the Creative Commons Attribution 4.0 License.
the Creative Commons Attribution 4.0 License.
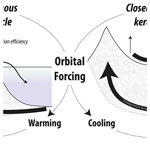
Ideas and perspectives: Emerging contours of a dynamic exogenous kerogen cycle
Thomas M. Blattmann
Growing evidence points to the dynamic role that kerogen is playing on Earth's surface in controlling atmospheric chemistry over geologic time. Although quantitative constraints on the weathering of kerogen remain loose, its changing weathering behavior modulated by the activity of glaciers suggests that this largest pool of reduced carbon on Earth may have played a key part in atmospheric CO2 variability across recent glacial–interglacial cycles and beyond. This work enunciates the possibility of kerogen oxidation as a major driver of atmospheric CO2 increase in the wake of glacial episodes. This hypothesis of centennial- and millennial-timescale relevance for this chemical weathering pathway is substantiated by several lines of independent evidence synthesized in this contribution, including the timing of atmospheric CO2 increase, atmospheric CO2 isotope composition (13C and 14C), kerogen oxidation kinetics, observations of kerogen reburial, and modeling results. The author hypothesizes that the deglaciation of kerogen-rich lithologies in western Canada contributed to the characteristic deglacial increase in atmospheric CO2, which reached an inflection point ≤ 300 years after the Laurentide Ice Sheet retreated into the kerogen-poor Canadian Shield. To reconcile the release of isotopically light carbon via kerogen oxidation with Earth surface carbon pool constraints, major oceanic degassing and biospheric regrowth must have acted in concert across glacial–interglacial transitions. Additionally, a process such as a strong shift in the ratio of C3 to C4-derived organic matter must be invoked to maintain isotope mass balance, a point key for reconciling the hypothesis with the carbon isotope record of marine dissolved inorganic carbon. In order to test this hypothesis, quantitative constraints on the contribution of kerogen oxidation to CO2 rise at glacial terminations are needed through systematic studies on (1) CO2 fluxes emanating from the weathering of different lithologies, (2) oxidation kinetics of kerogen along glacial chronosequences, and (3) high-resolution temporal changes in the aerial extent of glacially exposed lithological units and glacial flour.
- Article
(4492 KB) - Full-text XML
-
Supplement
(205 KB) - BibTeX
- EndNote
Over geologic timescales, atmospheric CO2 is controlled by the combined effects of chemical weathering of silicates and carbonates and the organic carbon cycle (Berner et al., 1983; Berner, 1990; Torres et al., 2014). Organic carbon in the form of kerogen1 comprises around 15×106 Pg C, which encompasses over 99.9 % of reduced carbon present on Earth. Tectonic uplift and denudation subjects 150 Pg C kyr−1 of kerogen to weathering on Earth's surface (Hedges and Oades, 1997), thereby facilitating entry of this geologically ancient carbon into the atmosphere. Upon oxidation of kerogen, O2 is consumed and CO2 is released to the atmosphere. In reverse, biospheric organic carbon burial in marine sediments removes carbon from Earth's surface, thereby drawing down atmospheric CO2 and increasing O2 over geological timescales (Galy et al., 2008). Therefore, kerogen weathering and sedimentary organic matter burial in the ocean play compensatory roles in governing atmospheric chemistry (Fig. 1) with kerogen oxidation considered important for atmospheric chemistry over million-year timescales (e.g., Petsch, 2014; Bolton et al., 2006).
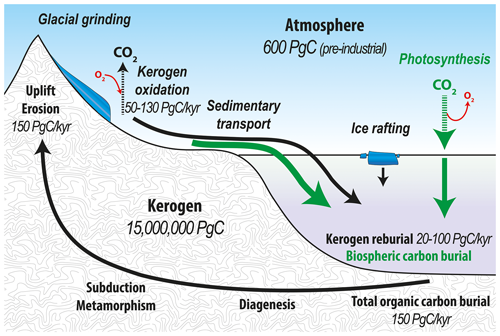
Figure 1Organic carbon cycle with the flow of kerogen (solid black lines) and the flow of biospheric carbon (solid green lines) showing the fixation of atmospheric CO2 by both terrestrial and marine primary productivity. The combined flux of reworked kerogen and biospheric carbon into ocean sediments constitutes the total organic carbon burial into the endogenous kerogen pool (Galy et al., 2015; Hedges and Oades, 1997).
However, the decay of kerogen on Earth's surface is incomplete (e.g., Hemingway et al., 2018; Leythaeuser, 1973), with physical erosion followed by riverine transport (Galy et al., 2015) and reburial in lacustrine and marine settings (e.g., Blattmann et al., 2018a, 2019b; Cui et al., 2016; Sackett et al., 1974; Sparkes et al., 2020). The operation of this “simple cycle besides the more complicated common circulation of carbon”, enunciated by Sauramo (1938), begs the following questions: (i) what is the reburial efficiency of kerogen, (ii) what is the weathering efficiency of kerogen, (iii) what are their controlling factors, and (iv) how do reburial and weathering efficiency vary over geologic time and space? The answers presented will suggest that the exogenous kerogen cycle behaves dynamically, with this contribution hypothesizing a connection between kerogen oxidation and millennial/centennial-scale atmospheric CO2 increases during glacial terminations.
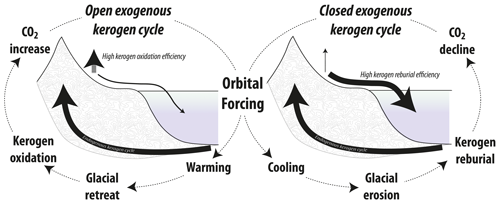
Figure 2Conceptual hypothesis overview: changes in kerogen reburial efficiency and its effect on the reentry of ancient carbon into surficial carbon pools as a function of overall climate state. During glacial times, kerogen reburial is promoted by the activity of glaciers and ice sheets, with relatively little oxidation of this carbon during its transit across Earth's surface characteristic of a “closed” exogenous kerogen cycle. During glacial terminations and interglacials, the oxidation of kerogen is more efficient, leading to the exhalation of this carbon to the atmosphere characteristic of an “open” exogenous kerogen cycle.
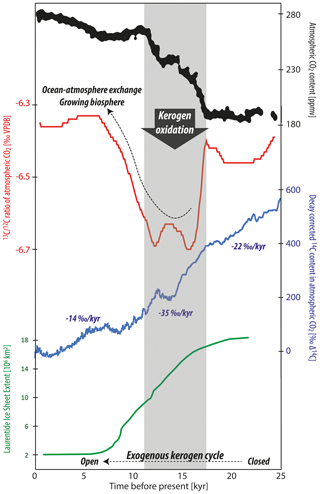
Figure 3Evolution of atmospheric CO2 and its carbon isotope composition starting from the Last Glacial Maximum with redrawn data from Schmitt et al. (2012) and 14C data from Reimer et al. (2013). The area of the Laurentide Ice Sheet (including Greenland) is plotted after Dalton et al. (2020). The gray time envelope indicates the greatest rate of atmospheric CO2 increase coinciding with a pronounced negative pulse in stable carbon isotope composition and accelerated decrease in radiocarbon concentration. In addition to the hypothesized role of kerogen oxidation, the superimposed effects of ocean–atmosphere CO2 exchange and a growing terrestrial biosphere must have contributed in a major way to the evolution of these parameters.
Driven mainly by orbital cyclicity, glaciers have rhythmically waxed and waned across Earth's surface together with changes in atmospheric chemistry, including the greenhouse gas CO2 (Hays et al., 1976: Barnola et al., 1987). The amount and carbon isotope composition of atmospheric CO2 (Fig. 2) depend on (1) the terrestrial biospheric organic carbon pool, (2) the dissolved inorganic carbon (DIC) pools residing in the surface and deep ocean, (3) exchanges between the atmosphere and the terrestrial biosphere and ocean, and (4) the export of organic matter and carbonate from the surface to deep waters and sediments of the oceans (Sigman and Boyle, 2000). During deglaciation, an increasingly large terrestrial biospheric carbon pool (Bird et al., 1994; Shackleton, 1977; Shackleton et al., 1983) is inferred to have controlled an increase in the stable carbon isotope composition of marine DIC, reconstructed primarily from foraminifera (e.g., Schmittner et al., 2017). However, during times of the most rapid CO2 increase during transitions from glacial to interglacial periods, negative stable carbon isotope shifts in atmospheric CO2 occurred (Fig. 3; Smith et al., 1999; Schmitt et al., 2012). This is a strong indicator that, in conjunction with ocean–air exchange, respired organic matter acted as a direct source of carbon to the atmosphere (Bauska et al., 2016). Organic matter can be categorized into biospheric and lithogenic (i.e., kerogen) forms, with both exhibiting similar 13C signatures yet modern and dead 14C signatures, respectively (e.g., Lewan, 1986; Meyers, 1994). The constraints imposed by radiocarbon indicate there was a source of carbon to the atmosphere and ocean depleted in or devoid of radiocarbon (Broecker and Clark 2010; Hain et al., 2014; Rafter et al., 2019; Zhao et al., 2018), thereby limiting potential contributions from modern biospheric organic carbon sources. Other studies have proposed that carbon sourced from deep ocean DIC was the predominant source for carbon transferred to the atmosphere during glacial terminations (e.g., Hain et al., 2014). However, this hypothesis appears inconsistent with the negative fluctuation observed in the 13C fingerprint of atmospheric CO2 (see discussion in Broecker and McGee, 2013). This leaves us with some apparent contradictions. If there was a source of oxidized organic matter to the atmosphere, how is this reconcilable with the changes in the carbon isotope trajectory of marine DIC? From the author's perspective, this is where a new idea is needed.
Table 1Carbon budget example illustrating a possible partitioning of carbon between pools mirroring the characteristic features of the stable carbon isotope trajectory of marine DIC and atmospheric CO2 from the Last Glacial Maximum into the Holocene working with the idea of kerogen oxidation (see also Fig. 3).
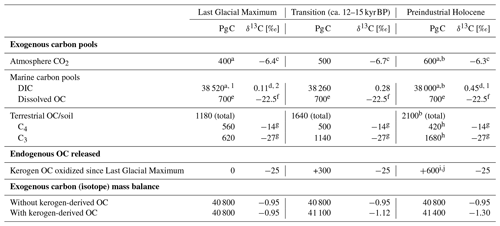
Numerical constraints from/modified after a Ciais et al. (2012), b Sigman and Boyle (2000), c Schmitt et al. (2012), d Peterson et al. (2014), e Hedges and Oades (1997), f Beaupré (2015), g Meyers (1994), h Still et al. (2003), i Zeng (2003), and j Simmons et al. (2016). 1 The difference between Last Glacial Maximum and Holocene DIC size was set at 520 Pg C following Ciais et al. (2012). 2 The Last Glacial Maximum global ocean value representing 0.5–5 km depth was used and 0.34 ‰ was added to represent the isotope shift into the Holocene (Peterson et al., 2014). Values rounded to the nearest 10 Pg C and 0.01 ‰.
