.: Seawater consumption and water flux in the common dolphin Delphinus delphis, Physiol. Zool., 54, 430–440, 1981.
Hut, G.: Consultants' Group Meeting on Stable Isotope Reference Samples for Geochemical and Hydrological Investigations, IAEA Vienna 16–18 Sept. 1985 Rep. Dir. Gen. Int. At. Energy Agency Int. At. Energy Agency Vienna, 42, 1987.
Irving, L. and Hart, J. S.: The metabolism and insulation of seals as bare-skinned mammals in cold water, Can. J. Zool., 35, 497–511, 1957.
Irving, L. and Krog, J.: Temperature of skin in the Arctic as a regulator of heat, J. Appl. Physiol., 7, 355–364, 1955.
Kastelein, R. A., Macdonald, G. J., and Wiepkema, P. R.: A note on food consumption and growth of common dolphins (Delphinus delphis), J. Cetacean Res. Manag., 2, 69–74, 2000.
Kolodny, Y., Luz, B., and Navon, O.: Oxygen isotope variations in phosphate of biogenic apatites, I. Fish bone apatite – rechecking the rules of the game, Earth Planet. Sc. Lett., 64, 398–404, 1983.
Lécuyer, C., Grandjean, P., O'Neil, J. R., Cappetta, H., and Martineau, F.: Thermal excursions in the ocean at the Cretaceous – Tertiary boundary (northern Morocco): δ18O record of phosphatic fish debris, Palaeogeogr. Palaeocl., 105, 235–243, 1993.
Lécuyer, C., Amiot, R., Touzeau, A., and Trotter, J.: Calibration of the phosphate δ18O thermometer with carbonate–water oxygen isotope fractionation equations, Chem. Geol., 347, 217–226, 2013.
Legendre, L. J. and Davesne, D.: The evolution of mechanisms involved in vertebrate endothermy, Philos. Trans. R. Soc. B, 375, 20190136, https://doi.org/10.1098/rstb.2019.0136, 2020.
LeGrande, A. N. and Schmidt, G. A.: Global gridded data set of the oxygen isotopic composition in seawater, Geophys. Res. Lett., 33, L12604, https://doi.org/10.1029/2006GL026011, 2006.
Linnæus, C.: Systema naturæ per regna tria naturæ, secundum classes, ordines, genera, species, cum characteribus, differentiis, synonymis, locis, Tomus Ed. Decima Reformata 1–41–824 HolmiæSalvius, 1758.
Lockyer, C.: Body fat condition in Northeast Atlantic fin whales, Balaenoptera physalus, and its relationship with reproduction and food resource, Can. J. Fish. Aquat. Sci., 43, 142–147, 1986.
Longinelli, A.: Oxygen isotopes in mammal bone phosphate: a new tool for paleohydrological and paleoclimatological research?, Geochim. Cosmochim. Ac., 48, 385–390, 1984.
Longinelli, A. and Nuti, S.: Revised phosphate-water isotopic temperature scale, Earth Planet. Sc. Lett., 19, 373–376, 1973.
Luz, B., Kolodny, Y., and Horowitz, M.: Fractionation of oxygen isotopes between mammalian bone-phosphate and environmental drinking water, Geochim. Cosmochim. Ac., 48, 1689–1693, 1984.
McMaster, M. K. and Downs, C. T.: Thermal variability in body temperature in an ectotherm: Are cloacal temperatures good indicators of tortoise body temperature?, J. Therm. Biol., 38, 163–168, 2013.
Meunier, F. J. and Huysseune, A.: The concept of bone tissue in Osteichthyes, Neth. J. Zool., 42, 445–458, https://doi.org/10.1163/156854291X00441, 1992.
Missell, C. A.: Thermoregulatory adaptations of Acrocanthosaurus atokensis – evidence from oxygen isotopes, North Carolina State University, 82 pp., 2004.
Morrison, P.: Body temperatures in some Australian mammals. III. Cetacea (Megaptera), Biol. Bull., 123, 154–169, 1962.
Myrick, A. C.: Some new potential uses of dental layers in studying delphinid populations, in: Dolphin societies, edited by: Pryor, K. and Norris, K. S., University of California Press, Berkeley, CA, 251–279, 1991.
Ortiz, R. M.: Osmoregulation in marine mammals, J. Exp. Biol., 204, 1831–1844, 2001.
Picard, S., Garcia, J.-P., Lécuyer, C., Sheppard, S. M., Cappetta, H., and Emig, C. C.: δ18O values of coexisting brachiopods and fish: Temperature differences and estimates of paleo–water depths, Geology, 26, 975–978, 1998.
Ponganis, P. J., Van Dam, R. P., Levenson, D. H., Knower, T., Ponganis, K. V., and Marshall, G.: Regional heterothermy and conservation of core temperature in emperor penguins diving under sea ice, Comp. Biochem. Physiol. A. Mol. Integr. Physiol., 135, 477–487, 2003.
Ponganis, P. J., Kreutzer, U., Stockard, T. K., Lin, P.-C., Sailasuta, N., Tran, T.-K., Hurd, R., and Jue, T.: Blood flow and metabolic regulation in seal muscle during apnea, J. Exp. Biol., 211, 3323–3332, 2008.