Unlike the global, nearly unison rhythm of the glacial–interglacial marine oxygen isotope record, the global deglacial increase in carbon isotopes shows a notable exception: for much of the North Atlantic, the Holocene stable carbon isotope values of DIC are lighter than those of the Last Glacial Maximum (e.g., Peterson et al., 2014; Broecker and McGee, 2013; Bouttes et al., 2020). From the author's perspective, this is notable because the northernmost Atlantic is the locus of major downwelling driving global thermohaline circulation (de Carvalho Ferreira and Kerr, 2017). The negative shift observed in the North Atlantic was modeled by Crichton et al. (2016), showing this is explainable by the marine uptake and subduction of light carbon released to the atmosphere by terrestrial organic matter oxidation (hypothesized as permafrost in their case). Furthermore, Crichton et al. (2016) model the positive carbon isotope shift in DIC observed in the South Atlantic, demonstrating that contemporaneous positive and negative shifts in different ocean sectors are reconcilable with the release of isotopically light carbon from land. On a global scale, how could the stable carbon isotope mass balance add up if a scenario of organic matter oxidation is assumed? In addition, is it reconcilable with the global marine DIC carbon isotope shift? An example of such a budget is shown in Table 1, which pegs atmospheric CO2 and marine DIC stable carbon isotope shifts to recorded values and uses constraints of carbon pools sizes (see table caption for references). While the constraints lead to an array of solutions depending on the degrees of freedom chosen and which literature values one accepts for the various parameters, a plausible budget is attempted here. Based on this scenario of 600 Pg C released to the atmosphere by kerogen oxidation and 520 Pg C degassed from the oceans, isotope mass balance constraints suggest a glacial–interglacial regrowth of the biosphere on the order of 920 Pg C. To maintain isotope mass balance, this solution assumes a constant marine dissolved OC pool size and requires a strong increase in the ratio of C3 to C4 vegetation-derived biomass from approximately 1:1 until the ratio reaches the modern-day value of about 4:1 (Still et al., 2003), which is plausible given the large-scale change in vegetation across glacial–interglacial cycles (Adams et al., 1990; Huang et al., 2001). The terrestrial OC pool increase of 920 Pg C is larger than usually computed using carbon isotopes of marine DIC but lies in the ballpark expected from palynological observations (see Table 1 in Zeng, 2003; see also Crowley, 1995, and discussion in Jeltsch-Thömmes et al., 2019). These are back-of-the-envelope calculations as constraints like the glacial–interglacial change in the carbon isotope composition of DIC carry heavy weight and considerable uncertainty (+0.34 ± 0.19 ‰ 2σ, Peterson et al., 2014). Additionally, the fitted values C4 and C3 vegetation amounts are sensitive to other parameters such as the change in marine DIC pool size and are therefore limited in their value to proof of concept: these calculations show that the release of CO2 from organic matter such as kerogen is compatible with (1) the negative perturbation in atmospheric CO2 during the glacial–interglacial transition, (2) the increase in the carbon isotope composition of global marine DIC, and (3) general carbon pool size constraints. Complementing the widely supported hypotheses of an increasing terrestrial biospheric organic carbon pool and major changes in ocean–atmosphere CO2 exchange (e.g., Shackleton, 1977; Lindgren et al., 2018; Sigman and Boyle, 2000), the author will propose a major release of CO2 to the atmosphere via kerogen oxidation during deglaciation.
Chemical weathering of silicates and carbonates as well as the burial and oxidation of organic matter exert fundamental control over atmospheric chemistry on Earth over geologic timescales (Berner et al., 1983; Berner, 1990: Hilton and West, 2020; Petsch, 2014). On modern-day Earth, carbon sources and sinks from mineral weathering (i.e., silicates and carbonates via carbonic and sulfuric acids) and biogeochemical fluxes (i.e., organic matter burial and kerogen oxidation) stand in fine balance to one another with contrasting concoctions of these under different tectonosedimentary settings (e.g., Bufe et al., 2021; Hilton and West, 2020; Blattmann et al., 2019a; Horan et al., 2019). Deglaciation likely modulated silicate and carbonate weathering, resulting in enhanced (temporary) drawdown of atmospheric CO2 (e.g., Tranter, 1996; Gibbs and Kump, 1996; Munhoven and François 1996; cf. Schachtman et al., 2019). Glaciers have been invoked as agents for accelerating chemical weathering of carbonate and silicate minerals by increasing sediment yield and creating a high-surface-area, reactive substrate (Torres et al., 2017; Vance et al., 2009; Yu et al., 2021), with carbonate weathering constituting a source of CO2 to the atmosphere when sulfuric acid, stemming from oxidized sulfide minerals, is involved (see Torres et al., 2014; Kölling et al., 2019). In the following, the idea of glaciers producing reactive substrate susceptible to chemical weathering is reviewed and explored for kerogen.
Evidence accumulated from over a century of scientific studies supports the idea that the reburial of kerogen was more extensive during cold interludes in Earth history where glacial erosion and ice rafting was widespread (Blattmann et al., 2018b). This general pattern of kerogen reburial is observed spatially today with more efficient reburial in high-latitude glaciated regions than in low-latitude sedimentary systems (Cui et al., 2016). This enhanced reburial efficiency strengthens the short-circuiting of the exogenous kerogen cycle by keeping this ancient, reduced carbon locked away. However, under glaciofluvial conditions, the entry of kerogen-bound carbon into surficial carbon pools is promoted (Horan, 2018), with glacial meltwater releasing dissolved organic matter from kerogen, which is readily degraded and consumed by microbes (Hood et al., 2009; Schillawski and Petsch, 2008; Sharp et al., 1999). Additionally, frost shattering, together with the retreat of glaciers, exposes finely ground, high surface area, reactive sediment, thereby accelerating oxidation and the release of kerogen-derived CO2 to the atmosphere (Fischer and Gaupp, 2005; Horan et al., 2017). The initially strong input of kerogen-derived CO2 would die down as the availability of glacially ground, reactive kerogen declines into an interglacial period. Enhanced degradation of kerogen in the wake of glacial episodes is consistent with observations from areas of ongoing deglaciation (Horan et al., 2017). Contemporaneous perturbations in CO2 fluxes are expected to result for silicate and carbonate weathering via carbonic and sulfuric acids (Vance et al., 2009; Torres et al., 2014). However, kerogen oxidation, owing to its faster weathering kinetics and direct conversion to CO2, leads to considerable losses in kerogen content over decadal timescales (Horan et al., 2017, and references therein; Fischer et al., 2007). This is a process by which CO2 can be injected directly into the atmosphere and impact glacial–interglacial cycles (Fig. 2).
In the following, quantitative arguments are presented: of the approximately 150 Pg C kyr−1 of kerogen reaching the Earth's surface (FL; Hedges and Oades, 1997), Pg C kyr−1 is currently exported by rivers to oceans (FL-O; Galy et al., 2015; Copard et al., 2007), indicating that the modern-day reburial efficiency of this carbon lies in the ballpark of 30 % (10 %–70 %). Normalized to global land area (AL = 149 000 000 km2), the flux of carbon due to oxidation of kerogen to the atmosphere (FL-A) can be calculated from
Table 2Fluxes of kerogen oxidation normalized to 1 year from on-site (soil and outcrops), catchment-wide studies (river), and laboratory-based incubation studies ordered approximately from small to large CO2 release fluxes. For calculations, see the Supplement.
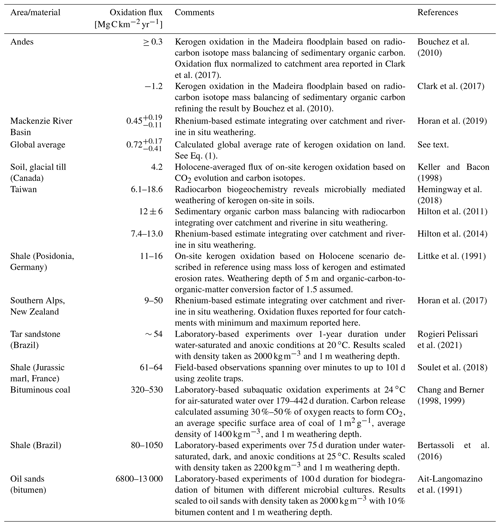
Here, Eq. (1) yields a modern-day global FL-A of . Reports of local and regional CO2 fluxes from kerogen oxidation are based on disparate study sites and methods (Table 2). From this, reported CO2 fluxes stemming from oxidation of rock-disseminated forms of kerogen and across larger catchment areas under aerobic conditions span over 2 orders of magnitude ranging from 0.3 to 64 . The only quantities available for catchments of ongoing deglaciation stem from the Southern Alps of New Zealand, which are 1 to 2 orders of magnitude faster than those of the average Earth surface (ranging between 9–50 ; Horan et al., 2017). Taking this flux range as a working estimate, how would kerogen oxidation translate into changes in atmospheric CO2 concentration? In Zeng's (2003) modeling work postulating soil oxidation upon retreat of overriding glaciers, the effect of continuous inputs of organic-matter-derived carbon directly to the atmosphere is calculated: 550 Pg C oxidized leads to a 60 Pg C increase in atmospheric CO2 (30 ppm increase). Similarly, in their modeling, Simmons et al. (2016) also propose the oxidation of overridden soil organic carbon subsequent to deglaciation and calculate a 600 Pg C release resulting in a 40–60 ppm increase in atmospheric CO2 (see also Ciais et al., 2012, who calculate the deglacial oxidation of 700 Pg C of an “inert” terrestrial organic carbon pool). The idea of soil oxidation is mathematically analogous to kerogen oxidation in the hypothesis presented here. How much area of deglaciated terrain (AD) would therefore be needed to release 550–600 Pg C of kerogen-derived carbon? Using Zeng's (2003) and Simmons et al.'s (2016) carbon release estimates (550 Pg C ≤ CR ≤ 600 Pg C) and Horan et al.'s (2017) kerogen oxidation kinetics (9 ≤ FKO ≤ 50 ) over a 6000-year time window (TG-I, time span of pronounced CO2 increase over the glacial–interglacial transition; see Fig. 3) and subtracting global average baseline kerogen oxidation ( ; see Eq. 1),
With calculated AD yielding between 2 and 12×106 km2, in the most conservative estimate, 12×106 km2 of deglaciated terrain containing sedimentary and metasedimentary rocks and their debris (glacial flour accumulated over millennia of glaciation) would cover the source. In comparison, Canada covers approximately 10×106 km2, which was mostly covered by the Laurentide Ice Sheet during the Last Glacial Maximum. With this, a plausible scenario for releasing kerogen-derived CO2 to the atmosphere that could account for a 30–60 ppm rise during the glacial–interglacial transition encompassing an area equal to or less than the terrestrial extent of the Laurentide Ice Sheet is identified. Similar to the oxidation fluxes reported from deglaciating catchments (Horan et al., 2017) are those reported for the in situ weathering of massive outcrop formations of marls and shales (e.g., Soulet et al., 2018; Littke et al., 1991; see Table 2). Kerogen oxidation fluxes appear higher for catchments showing larger glacial coverage (Horan et al., 2017), suggesting that the relative contribution of freshly exposed and ground bedrock is highest for these areas and that kerogen oxidation fluxes progress over the course of deglaciation. The CO2 flux emanating from kerogen oxidation in soils formed from glacial till (Keller and Bacon, 1998) suggests that oxidation fluxes an order of magnitude greater than the global average can sustain for millennia after deglaciation. However, a systematic chronosequence understanding that allows conclusions to be drawn is currently lacking as a variety of factors (e.g., lithology, initial kerogen content, grain size) exert control over kerogen oxidation (cf. Fischer and Gaupp, 2005; Bolton et al., 2006; Martínez and Escobar, 1995). The bedrock and river sediments of the Southern Alps are relatively lean in kerogen (Copard et al., 2007; Horan et al., 2017). Therefore, for deglaciating landscapes exposing glacially ground kerogen-rich lithologies, larger oxidation fluxes than those reported from the Southern Alps can be expected, increasing and decreasing the plausible ranges for FKO and AD, respectively (see next section).
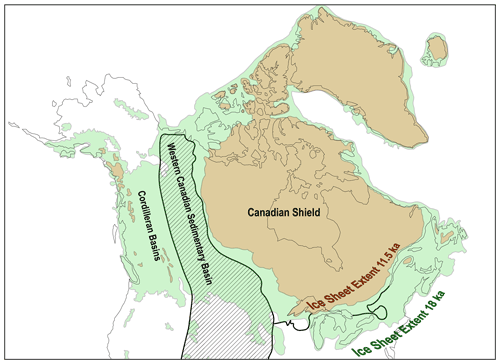
Figure 4Laurentide Ice sheet extents bracketing the time span of the most pronounced glacial–interglacial CO2 increase (redrawn after Dalton et al., 2020). The Western Canadian Sedimentary Basin (hatched area, with extension of related units into the United States) and the Cordilleran Basins are to the west of the Canadian Shield (simplified after Miall and Blakey, 2019). The southern border of the Canadian Shield is drawn with mainly Phanerozoic sedimentary units to the south (simplified after Reed et al., 2004). The surficial kerogen content in the Canadian Shield is generally low, while high kerogen contents are present along the axes of the Cordilleran Basins and Western Canadian Sedimentary Basin (see Fig. 2 in Copard et al., 2007). Less than or equal to 300 years after glacial retreat extended into the Canadian Shield, the rise in atmospheric CO2 subsided (compare with Fig. 3). Here, kerogen oxidation in western Canada is hypothesized to have acted as a major source of CO2 to the atmosphere in the direct wake of glacial retreat.
Long-term accelerated decline in the radiocarbon concentration of atmospheric CO2 parallel to an overall increase in CO2 amount occurred since the Last Glacial Maximum (Reimer et al., 2013; Roth and Joos, 2013). Consistent with such a dilutional process, kerogen oxidation releases radiocarbon-dead CO2 to the atmosphere. During the time period of greatest CO2 increase from 17.5 until 11.5 kyr before the present, the rate of decline in the concentration of 14C in atmospheric CO2 is greatest and averages about 35 ‰ kyr−1 (Fig. 3; cf. Broecker and Barker, 2007). With the release of 500 Pg C (radiocarbon-dead) to the atmosphere over deglaciation, a drop in 100 ‰ to 200 ‰ Δ14C of atmospheric CO2 can be expected (see calculations by Zeng, 2007). Kerogen oxidation would also release isotopically light carbon to the atmosphere, which is also consistent with the atmospheric record (Fig. 3; Bauska et al., 2016). Finally, driven by orbital forcing (Hays et al., 1976), the response in atmospheric CO2 faithfully echoing increasing global temperatures and diminishing glaciated terrain (Sigman and Boyle, 2000; Stips et al., 2016) goes hand in hand with continuous aerial exposure and enhanced oxidation of finely ground, reactive kerogen (Fig. 2).
Kerogen exposed on Earth's surface is distributed unevenly, with kerogen-rich surface lithologies extending across much of western Canada (see Fig. 2 in Copard et al., 2007). These areas experienced dramatic deglaciation across the 17.5–11.5 kyr BP timeframe (Fig. 4; Dyke, 2004; Dalton et al., 2020). In addition to the widespread occurrence and high abundance of rock-disseminated forms of kerogen, there are also notable surficial occurrences of hydrocarbon-rich lithologies including coal and oil sands within the deglaciated terrains of western Canada. Within the province of Alberta, Andriashek and Pawlowicz (2002) report the widespread occurrence of reworked shale and bitumen in Quaternary till stemming from Cretaceous shales and the oil sands, with unoxidized and oxidized forms present, and the latter enhanced by aerial exposure across paleosurfaces. Today, bituminous erratic boulders are found strewn around the region over tens of thousands of square kilometers (Rutherford, 1928; Andriashek, 2018). As Andriashek (2018) points out, Rutherford (1928) states “There are perhaps many more occurrences of bituminous sand within the glacial deposits of Alberta, but since they weather comparatively readily and become covered with soil, they are not likely to be detected unless by accident, …”. This observation of rapid weathering of bitumen indicates that today's occurrences in glacial tills represent the tip of the iceberg of what once was present. Laboratory incubations simulating CO2 respiration from bituminous materials reveal fluxes that are markedly higher than those associated with oxidation of rock-disseminated forms of kerogen (see Table 2). Chang and Berner (1998, 1999) report subaquatic bituminous coal oxidation (calculated > 300 ) releasing CO2 at rates 1–2 orders of magnitude higher than those reported for rock-disseminated kerogen and 3 orders of magnitude greater than the average for Earth's surface. Microbes likely play key roles in assimilating and releasing this ancient organic carbon as CO2 to the atmosphere (ZoBell, 1946; Hemingway et al., 2018) with microbial communities on the surface colonizing oil-sand-derived bitumen under both summer and winter conditions (Wyndham and Costerton, 1981; Wong et al., 2015). Biodegradation is accelerated during summer months when temperatures of subaerially exposed outcrops of oil sands reach 60 ∘C (Wong et al., 2015). Field experiments show a 2.2-fold increase in kerogen-derived CO2 with a 10 ∘C increase (Soulet et al., 2021). Microbial degradation experiments on bitumen (Ait-Langomazino et al., 1991) reveal an even greater CO2 release when extrapolated to natural systems (e.g., oil sands, see Table 2) exceeding 18 , over 100 times greater than the highest CO2 flux released by rock-disseminated kerogen oxidation. Other laboratory-based studies (e.g., Uribe-Alvarez et al., 2011) that investigated the oxidative decay of hydrocarbon fractions also suggest similarly high fluxes when scaled to natural systems, even though these studies were conducted over periods of only a few weeks. Such observations likely overestimate long-term natural fluxes over multiple years and decades, while the fluxes reported by Chang and Berner (1998, 1999) likely represent an underestimate due to sample storage for several years prior to analysis and the absence of microbial activity under their experimental conditions. Additionally, comparable quantities of kerogen-derived CO2 can also be released under anaerobic conditions, which may become relevant under warming glaciers (e.g., Bertassoli et al., 2016; Rogieri Pelissari et al., 2021; see also Sharp et al., 1999; Sharp and Tranter, 2017). Overall, it is conceivable that super carbon source terrains (hypothetically, areas laced or covered with coal, bituminous materials, etc.) across western Canada could supply an overproportionate quantity of radiocarbon-dead CO2 to the atmosphere during glacial–interglacial transitions.
By 11.5 kyr before the present, the pronounced rise in atmospheric CO2 had subsided. By this time, the Laurentide Ice Sheet had retreated beyond the easternmost reaches of the Western Canadian Sedimentary Basin and was exposing bedrock from the relatively kerogen-poor, highly metamorphosed Canadian Shield (Fig. 4; Dalton et al., 2020). The timing of the inflection point in the rise of CO2 occurs ≤ 300 years after the entire western edge of the Laurentide Ice Sheet advanced onto the Canadian Shield suggests diminishing decay of kerogen and relaxation of the landscape. By this time, the rate of soil organic carbon sequestration in these paraglacial landscapes begins to pick up substantially (Harden et al., 1992). Lithologies of the Fennoscandian Shield and most of its adjacent landmasses (deglaciated eastern and southern sectors of the Fennoscandian Ice Sheet; see Stroeven et al., 2016 and Copard et al., 2007) contain relatively low kerogen contents, therefore limiting its contribution to atmospheric CO2 rise in the wake of Fennoscandian Ice Sheet retreat. Glacially ground graphite shed from these highly metamorphosed rocks is chemically recalcitrant and is mostly redeposited (Sauramo, 1938; Sackett et al., 1974; Sparkes et al., 2020). Geophysical arguments propose that the relatively narrow timing of the Laurentide Ice Sheet's isochrons retreating into the Canadian Shield is more than coincidence: the lithological base and its overlying glacial till influence the dynamics of ice sheet retreat, with the Western Canadian Sedimentary Basin representing a deformable base while the Canadian Shield behaves rigidly, providing an explanation for why the geological and ice sheet isochron maps show similarities (Clark, 1994 and see Fig. 3 therein; Licciardi et al., 1998). At the same time in the context of the kerogen oxidation hypothesis, this offers an explanation for the well-defined inflection point in the rise of atmospheric CO2 characteristic for glacial–interglacial transitions.