Pucéat, E., Lécuyer, C., Sheppard, S. M., Dromart, G., Reboulet, S., and Grandjean, P.: Thermal evolution of Cretaceous Tethyan marine waters inferred from oxygen isotope composition of fish tooth enamels, Paleoceanography, 18, 1–12, 2003.
R Core Team: R: A language and environment for statistical computing, Vienna, Austria, R Foundation for Statistical Computing, 2017.
Riccialdelli, L., Newsome, S. D., Fogel, M. L., and Goodall, R. N. P.: Isotopic assessment of prey and habitat preferences of a cetacean community in the southwestern South Atlantic Ocean, Mar. Ecol. Prog. Ser., 418, 235–248, 2010.
Robineau, D., Goodall, R. N. P., Pichler, F., and Baker, C. S.: Description of a new subspecies of Commerson's dolphin, Cephalorhynchus commersonii (Lacépède, 1804), inhabiting the coastal waters of the Kerguelen Islands, Mammalia, 71, 172–180, https://doi.org/10.1515/MAMM.2007.034, 2007.
Rommel, S. A., Pabst, D. A., McLellan, W. A., Mead, J. G., and Potter, C. W.: Anatomical evidence for a countercurrent heat exchanger associated with dolphin testes, Anat. Rec., 232, 150–156, 1992.
Rosen, D. A. and Worthy, G. A.: Nutrition and energetics, in: CRC handbook of marine mammal medicine, CRC Press, 695–738, 3rd Edn., ISBN 978-1-31514-4-931, 2018.
Rosenthal, H. L.: Uptake, turnover and transport of bone seeking elements in fishes, Ann. N. Y. Acad. Sci. US, 109, 278–293, https://doi.org/10.1111/j.1749-6632.1963.tb13472.x, 1963.
Scholander, P. F. and Schevill, W. E.: Counter-current vascular heat exchange in the fins of whales, J. Appl. Physiol., 8, 279–282, 1955.
Schwab, I. R.: These eyes are hot…, Br. J. Ophthalmol., 86, 266–266, 2002.
Stoehr, A., St. Martin, J., Aalbers, S., Sepulveda, C., and Bernal, D.: Free-swimming swordfish, Xiphias gladius, alter the rate of whole body heat transfer: morphological and physiological specializations for thermoregulation, ICES J. Mar. Sci., 75, 858–870, 2018.
Stoskopf, M. K., Barrick, R. E., and Showers, W. J.: Oxygen isotope variability in bones of wild caught and constant temperature reared sub-adult American alligators, J. Therm. Biol., 26, 183–191, 2001.
Tattersall, G. J., Andrade, D. V., and Abe, A. S.: Heat exchange from the toucan bill reveals a controllable vascular thermal radiator, Science, 325, 468–470, 2009.
Telfer, N., Cornell, L. H., and Prescott, J. H.: Do dolphins drink water?, J. Am. Vet. Med. Assoc., 157, 555–558, 1970.
Tomilin, A. G.: Notes on Siberian white-sided dolphin, Rybn. Khozaistvo, 26, 50–53, 1950.
Tucker, A. S. and Fraser, G. J.: Evolution and developmental diversity of tooth regeneration, Seminars in Cell & Developmental Biology, 25–26, 71–80, https://doi.org/10.1016/j.semcdb.2013.12.013, 2014.
Ungar, P. S.: Mammal teeth: origin, evolution, and diversity, John Hopkins University Press, https://doi.org/10.1353/book.485, 2010.
Vennemann, T. W., Hegner, E., Cliff, G., and Benz, G. W.: Isotopic composition of recent shark teeth as a proxy for environmental conditions, Geochim. Cosmochim. Ac., 65, 1583–1599, 2001.
Watanabe, Y. Y., Goldman, K. J., Caselle, J. E., Chapman, D. D., and Papastamatiou, Y. P.: Comparative analyses of animal-tracking data reveal ecological significance of endothermy in fishes, P. Natl. Acad. Sci. USA, 112, 6104–6109, 2015.
Williams, T. M., Haun, J., Davis, R. W., Fuiman, L. A., and Kohin, S.: A killer appetite: metabolic consequences of carnivory in marine mammals, Comp. Biochem. Physiol. A. Mol. Integr. Physiol., 129, 785–796, 2001.
Witten, P. E. and Huysseune, A.: A comparative view on mechanisms and functions of skeletal remodelling in teleost fish, with special emphasis on osteoclasts and their function, Biol. Rev., 84, 315–346, 2009.
Wright, L. E. and Schwarcz, H. P.: Stable carbon and oxygen isotopes in human tooth enamel: identifying breastfeeding and weaning in prehistory, Am. J. Phys. Anthropol. Off. Publ. Am. Assoc. Phys. Anthropol., 106, 1–18, 1998.
Yeates, L. C. and Houser, D. S.: Thermal tolerance in bottlenose dolphins (Tursiops truncatus), J. Exp. Biol., 211, 3249–3257, 2008.
Yoshida, N. and Miyazaki, N.: Oxygen isotope correlation of cetacean bone phosphate with environmental water, J. Geophys. Res. Oceans, 96, 815–820, 1991.
Young, D. D. and Cockcroft, V. G.: Diet of common dolphins (Delphinus delphis) off the south-east coast of southern Africa: opportunism or specialization?, J. Zool., 234, 41–53, 1994.