In the bigger picture, during Earth's recent glacial episodes, the Laurentide Ice Sheet was the most extensive element of the cryosphere that waxed and waned across the continents (Batchelor et al., 2019) and, in conjunction with its lithological underpinning (Copard et al., 2007), likely played the largest role in releasing kerogen-derived CO2 to the atmosphere upon glacial retreat. However, estimates of CO2 fluxes emanating from bedrock show a wide range (Table 2), and there is considerable uncertainty in our current state of knowledge: dedicated biogeochemical weathering studies that provide estimates of CO2 fluxes from bedrock-derived kerogen under relevant environmental conditions and over appropriate timescales are lacking. Chronosequence studies of kerogen oxidation rates in deglaciated terrains are needed to provide constraints on time-integrated CO2 release to the atmosphere. In tandem with this, quantification is needed for (temporary) kerogen reburial in subaerial and subaquatic terrestrial systems (e.g., moraines, lakes) on global and regional scales (e.g., Meybeck, 1993; Vonk et al., 2016; Blattmann et al., 2019b; Fox et al., 2020). Such information in conjunction with high-resolution reconstructions of changes in land ice extent and the lithologies of bedrock and glacial till (including data on kerogen content and its stable carbon isotope composition) exposed by glacial retreat can, in theory, quantitatively disentangle the time-integrated contribution of kerogen-derived CO2 to the atmosphere during glacial–interglacial transitions.
While modern-day kerogen weathering and reburial efficiencies are only loosely constrained, even less is known about how they varied back in geologic time. Overarching controls on these processes include the mode of erosion and transport ranging from glacial to glaciofluvial to fluvial, remineralization intensity, controlled by continental margin type and geomorphology, and the intrinsic reactivity of the kerogen present locally (Blair and Aller, 2012; Blattmann et al., 2018b). Also important are the bedrock lithology and regolith composition, which have been hypothesized to exert feedback on temporal patterns of glacial–interglacial cyclicity (e.g., Roy et al., 2004; Zeng, 2007). For the Paleocene–Eocene Thermal Maximum, an extreme greenhouse episode, evidence increasingly suggests that enhanced remineralization and leaching of kerogen (Boucsein and Stein, 2009; Lyons et al., 2019), possibly enhanced by the activity of microbes (Hemingway et al., 2018; Petsch et al., 2001), increased the flux of carbon entering actively circulating pools on Earth's surface. Over Earth's history on 109-year timescales, the reburial efficiency of kerogen presumably varied as a function of atmospheric O2 content, with lower O2 contents tied to higher reburial efficiency (Daines et al., 2017). Kerogen is surmised to have acted as a major source of carbon to the atmosphere and as an “antioxidant” during the early rise of atmospheric O2 (Daines et al., 2017; Kump et al., 2011) and balancing O2 levels over the Cenozoic (Galvez, 2020; see also Derry and France-Lanord, 1996, for discussion on the Paleogene). Across multiple glacial–interglacial cycles, enhanced kerogen oxidation would also be consistent with declining atmospheric O2 on 106-year timescales (Stolper et al., 2016).
In order to understand changes in atmospheric chemistry through geologic time, in addition to comprehensively budgeting the effect of mineral chemical weathering (Blattmann et al., 2019a; Horan et al., 2019; Hilton et al., 2014), the changing efficiency of the oxidation and reburial of kerogen needs to be evaluated. Direct quantification of kerogen found reburied in sediments is often associated with considerable uncertainty owing to uncertainties in organic matter source apportionment (e.g., Lin et al., 2020; Blattmann et al., 2019b) and uneven spatial dispersal (e.g., Berg et al., 2021; Blattmann et al., 2018a; Cui et al., 2016). While radiocarbon was paramount for quantifying and establishing the importance of the reburial of kerogen in recent times (Blattmann et al., 2018b), its utility diminishes quickly for strata that predate the Last Glacial Maximum owing to its radioactive decay. However, associated with kerogen are a promising suite of trace elements and their respective isotope signatures including, among others, rhenium (Hilton et al., 2014; Horan et al., 2017), osmium (Georg et al., 2013; Ravizza and Esser, 1993), and iodine (Moran et al., 1998) as well as clay minerals (Blattmann and Liu, 2021), which can be exploited to trace sedimentary kerogen and its degradation. In the case of osmium, seawater records reveal isotopic shifts at the beginning of interglacial periods that are attributable to kerogen oxidation (Georg et al., 2013; compare discussions in Peucker-Ehrenbrink and Ravizza, 2020), consistent with the hypothesis presented here. More research constraining the exogenous kerogen cycle by quantification of reburied kerogen inputs (e.g., iodine isotopes, organic petrology) and kerogen oxidation recorded by chemical weathering proxies (e.g., osmium isotopes) is needed to put the presented hypothesis to the test.
In a nutshell, the hypothesis presented proposes the following: less than 300 years after the Laurentide Ice Sheet retreated east past the easternmost edges of the kerogen-rich Western Canadian Sedimentary Basin, atmospheric CO2 levels stabilized and the rate of decrease in 14C concentrations of CO2 subsided (Figs. 3 and 4). This inflection point is mirrored in the lithologies of the Canadian Shield that were exposed during deglaciation, which contain relatively minor amounts of reactive kerogen. Within the context of the presented hypothesis, the coincidence in time of global trends in atmospheric chemistry with spatiotemporal patterns in the distribution of freshly exposed deglaciated terrain impregnated with oxidizable and biodegradable kerogen and bituminous materials suggests that a burst (or bursts) of respired CO2 contributed to the characteristic deglacial increase in atmospheric CO2. As soon as glacially ground shales and bituminous materials exhausted their labile kerogen content and/or became buried by soil and vegetation taking hold on the deglaciated landscape, classically considered processes (land–ocean exchange, biospheric uptake, etc.) reassumed dominant control on fluctuations of atmospheric chemistry. As a corollary of this hypothesis: CO2 rise within the envelope of glacial–interglacial cyclicity would primarily have responded as a slave to orbitally controlled glacial retreat across kerogen-impregnated landscapes as a result of global temperature increase. CO2 rise may therefore lead or lag global temperature depending on the spatiotemporal patterns of glacial retreat that exposes glacially ground, kerogen-rich or even bituminous parent material.
However, in tandem with hypothesized kerogen oxidation, Earth system constraints such as the carbon isotope record dictate that other major processes must have acted. Various marine mechanisms have been proposed to explain CO2 increases at glacial–interglacial transitions including the solubility pump hypothesis, iron fertilization hypothesis, and ocean circulation hypotheses (see hypotheses and reviews by Martin, 1990; Broecker and Peng, 1993; Kohfeld and Ridgwell, 2009; Rapp, 2019). As a cogwheel operating under manifold feedbacks in the greater Earth system (Sigman and Boyle, 2000), continuous glacial retreat and the oxidation of finely ground kerogen provide a hypothesis consistent with contributing to CO2 increase in the wake of glacial episodes. This dilution of radiocarbon-dead CO2 in the atmosphere may well have been complemented by other terrestrial sources such as the oxidation of subglacial paleosols and permafrost-bound organic carbon (Zeng, 2007; Simmons et al., 2016; Tesi et al., 2016; Crichton et al., 2016; Martens et al., 2020; Winterfeld et al., 2018; Lindgren et al., 2018; Köhler et al., 2014; Ciais et al., 2012) and by volcanic emissions triggered by deglacial unloading of the lithosphere (Roth and Joos, 2012). In addition to studies of weathering in glacial forefields and source-to-sink tracing of sedimentary kerogen, several lines of geochemical evidence including atmospheric carbon isotope composition (13C and 14C), which have thus far received contorted, partial explanations (Broecker and Clark, 2010; Schmitt et al., 2012; Broecker and McGee, 2013), glacial–interglacial changes in 14C of DIC in seawater (Rafter et al., 2019; cf. discussions therein), seawater osmium isotope changes, and long-term atmospheric O2 content, conceptually go hand in hand with an opening of the exogenous kerogen cycle modulated by glacial activity. While geomagnetic variability and ocean ventilation together struggle to fully explain observed changes in atmospheric radiocarbon (Broecker and Barker, 2007; Cheng et al., 2018), the dilution of atmospheric CO2 by accelerated oxidation of ancient terrestrial organic carbon at glacial terminations, in conjunction with other mechanisms including atmosphere–ocean gas exchange (e.g., Sigman et al., 2010; Marcott et al., 2014; Menviel et al., 2018; Martin, 1990; Sarntheim et al., 2013; Ai et al., 2020), appears as a simple and plausible explanation. In addition to kerogen oxidation at glacial terminations, the hypothesis presented carries other important implications, including the closure of the exogenous kerogen cycle during glacial periods (Fig. 2) potentially contributing to relatively high atmospheric 14C signatures, a problem highlighted by Dinauer et al. (2020), as a reduced 14C-free CO2 flux would reduce the dilution of the atmosphere's cosmogenic 14C. Overall, increased reburial efficiency of kerogen can account for several tens of petagrams of carbon over millennial timescales, entirely bypassing actively circulating carbon pools on Earth's surface (Fig. 1). In contrast, increased oxidation efficiency of kerogen in the wake of glacial episodes that have built up stores of finely ground reactive substrate and exposed fresh weathering profiles can account for several hundreds of petagrams of carbon over millennial timescales released into actively circulating pools. Owing to increased bedrock exhumation over the ice ages (Herman et al., 2013, 2015), the dynamism of the exogenous kerogen cycle may have been intensified, with greater fluxes of detrital kerogen reburied in ocean sediments during glacial episodes and increased supplies of ground kerogen exposed to the elements in their wake.
While basic controls on kerogen reburial efficiency have emerged, its quantitative impact on atmospheric chemistry through geologic time remains conjectural. Mathematically analogous modeling results (Zeng, 2003; Simmons et al., 2016) suggest that kerogen oxidation could account for a 30–60 ppm rise in atmospheric CO2 over the course of the last deglaciation. Aligning with this, the back-of-the-envelope mass balance calculations presented in Table 1 suggest CO2 release from kerogen and the ocean each contributed around half to the total increase in atmospheric and terrestrial biospheric carbon pool sizes. However, there is a lack of studies on kerogen weathering to provide sufficient quantitative constraint for testing this hypothesis. To work towards such a test, investigating kinetics of kerogen oxidation along glacial chronosequences with contrasting lithologies would provide numerical input for Earth system models. Such information in conjunction with spatiotemporal changes in land ice extent (e.g., Dalton et al., 2020; Stroeven et al., 2016) integrating over areal changes of glacially exposed lithological units would constrain the quantitative impact of the exogenous kerogen cycle on atmospheric chemistry over glacial–interglacial cycles (e.g., see Fig. 2 in Kohfeld and Ridgwell, 2009). Additionally, the role of super carbon source terrains (e.g., surficial oil sands and coal) deserves special attention as biogeochemical weathering and associated CO2 fluxes emanating from such areas conceivably contribute disproportionately, particularly upon deglaciation. Overall, the spatiotemporal deglaciation of contrasting source terrains across North America with the coeval progression of atmospheric chemistry provides a strong incentive to explore the potential role of the exogenous kerogen cycle in glacial–interglacial patterns. From a greenhouse perspective, further study of pivotal episodes such as the Paleocene–Eocene Thermal Maximum under this lens may provide geological outlooks that are relevant today. In the context of our warming world, once critical thresholds are breached (Steffen et al., 2018), enhanced opening of the exogenous kerogen cycle may entrain the Earth system onto a new trajectory influencing the carbon cycle and climate for millennia to come.
The data used in this paper are available upon request.
The supplement related to this article is available online at: https://doi.org/10.5194/bg-19-359-2022-supplement.
The contact author has declared that there are no competing interests.
Publisher's note: Copernicus Publications remains neutral with regard to jurisdictional claims in published maps and institutional affiliations.
This work greatly benefitted from the input of several anonymous reviewers. The author is thankful for discussions with Timothy Eglinton, Dominik Letsch, Maarten Lupker, Jesper Suhrhoff, Valier Galy, Naohiko Ohkouchi, and Robert Hilton. This work was supported by funding from JAMSTEC.
This research has been supported by the Japan Agency for Marine-Earth Science and Technology.
This paper was edited by Markus Kienast and reviewed by four anonymous referees.
Adams, J. M., Faure, H., Faure-Denard, L., McGlade, J. M., and Woodward, F. I.: Increases in terrestrial carbon storage from the Last Glacial Maximum to the present, Nature, 348, 711–714, 1990.
Ai, X. E., Studer, A. S., Sigman, D. M., Martínez-García, A., Fripiat, F., Thöle, L. M., Michel, E., Gottschalk, J., Arnold, L., Moretti, S., Schmitt, M., Oleynik, S., Jaccard, S. L., and Haug, G. H.: Southern Ocean upwelling, Earth's obliquity, and glacial-interglacial atmospheric CO2 change, Science, 370, 1348–1352, https://doi.org/10.1126/science.abd2115, 2020.
Ait-Langomazino, N., Sellier, R., Jouquet, G., and Trescinski, M.: Microbial degradation of bitumen, Experientia, 47, 533–539, 1991.
Andriashek, L. D.: On the origin of oil sand and related bedrock erratics in glacial sediments of central Alberta; Alberta Energy Regulator/Alberta Geological Survey, AER/AGS Open File Report 2018–13, 42 p., 2018.
Andriashek, L. D. and Pawlowicz, J. G.: Observations of naturally occurring hydrocarbons (bitumen) in Quaternary sediments, Athabasca Oil Sands area and areas west, Alberta, Alberta Energy Regulator/Alberta Geological Survey, Edmonton, Alberta, Canada, EUB/AGS Geo-Note 2002-01, 27 p., 2002.
Barnola, J. M., Raynaud, D., Korotkevich, Y. S., and Lorius, C.: Vostok ice core provides 160,000-year record of atmospheric CO2, Nature, 329, 408–414, 1987.
Batchelor, C. L., Margold, M., Krapp, M., Murton, D. K., Dalton, A. S., Gibbard, P. L., Stokes, C. R., Murton, J. B., and Manica, A.: The configuration of Northern Hemisphere ice sheets through the Quaternary, Nat. Commun., 10, 3713, https://doi.org/10.1038/s41467-019-11601-2, 2019.
Bauska, T. K., Baggenstos, D., Brook, E. J., Mix, A. C., Marcott, S. A., Petrenko, V. V., Schaefer, H., Severinghaus, J. P., and Lee, J. E.: Carbon isotopes characterize rapid changes in atmospheric carbon dioxide during the last deglaciation, P. Natl. Acad. Sci. USA, 113, 3465–3470, 2016.
Beaupré, S. R.: The carbon isotopic composition of marine DOC, in: Biogeochemistry of Marine Dissolved Organic Matter, second edn., edited by: Hansell, D. A. and Carlson, C. A., Academic Press, Boston, 335–368, 2015.
Berg, S., Jivcov, S., Kusch, S., Kuhn, G., White, D., Bohrmann, G., Melles, M., and Rethemeyer, J.: Increased petrogenic and biospheric organic carbon burial in sub-Antarctic fjord sediments in response to recent glacier retreat, Limnol. Oceanogr., 66, 4347–4362, https://doi.org/10.1002/lno.11965, 2021.
Berner, R. A.: Atmospheric carbon dioxide levels over Phanerozoic time, Science, 249, 1382–1386, 1990.
Berner, R. A., Lasaga, A. C., and Garrels, R. M.: The carbonate-silicate geochemical cycle and its effect on atmospheric carbon dioxide over the past 100 million years, Am. J. Sci., 283, 641–683, https://doi.org/10.2475/ajs.283.7.641, 1983.
Bertassoli Jr., D. J., Sawakuchi, H. O., Almeida, N. S., Castanheira, B., Alem, V. A. T., Camargo, M. G. P., Krusche, A. V., Brochsztain, S., and Sawakuchi, A. O.: Biogenic methane and carbon dioxide generation in organic-rich shales from southeastern Brazil, Int. J. Coal Geol., 162, 1–13, 2016.
Bird, M. I., Lloyd, J., and Farquhar, G. D.: Terrestrial carbon storage at the LGM, Nature, 371, 566–566, 1994.
Blair, N. E. and Aller, R. C.: The fate of terrestrial organic carbon in the marine environment, Annu. Rev. Mar. Sci., 4, 401–423, 2012.
Blattmann, T. M. and Liu, Z.: Proposing a classic clay mineral proxy for quantifying kerogen reburial in the geologic past, Appl. Clay Sci., 211, https://doi.org/10.1016/j.clay.2021.106190, 2021.
Blattmann, T. M., Zhang, Y., Zhao, Y., Wen, K., Lin, S., Li, J., Wacker, L., Haghipour, N., Plötze, M., Liu, Z., and Eglinton, T. I.: Contrasting fates of petrogenic and biospheric carbon in the South China Sea, Geophys. Res. Lett., 45, 9077–9086, 2018a.
Blattmann, T. M., Letsch, D., and Eglinton, T. I.: On the geological and scientific legacy of petrogenic organic carbon, Am. J. Sci., 318, 861–881, 2018b.
Blattmann, T. M., Wang, S. L., Lupker, M., Märki, L., Haghipour, N., Wacker, L., Chung, L. H., Bernasconi, S. M., Plötze, M., and Eglinton, T. I.: Sulphuric acid-mediated weathering on Taiwan buffers geological atmospheric carbon sinks, Sci. Rep.-UK, 9, 2945, https://doi.org/10.1038/s41598-019-39272-5, 2019a.
Blattmann, T. M., Wessels, M., McIntyre, C. P., and Eglinton, T. I.: Petrogenic organic carbon retention in terrestrial basins: A case study from perialpine Lake Constance, Chem. Geol., 503, 52–60, 2019b.
Bolton, E. W., Berner, R. A., and Petsch, S. T.: The weathering of sedimentary organic matter as a control on atmospheric O2: II. Theoretical modelling, Am. J. Sci., 306, 575–615, 2006.
Bouchez, J., Beyssac, O., Galy, V., Gaillardet, J., France-Lanord, C., Maurice, L., and Moreira-Turcq, P.: Oxidation of petrogenic organic carbon in the Amazon floodplain as a source of atmospheric CO2, Geology, 38, 255–258, 2010.
Boucsein, B. and Stein, R.: Black shale formation in the late Paleocene/early Eocene Arctic Ocean and paleoenvironmental conditions: New results from a detailed organic petrological study, Mar. Petrol. Geol., 26, 416–426, 2009.
Bouttes, N., Vazquez Riveiros, N., Govin, A., Swingedouw, D., Sanchez-Goni, M. F., Crosta, X., and Roche, D. M.: Carbon 13 isotopes reveal limited ocean circulation changes between interglacials of the last 800 ka, Paleoceanogr. Paleoclimatol., 35, https://doi.org/10.1029/2019PA003776, 2020.
Broecker, W. and Barker, S.: A 190 ‰ drop in atmosphere's Δ14C during the “Mystery Interval” (17.5 to 14.5 kyr), Earth Planet. Sc. Lett., 256, 90–99, 2007.
Broecker, W. and Clark, E.: Search for a glacial-age 14C-depleted ocean reservoir, Geophys. Res. Lett., 37, https://doi.org/10.1029/2010GL043969, 2010.
Broecker, W. S. and McGee, D.: The 13C record for atmospheric CO2: What is it trying to tell us?, Earth Planet. Sc. Lett., 368, 175–182, 2013.
Broecker, W. S. and Peng, T. H.: What caused the glacial to interglacial CO2 change?, in: The Global Carbon Cycle, edited by: Heimann, M., Springer Berlin Heidelberg, Berlin, Heidelberg, 95–115, 1993.
Bufe, A., Hovius, N., Emberson, R., Rugenstein, J. K. C., Galy, A., Hassenruck-Gudipati, H. J., and Chang, J.-M.: Co-variation of silicate, carbonate and sulfide weathering drives CO2 release with erosion, Nat. Geosci., 14, 211–216, 2021.
Chang, S. and Berner, R. A.: Humic substance formation via the oxidative weathering of coal, Environ. Sci. Technol., 32, 2883–2886, 1998.
Chang, S. and Berner, R. A.: Coal weathering and the geochemical carbon cycle, Geochim. Cosmochim. Ac., 63, 3301–3310, 1999.
Cheng, H., Edwards, R. L., Southon, J., Matsumoto, K., Feinberg, J. M., Sinha, A., Zhou, W., Li, H., Li, X., Xu, Y., Chen, S., Tan, M., Wang, Q., Wang, Y., and Ning, Y.: Atmospheric 14C/12C changes during the last glacial period from Hulu Cave, Science, 362, 1293–1297, 2018.
Ciais, P., Tagliabue, A., Cuntz, M., Bopp, L., Scholze, M., Hoffmann, G., Lourantou, A., Harrison, S. P., Prentice, I. C., Kelley, D. I., Koven, C., and Piao, S. L.: Large inert carbon pool in the terrestrial biosphere during the Last Glacial Maximum, Nat. Geosci., 5, 74–79, 2012.
Clark, K. E., Hilton, R. G., West, A. J., Robles Caceres, A., Gröcke, D. R., Marthews, T. R., Ferguson, R. I., Asner, G. P., New, M., and Malhi, Y.: Erosion of organic carbon from the Andes and its effects on ecosystem carbon dioxide balance, J. Geophys. Res.-Biogeo., 122, 449–469, 2017.
Clark, P. U.: Unstable behavior of the Laurentide Ice Sheet over deforming sediment and its implications for climate change, Quaternary Res., 41, 19–25, 1994.
Copard, Y., Amiotte-Suchet, P., and Di-Giovanni, C.: Storage and release of fossil organic carbon related to weathering of sedimentary rocks, Earth Planet. Sc. Lett., 258, 345–357, 2007.
Crichton, K. A., Bouttes, N., Roche, D. M., Chappellaz, J., and Krinner, G.: Permafrost carbon as a missing link to explain CO2 changes during the last deglaciation, Nat. Geosci., 9, 683–686, 2016.
Crowley, T. J.: Ice Age terrestrial carbon changes revisited, Global Biogeochem. Cy., 9, 377–389, 1995.
Cui, X., Bianchi, T. S., Jaeger, J. M., and Smith, R. W.: Biospheric and petrogenic organic carbon flux along southeast Alaska, Earth Planet. Sc. Lett., 452, 238–246, 2016.
Daines, S. J., Mills, B. J. W., and Lenton, T. M.: Atmospheric oxygen regulation at low Proterozoic levels by incomplete oxidative weathering of sedimentary organic carbon, Nat. Commun., 8, 14379, https://doi.org/10.1038/ncomms14379, 2017.
Dalton, A. S., Margold, M., Stokes, C. R., Tarasov, L., Dyke, A. S., Adams, R. S., Allard, S., Arends, H. E., Atkinson, N., Attig, J. W., Barnett, P. J., Barnett, R. L., Batterson, M., Bernatchez, P., Borns, H. W., Breckenridge, A., Briner, J. P., Brouard, E., Campbell, J. E., Carlson, A. E., Clague, J. J., Curry, B. B., Daigneault, R.-A., Dubé-Loubert, H., Easterbrook, D. J., Franzi, D. A., Friedrich, H. G., Funder, S., Gauthier, M. S., Gowan, A. S., Harris, K. L., Hétu, B., Hooyer, T. S., Jennings, C. E., Johnson, M. D., Kehew, A. E., Kelley, S. E., Kerr, D., King, E. L., Kjeldsen, K. K., Knaeble, A. R., Lajeunesse, P., Lakeman, T. R., Lamothe, M., Larson, P., Lavoie, M., Loope, H. M., Lowell, T. V., Lusardi, B. A., Manz, L., McMartin, I., Nixon, F. C., Occhietti, S., Parkhill, M. A., Piper, D. J. W., Pronk, A. G., Richard, P. J. H., Ridge, J. C., Ross, M., Roy, M., Seaman, A., Shaw, J., Stea, R. R., Teller, J. T., Thompson, W. B., Thorleifson, L. H., Utting, D. J., Veillette, J. J., Ward, B. C., Weddle, T. K., and Wright, H. E.: An updated radiocarbon-based ice margin chronology for the last deglaciation of the North American Ice Sheet Complex, Quaternary Sci. Rev., 234, 106223, https://doi.org/10.1016/j.quascirev.2020.106223, 2020.
de Carvalho Ferreira, M. L. and Kerr, R.: Source water distribution and quantification of North Atlantic Deep Water and Antarctic Bottom Water in the Atlantic Ocean, Prog. Oceanogr., 153, 66–83, 2017.
Derry, L. A. and France-Lanord, C.: Neogene growth of the sedimentary organic carbon reservoir, Paleoceanography, 11, 267–275, 1996.
Dinauer, A., Adolphi, F., and Joos, F.: Mysteriously high Δ14C of the glacial atmosphere: influence of 14C production and carbon cycle changes, Clim. Past, 16, 1159–1185, https://doi.org/10.5194/cp-16-1159-2020, 2020.
Dyke, A. S.: An outline of North American deglaciation with emphasis on central and northern Canada, in: Developments in Quaternary Sciences, edited by: Ehlers, J. and Gibbard, P. L., Elsevier, 373–424, https://doi.org/10.1016/S1571-0866(04)80209-4, 2004.
Fischer, C. and Gaupp, R.: Change of black shale organic material surface area during oxidative weathering: Implications for rock-water surface evolution, Geochim. Cosmochim. Ac., 69, 1213–1224, 2005.
Fischer, C., Karius, V., and Thiel, V.: Organic matter in black slate shows oxidative degradation within only a few decades, J. Sediment. Res., 77, 355–365, 2007.
Fox, P. M., Bill, M., Heckman, K., Conrad, M., Anderson, C., Keiluweit, M., and Nico, P. S.: Shale as a source of organic carbon in floodplain sediments of a mountainous watershed, J. Geophys. Res.-Biogeo., 125, https://doi.org/10.1029/2019JG005419, 2020.
Galvez, M. E.: Redox constraints on a Cenozoic imbalance in the organic carbon cycle, Am. J. Sci., 320, 730–751, https://doi.org/10.2475/10.2020.03, 2020.
Galy, V., Beyssac, O., France-Lanord, C., and Eglinton, T. I.: Recycling of graphite during Himalayan erosion: A geological stabilization of carbon in the crust, Science, 322, 943–945, 2008.
Galy, V., Peucker-Ehrenbrink, B., and Eglinton, T. I.: Global carbon export from the terrestrial biosphere controlled by erosion, Nature, 521, 204–207, 2015.
Georg, R. B., West, A. J., Vance, D., Newman, K., and Halliday, A. N.: Is the marine osmium isotope record a probe for CO2 release from sedimentary rocks?, Earth Planet. Sc. Lett., 367, 28–38, 2013.
Gibbs, M. and Kump, L.: Global chemical weathering during deglaciation, in: Fourth International Symposium on the Geochemistry of the Earth's Surface, University of Leeds, 22–28 July 1996, Ilkley, Yorkshire, England, 733–737, 1996.
Hain, M. P., Sigman, D. M., and Haug, G. H.: Distinct roles of the Southern Ocean and North Atlantic in the deglacial atmospheric radiocarbon decline, Earth Planet. Sc. Lett., 394, 198–208, 2014.
Harden, J. W., Mark, R. K., Sundquist, E. T., and Stallard, R. F.: Dynamics of soil carbon during deglaciation of the Laurentide Ice Sheet, Science, 258, 1921–1924, https://doi.org/10.1126/science.258.5090.1921, 1992.
Hays, J. D., Imbrie, J., and Shackleton, N. J.: Variations in the Earth's orbit: Pacemaker of the Ice Ages, Science, 194, 1121–1132, 1976.
Hedges, J. I. and Oades, J. M.: Comparative organic geochemistries of soils and marine sediments, Org. Geochem., 27, 319–361, 1997.
Herman, F., Seward, D., Valla, P. G., Carter, A., Kohn, B., Willett, S. D., and Ehlers, T. A.: Worldwide acceleration of mountain erosion under a cooling climate, Nature, 504, 423–426, 2013.
Herman, F., Beyssac, O., Brughelli, M., Lane, S. N., Leprince, S., Adatte, T., Lin, J. Y. Y., Avouac, J.-P., and Cox, S. C.: Erosion by an Alpine glacier, Science, 350, 193–195, 2015.
Hemingway, J. D., Hilton, R. G., Hovius, N., Eglinton, T. I., Haghipour, N., Wacker, L., Chen, M.-C., and Galy, V. V.: Microbial oxidation of lithospheric organic carbon in rapidly eroding tropical mountain soils, Science, 360, 209–212, https://doi.org/10.1126/science.aao6463, 2018.
Hilton, R. G. and West, A. J.: Mountains, erosion and the carbon cycle, Nat. Rev. Earth Environ., 1, 284–299, 2020.
Hilton, R. G., Galy, A., Hovius, N., Horng, M.-J., and Chen, H.: Efficient transport of fossil organic carbon to the ocean by steep mountain rivers: An orogenic carbon sequestration mechanism, Geology, 39, 71–74, 2011.
Hilton, R. G., Gaillardet, J., Calmels, D., and Birck, J.-L.: Geological respiration of a mountain belt revealed by the trace element rhenium, Earth Planet. Sc. Lett., 403, 27–36, 2014.
Hood, E., Fellman, J., Spencer, R. G. M., Hernes, P. J., Edwards, R., D'Amore, D., and Scott, D.: Glaciers as a source of ancient and labile organic matter to the marine environment, Nature, 462, 1044–1047, https://doi.org/10.1038/nature08580, 2009.
Horan, K.: The oxidative weathering of organic matter and its carbon dioxide emissions: Insight from the trace elements rhenium and molybdenum, PhD thesis, Durham University, UK, 223 pp., 2018.
Horan, K., Hilton, R. G., Selby, D., Ottley, C. J., Gröcke, D. R., Hicks, M., and Burton, K. W.: Mountain glaciation drives rapid oxidation of rock-bound organic carbon, Sci. Adv., 3, https://doi.org/10.1126/sciadv.1701107, 2017.
Horan, K., Hilton, R. G., Dellinger, M., Tipper, E., Galy, V., Calmels, D., Selby, D., Gaillardet, J., Ottley, C. J., Parsons, D. R., and Burton, K. W.: Carbon dioxide emissions by rock organic carbon oxidation and the net geochemical carbon budget of the Mackenzie River Basin, Am. J. Sci., 319, 473–499, 2019.
Huang, Y., Street-Perrott, F. A., Metcalfe, S. E., Brenner, M., Moreland, M., and Freeman, K. H.: Climate change as the dominant control on glacial-interglacial variations in C3 and C4 plant abundance, Science, 293, 1647–1651, 2001.
Jeltsch-Thömmes, A., Battaglia, G., Cartapanis, O., Jaccard, S. L., and Joos, F.: Low terrestrial carbon storage at the Last Glacial Maximum: constraints from multi-proxy data, Clim. Past, 15, 849–879, https://doi.org/10.5194/cp-15-849-2019, 2019.
Keller, C. K. and Bacon, D. H.: Soil respiration and georespiration distinguished by transport analyses of vadose CO2, 13CO2, and 14CO2, Global Biogeochem. Cy., 12, 361–372, 1998.
Kohfeld, K. E. and Ridgwell, A.: Glacial-interglacial variability in atmospheric CO2, in: Surface Ocean–Lower Atmosphere Processes, edited by: Le Quéré, C. and Saltzman, E. S., Geophys. Monogr. Ser., American Geophysical Union, 251–286, 2009.
Köhler, P., Knorr, G., and Bard, E.: Permafrost thawing as a possible source of abrupt carbon release at the onset of the Bølling/Allerød, Nat. Commun., 5, 5520, https://doi.org/10.1038/ncomms6520, 2014.
Kölling, M., Bouimetarhan, I., Bowles, M. W., Felis, T., Goldhammer, T., Hinrichs, K.-U., Schulz, M., and Zabel, M.: Consistent CO2 release by pyrite oxidation on continental shelves prior to glacial terminations, Nat. Geosci., 12, 929–934, 2019.
Kump, L. R., Junium, C., Arthur, M. A., Brasier, A., Fallick, A., Melezhik, V., Lepland, A., Črne, A. E., and Luo, G.: Isotopic evidence for massive oxidation of organic matter following the Great Oxidation Event, Science, 334, 1694–1696, 2011.
Lewan, M. D.: Stable carbon isotopes of amorphous kerogens from Phanerozoic sedimentary rocks, Geochim. Cosmochim. Ac., 50, 1583–1591, 1986.
Leythaeuser, D.: Effects of weathering on organic matter in shales, Geochim. Cosmochim. Ac., 37, 113–120, 1973.
Licciardi, J. M., Clark, P. U., Jenson, J. W., and Macayeal, D. R.: Deglaciation of a soft-bedded Laurentide Ice Sheet, Quaternary Sci. Rev., 17, 427–448, 1998.
Lin, B., Liu, Z., Eglinton, T. I., Kandasamy, S., Blattmann, T. M., Haghipour, N., Huang, K.-F., and You, C.-F.: Island-wide variation in provenance of riverine sedimentary organic carbon: A case study from Taiwan, Earth Planet. Sc. Lett., 539, 116238, https://doi.org/10.1016/j.epsl.2020.116238, 2020.
Lindgren, A., Hugelius, G., and Kuhry, P.: Extensive loss of past permafrost carbon but a net accumulation into present-day soils, Nature, 560, 219–222, 2018.
Littke, R., Klussmann, U., Krooss, B., and Leythaeuser, D.: Quantification of loss of calcite, pyrite, and organic matter due to weathering of Toarcian black shales and effects on kerogen and bitumen characteristics, Geochim. Cosmochim. Ac., 55, 3369–3378, 1991.
Lyons, S. L., Baczynski, A. A., Babila, T. L., Bralower, T. J., Hajek, E. A., Kump, L. R., Polites, E. G., Self-Trail, J. M., Trampush, S. M., Vornlocher, J. R., Zachos, J. C., and Freeman, K. H.: Palaeocene–Eocene Thermal Maximum prolonged by fossil carbon oxidation, Nat. Geosci., 12, 54–60, 2019.
Marcott, S. A., Bauska, T. K., Buizert, C., Steig, E. J., Rosen, J. L., Cuffey, K. M., Fudge, T. J., Severinghaus, J. P., Ahn, J., Kalk, M. L., McConnell, J. R., Sowers, T., Taylor, K. C., White, J. W. C., and Brook, E. J.: Centennial-scale changes in the global carbon cycle during the last deglaciation, Nature, 514, 616–619, https://doi.org/10.1038/nature13799, 2014.
Martens, J., Wild, B., Muschitiello, F., O'Regan, M., Jakobsson, M., Semiletov, I., Dudarev, O. V., and Gustafsson, Ö.: Remobilization of dormant carbon from Siberian-Arctic permafrost during three past warming events, Sci. Adv., 6, https://doi.org/10.1126/sciadv.abb6546, 2020.
Martin, J. H.: Glacial-interglacial CO2 change: The iron hypothesis, Paleoceanography, 5, 1–13, 1990.
Martínez, M., and Escobar, M.: Effect of coal weathering on some geochemical parameters, Org. Geochem., 23, 253–261, 1995.
Menviel, L., Spence, P., Yu, J., Chamberlain, M. A., Matear, R. J., Meissner, K. J., and England, M. H.: Southern Hemisphere westerlies as a driver of the early deglacial atmospheric CO2 rise, Nat. Commun., 9, 2503, https://doi.org/10.1038/s41467-018-04876-4, 2018.
Meybeck, M.: Riverine transport of atmospheric carbon: Sources, global typology and budget, Water Air Soil Pollut., 70, 443–463, 1993.
Meyers, P. A.: Preservation of elemental and isotopic source identification of sedimentary organic matter, Chem. Geol., 114, 289–302, 1994.
Miall, A. D. and Blakey, R. C.: The Phanerozoic tectonic and sedimentary evolution of North America, in: The Sedimentary Basins of the United States and Canada, second edn., edited by: Miall, A. D., Elsevier, 1–38, https://doi.org/10.1016/B978-0-444-63895-3.00001-2, 2019.
Moran, J. E., Fehn, U., and Teng, R. T. D.: Variations in ratios in recent marine sediments: evidence for a fossil organic component, Chem. Geol., 152, 193–203, 1998.
Munhoven, G. and François, L. M.: Glacial-interglacial variability of atmospheric CO2 due to changing continental silicate rock weathering: A model study, J. Geophys. Res.-Atmos., 101, 21423–21437, 1996.
Peterson, C. D., Lisiecki, L. E., and Stern, J. V.: Deglacial whole-ocean δ13C change estimated from 480 benthic foraminiferal records, Paleoceanography, 29, 549–563, 2014.
Petsch, S. T.: Weathering of organic carbon, in: Treatise on Geochemistry, edited by: Holland, H. D. and Turekian, K. K., Elsevier, Oxford, 217–238, 2014.
Petsch, S. T., Eglinton, T. I., and Edwards, K. J.: 14C-dead living biomass: Evidence for microbial assimilation of ancient organic carbon during shale weathering, Science, 292, 1127–1131, 2001.
Peucker-Ehrenbrink, B. and Ravizza, G. E.: Osmium isotope stratigraphy, in: Geologic Time Scale 2020, edited by: Gradstein, F. M., Ogg, J. G., Schmitz, M. D., and Ogg, G. M., Elsevier, 239–257, https://doi.org/10.1016/B978-0-12-824360-2.00008-5, 2020.
Rafter, P. A., Carriquiry, J. D., Herguera, J.-C., Hain, M. P., Solomon, E. A., and Southon, J. R.: Anomalous > 2000-year-old surface ocean radiocarbon age as evidence for deglacial geologic carbon release, Geophys. Res. Lett., 46, 13950–13960, 2019.
Rapp, D.: Ice Ages and Interglacials: Measurements, Interpretation, and Models, 3rd edn., Springer Nature Switzerland, pp. 346, https://doi.org/10.1007/978-3-030-10466-5, 2019.
Ravizza, G. and Esser, B. K.: A possible link between the seawater osmium isotope record and weathering of ancient sedimentary organic matter, Chem. Geol., 107, 255–258, 1993.
Reed Jr., J. C., Wheeler, J. O., and Tucholke, B. E.: Geologic map of North America: Decade of North American geology continental scale map 001, scale , Geological Society of America, Boulder, 2004.
Reimer, P. J., Bard, E., Bayliss, A., Beck, J. W., Blackwell, P. G., Ramsey, C. B., Buck, C. E., Cheng, H., Edwards, R. L., Friedrich, M., Grootes, P. M., Guilderson, T. P., Haflidason, H., Hajdas, I., Hatté, C., Heaton, T. J., Hoffmann, D. L., Hogg, A. G., Hughen, K. A., Kaiser, K. F., Kromer, B., Manning, S. W., Niu, M., Reimer, R. W., Richards, D. A., Scott, E. M., Southon, J. R., Staff, R. A., Turney, C. S. M., and van der Plicht, J.: IntCal13 and Marine13 radiocarbon age calibration curves 0–50,000 years cal BP, Radiocarbon, 55, 1869–1887, 2013.
Rogieri Pelissari, M., Oliveira Sawakuchi, H., Bertassoli Junior, D. J., da Silva Almeida, N., and Oliveira Sawakuchi, A.: Water influence on CH4 and CO2 generation from tar sandstones: Insights from incubation experiments in the Pirambóia Formation, Paraná Basin, J. S. Am. Earth Sci., 106, 103097, https://doi.org/10.1016/j.jsames.2020.103097, 2021.
Roth, R. and Joos, F.: Model limits on the role of volcanic carbon emissions in regulating glacial–interglacial CO2 variations, Earth Planet. Sc. Lett., 329–330, 141–149, 2012.
Roth, R. and Joos, F.: A reconstruction of radiocarbon production and total solar irradiance from the Holocene 14C and CO2 records: implications of data and model uncertainties, Clim. Past, 9, 1879–1909, https://doi.org/10.5194/cp-9-1879-2013, 2013.
Roy, M., Clark, P. U., Raisbeck, G. M., and Yiou, F.: Geochemical constraints on the regolith hypothesis for the middle Pleistocene transition, Earth Planet. Sc. Lett., 227, 281–296, 2004.
Rutherford, R. L.: Two interesting boulders in the glacial deposits of Alberta, J. Geol., 36, 558–563, 1928.
Sackett, W. M., Poag, C. W., and Eadie, B. J.: Kerogen recycling in the Ross Sea, Antarctica, Science, 185, 1045–1047, 1974.
Sarnthein, M., Schneider, B., and Grootes, P. M.: Peak glacial 14C ventilation ages suggest major draw-down of carbon into the abyssal ocean, Clim. Past, 9, 2595–2614, https://doi.org/10.5194/cp-9-2595-2013, 2013.
Sauramo, M. R.: The mode of occurrence of carbon in Quaternary deposits, Suom. Kemistil., 3, 11–16, 1938.
Schachtman, N. S., Roering, J. J., Marshall, J. A., Gavin, D. G., and Granger, D. E.: The interplay between physical and chemical erosion over glacial-interglacial cycles, Geology, 47, 613–616, 2019.
Schillawski, S. and Petsch, S.: Release of biodegradable dissolved organic matter from ancient sedimentary rocks, Global Biogeochem. Cy., 22, https://doi.org/10.1029/2007GB002980, 2008.
Schmitt, J., Schneider, R., Elsig, J., Leuenberger, D., Lourantou, A., Chappellaz, J., Köhler, P., Joos, F., Stocker, T. F., Leuenberger, M., and Fischer, H.: Carbon isotope constraints on the deglacial CO2 rise from ice cores, Science, 336, 711–714, 2012.
Schmittner, A., Bostock, H. C., Cartapanis, O., Curry, W. B., Filipsson, H. L., Galbraith, E. D., Gottschalk, J., Herguera, J. C., Hoogakker, B., Jaccard, S. L., Lisiecki, L. E., Lund, D. C., Martínez-Méndez, G., Lynch-Stieglitz, J., Mackensen, A., Michel, E., Mix, A. C., Oppo, D. W., Peterson, C. D., Repschläger, J., Sikes, E. L., Spero, H. J., and Waelbroeck, C.: Calibration of the carbon isotope composition (δ13C) of benthic foraminifera, Paleoceanography, 32, 512–530, 2017.
Shackleton, N. J.: Carbon-13 in Uvigerina: Tropical rainforest history and the equatorial Pacific carbonate dissolution cycles, in: The Fate of Fossil Fuel CO2 in the Oceans, edited by: Andersen, N. R. and Malahoff, A., Plenum Press, 401–427, ISBN 030635506X, 1977.
Shackleton, N. J., Hall, M. A., Line, J., and Shuxi, C.: Carbon isotope data in core V19-30 confirm reduced carbon dioxide concentration in the ice age atmosphere, Nature, 306, 319–322, 1983.
Sharp, M. and Tranter, M.: Glacier biogeochemistry, Geochem. Perspect., 6, 173–174, 2017.
Sharp, M., Parkes, J., Cragg, B., Fairchild, I. J., Lamb, H., and Tranter, M.: Widespread bacterial populations at glacier beds and their relationship to rock weathering and carbon cycling, Geology, 27, 107–110, 1999.
Sigman, D. M. and Boyle, E. A.: Glacial/interglacial variations in atmospheric carbon dioxide, Nature, 407, 859–869, 2000.
Sigman, D. M., Hain, M. P., and Haug, G. H.: The polar ocean and glacial cycles in atmospheric CO2 concentration, Nature, 466, 47–55, 2010.
Simmons, C. T., Matthews, H. D., and Mysak, L. A.: Deglacial climate, carbon cycle and ocean chemistry changes in response to a terrestrial carbon release, Clim. Dynam., 46, 1287–1299, 2016.
Smith, H. J., Fischer, H., Wahlen, M., Mastroianni, D., and Deck, B.: Dual modes of the carbon cycle since the Last Glacial Maximum, Nature, 400, 248–250, 1999.
Soulet, G., Hilton, R. G., Garnett, M. H., Dellinger, M., Croissant, T., Ogrič, M., and Klotz, S.: Technical note: In situ measurement of flux and isotopic composition of CO2 released during oxidative weathering of sedimentary rocks, Biogeosciences, 15, 4087–4102, https://doi.org/10.5194/bg-15-4087-2018, 2018.
Soulet, G., Hilton, R. G., Garnett, M. H., Roylands, T., Klotz, S., Croissant, T., Dellinger, M., and Le Bouteiller, C.: Temperature control on CO2 emissions from the weathering of sedimentary rocks, Nat. Geosci., 14, 665–671, 2021.
Sparkes, R. B., Hovius, N., Galy, A., and Liu, J. T.: Survival of graphitized petrogenic organic carbon through multiple erosional cycles, Earth Planet. Sc. Lett., 531, 115992, https://doi.org/10.1016/j.epsl.2019.115992, 2020.
Steffen, W., Rockström, J., Richardson, K., Lenton, T. M., Folke, C., Liverman, D., Summerhayes, C. P., Barnosky, A. D., Cornell, S. E., Crucifix, M., Donges, J. F., Fetzer, I., Lade, S. J., Scheffer, M., Winkelmann, R., and Schellnhuber, H. J.: Trajectories of the Earth system in the Anthropocene, P. Natl. Acad. Sci. USA, 115, 8252–8259, https://doi.org/10.1073/pnas.1810141115, 2018.
Still, C. J., Berry, J. A., Collatz, G. J., and DeFries, R. S.: Global distribution of C3 and C4 vegetation: Carbon cycle implications, Global Biogeochem. Cy., 17, 6-1–6-14, 2003.
Stips, A., Macias, D., Coughlan, C., Garcia-Gorriz, E., and Liang, X. S.: On the causal structure between CO2 and global temperature, Sci. Rep.-UK, 6, 21691, https://doi.org/10.1038/srep21691, 2016.
Stolper, D. A., Bender, M. L., Dreyfus, G. B., Yan, Y., and Higgins, J. A.: A Pleistocene ice core record of atmospheric O2 concentrations, Science, 353, 1427–1430, 2016.
Stroeven, A. P., Hättestrand, C., Kleman, J., Heyman, J., Fabel, D., Fredin, O., Goodfellow, B. W., Harbor, J. M., Jansen, J. D., Olsen, L., Caffee, M. W., Fink, D., Lundqvist, J., Rosqvist, G. C., Strömberg, B., and Jansson, K. N.: Deglaciation of Fennoscandia, Quaternary Sci. Rev., 147, 91–121, 2016.
Tesi, T., Muschitiello, F., Smittenberg, R. H., Jakobsson, M., Vonk, J. E., Hill, P., Andersson, A., Kirchner, N., Noormets, R., Dudarev, O., Semiletov, I., and Gustafsson, Ö.: Massive remobilization of permafrost carbon during post-glacial warming, Nat. Commun., 7, 13653, https://doi.org/10.1038/ncomms13653, 2016.
Torres, M. A., West, A. J., and Li, G.: Sulphide oxidation and carbonate dissolution as a source of CO2 over geological timescales, Nature, 507, 346–349, 2014.
Torres, M. A., Moosdorf, N., Hartmann, J., Adkins, J. F., and West, A. J.: Glacial weathering, sulfide oxidation, and global carbon cycle feedbacks, P. Natl. Acad. Sci. USA, 114, 1–6, 2017.
Tranter, M.: Glacial runoff as a sink for atmospheric CO2 during the last glacial-interglacial transition, in: Fourth International Symposium on the Geochemistry of the Earth's Surface, University of Leeds, 22–28 July 1996, Ilkley, Yorkshire, England, 709–713, 1996.
Uribe-Alvarez, C., Ayala, M., Perezgasga, L., Naranjo, L., Urbina, H., and Vazquez-Duhalt, R.: First evidence of mineralization of petroleum asphaltenes by a strain of Neosartorya fischeri, Microb. Biotechnol., 4, 663–672, 2011.
Vance, D., Teagle, D. A. H., and Foster, G. L.: Variable Quaternary chemical weathering fluxes and imbalances in marine geochemical budgets, Nature, 458, 493–496, 2009.
Vonk, J. E., Dickens, A. F., Giosan, L., Hussain, Z. A., Kim, B., Zipper, S. C., Holmes, R. M., Montluçon, D. B., Galy, V., and Eglinton, T. I.: Arctic deltaic lake sediments as recorders of fluvial organic matter deposition, Front. Earth Sci., 4, https://doi.org/10.3389/feart.2016.00077, 2016.
Winterfeld, M., Mollenhauer, G., Dummann, W., Köhler, P., Lembke-Jene, L., Meyer, V. D., Hefter, J., McIntyre, C., Wacker, L., Kokfelt, U., and Tiedemann, R.: Deglacial mobilization of pre-aged terrestrial carbon from degrading permafrost, Nat. Commun., 9, 3666, https://doi.org/10.1038/s41467-018-06080-w, 2018.
Wong, M.-L., An, D., Caffrey, S. M., Soh, J., Dong, X., Sensen, C. W., Oldenburg, T. B. P., Larter, S. R., and Voordouw, G.: Roles of thermophiles and fungi in bitumen degradation in mostly cold oil sands outcrops, Appl. Environ. Microb., 81, 6825–6838, https://doi.org/10.1128/AEM.02221-15, 2015.
Wyndham, R. C. and Costerton, J. W.: In vitro microbial degradation of bituminous hydrocarbons and in situ colonization of bitumen surfaces within the Athabasca oil sands deposit, Appl. Environ. Microb., 41, 791–800, https://doi.org/10.1128/aem.41.3.791-800.1981, 1981.
Yu, Z., Wu, G., Li, F., Chen, M., Vi Tran, T., Liu, X., and Gao, S.: Glaciation enhanced chemical weathering in a cold glacial catchment, western Nyaingêntanglha Mountains, central Tibetan Plateau, J. Hydrol., 597, 126197, https://doi.org/10.1016/j.jhydrol.2021.126197, 2021.
Zeng, N.: Glacial-interglacial atmospheric CO2 change — The glacial burial hypothesis, Adv. Atmos. Sci., 20, 677–693, 2003.
Zeng, N.: Quasi-100 ky glacial-interglacial cycles triggered by subglacial burial carbon release, Clim. Past, 3, 135–153, https://doi.org/10.5194/cp-3-135-2007, 2007.
Zhao, N., Marchal, O., Keigwin, L., Amrhein, D., and Gebbie, G.: A synthesis of deglacial deep-sea radiocarbon records and their (in)consistency with modern ocean ventilation, Paleoceanogr. Paleocl., 33, 128–151, 2018.
ZoBell, C. E.: Action of microörganisms on hydrocarbons, Bacteriol. Rev., 10, 1–49, 1946.
In this work, kerogen is used as an umbrella term for all rock-derived forms of reduced carbon including soluble, insoluble, rock-disseminated, rock-forming, and solid and liquid forms, as well as fossil palynomorphs, biogenic and abiogenic graphite.
- Abstract
- Introduction
- Carbon isotopes and contradictions?
- Kerogen and glaciers – dynamic modulators of the global carbon cycle?
- A Canadian tale – and what about microbes?
- Tackling geologic deep time
- Synthesis and outlook
- Data availability
- Competing interests
- Disclaimer
- Acknowledgements
- Financial support
- Review statement
- References
- Supplement
- Abstract
- Introduction
- Carbon isotopes and contradictions?
- Kerogen and glaciers – dynamic modulators of the global carbon cycle?
- A Canadian tale – and what about microbes?
- Tackling geologic deep time
- Synthesis and outlook
- Data availability
- Competing interests
- Disclaimer
- Acknowledgements
- Financial support
- Review statement
- References
- Supplement