the Creative Commons Attribution 4.0 License.
the Creative Commons Attribution 4.0 License.
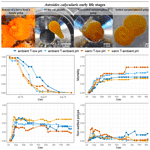
Early life stages of a Mediterranean coral are vulnerable to ocean warming and acidification
Chloe Carbonne
Steeve Comeau
Phoebe T. W. Chan
Keyla Plichon
Jean-Pierre Gattuso
Núria Teixidó
The ability of coral populations to recover from disturbance depends on larval dispersion and recruitment. While ocean warming and acidification effects on adult corals are well documented, information on early life stages is comparatively scarce. Here, we investigate whether ocean warming and acidification can affect the larval and recruit development of the Mediterranean azooxanthellate coral Astroides calycularis. Larvae and recruits were raised for 9 months at ambient (23 ∘C) and warm (26 ∘C) temperatures and ambient (8.0) and low pH (7.7, on the total scale). The timing of the larval metamorphosis, growth of the recruit polyp by linear extension and budding, and skeletal characteristics of the 9-month-old polyps were monitored. Settlement and metamorphosis were more successful and hastened under a warm temperature. In contrast, low pH delayed the metamorphosis and affected the growth of the recruits by reducing the calcified area of attachment to the substrate as well as by diminishing the skeleton volume and the number of septa. However, skeleton density was higher under low pH and ambient temperature. The warm temperature and low-pH treatment had a negative impact on the survival, settlement, and growth of recruits. This study provides evidence of the threat represented by ocean warming and acidification for the larval recruitment and the growth of recruits of A. calycularis.
- Article
(2084 KB) - Full-text XML
-
Supplement
(1085 KB) - BibTeX
- EndNote
Anthropogenic atmospheric CO2 emissions are driving major global threats to corals such as ocean warming and acidification (Kleypas et al., 2001). Under the high-CO2 emission scenario SSP5 – RCP8.5, sea surface temperature is expected to increase globally by +3.2 ∘C and ocean pH to decrease by 0.3 units by the end of the century (Kwiatkowski et al., 2020). Ocean warming has well-documented negative impacts on tropical reefs, where it can induce coral bleaching, which leads to a reduction in growth, reproduction, recruitment, and high mortality over large spatial scales (McClanahan et al., 2009; Sully et al., 2019). Ocean acidification, the change in carbonate chemistry resulting from the uptake of atmospheric CO2 by the ocean (Gattuso and Hansson, 2011), is responsible for decreasing seawater pH and the calcium carbonate saturation state (Orr et al., 2005). Calcifying species such as corals are perceived to be especially threatened by ocean acidification, as many studies have shown a decline in adult tropical coral calcification and potential accretion with a pH reduction (Cornwall et al., 2021). Mediterranean anthozoans, including scleractinian corals (six species) and gorgonians (seven species), have been suffering regular mass mortalities due to the increased intensity, duration and frequency of marine heat waves (Garrabou et al., 2022). However, temperate corals seem to be more tolerant of ocean acidification than tropical corals as their calcification is rarely affected by low pH (Rodolfo-Metalpa et al., 2011; Carbonne et al., 2021). The recovery of coral populations depends not only on adult resilience, but also on successful sexual reproduction, larval development and recruitment (Bahr et al., 2020).
Sexual reproduction is essential for the dynamics of populations since it maintains genetic diversity and favors adaptation to changing environmental conditions (Bay and Palumbi, 2014). It also facilitates recovery by replenishing populations after disturbances and maintaining resilience of marine communities (Hughes et al., 2019). Effective sexual reproduction is a complex process defined by different life-history factors: (1) gamete production and fertilization leading to high pelagic larval dispersal, (2) habitat selection by recognition of a suitable substrate and high recruitment by settlement, and (3) post-settlement growth and survival (Ritson-Williams et al., 2009; Albright, 2011). Although the production of larvae and early life stages are critical processes for rebuilding adult populations, corals in these early stages can be particularly sensitive to ongoing and projected environmental changes (Adjeroud et al., 2016). However, little is known about the response of coral larvae and recruits to ocean warming and acidification.
Ocean warming can be deleterious to coral larvae. For example, in early development, high temperatures can lead to embryonic abnormality (Woolsey et al., 2013). Furthermore, the survival of larvae and recruits is frequently reduced under ocean warming (Baria et al., 2015; Bahr et al., 2020). Elevated sea surface temperature is commonly related to higher metabolic rates, resulting in faster metamorphosis (Chua et al., 2013) and settlement (Nozawa and Harrison, 2007) and increasing larval retention to the native population.
Unlike warming, the response of coral early life stages to ocean acidification is equivocal. In some cases, lower pH leads to decreasing larval metabolism, metamorphosis, and settlement (Albright, 2011; Nakamura et al., 2011). However, Chua et al. (2013) have shown that embryonic development and metamorphosis of two coral species are differently affected by elevated pCO2 (low pH). On the other hand, most of the literature reports little impact of acidification on the survival of larvae and recruits (Suwa et al., 2010; Chua et al., 2013). As for adult corals, low pH has a negative impact on post-settlement calcification (Suwa et al., 2010; Varnerin et al., 2020) and induces skeletal deformities in recruits (Foster et al., 2016).
Although studies of the effects of warming and acidification in isolation are few, even fewer have assessed the combined effects of both stressors in the early life stages. Regarding adult corals, the combined effects of both high temperature and low pH are generally dependent on their intensities. For example, a moderate warming can counteract the impact of acidification, ending up with a neutral effect on calcification of adult corals (Kornder et al., 2018). Warming and acidification will have an additive effect (higher than the individual effects) on calcification rates under RCP4.5 and a synergistic effect (more than the sum of the individual effects) under RCP8.5 for the end of the century (Cornwall et al., 2021). In the early life stages, warming and acidification induce divergent responses with decreased or enhanced calcification (Anlauf et al., 2011; Foster et al., 2015). In contrast, studies have reported no effects of combined warming and acidification on larval survival, metamorphosis and settlement (Anlauf et al., 2011; Chua et al., 2013; Foster et al., 2015). However, most of the studies of the impact of both ocean warming and acidification on early life stages have focused on tropical zooxanthellate coral species (e.g., Albright, 2011; Baria et al., 2015), while studies on temperate corals are scarce (Caroselli et al., 2019; Varnerin et al., 2020).
Here, we assess the response of early life stages of the temperate azooxanthellate coral, Astroides calycularis, to the combined effects of elevated temperature and low pH. This colonial scleractinian coral is endemic to the Mediterranean Sea and is found between 0 and 50 m depth, more commonly in shallow rocky habitats (Zibrowius, 1995). Colonies are gonochoric (Goffredo et al., 2011), and fertilization occurs from April to May, with sperm release coinciding with increasing photoperiod and water temperature (Goffredo et al., 2011). A. calycularis is an internal brooder as egg fertilization takes place in the coelenteron, and females brood the embryos until they are fully developed into mature swimming larvae. Larvae are released at the beginning of summer when temperature increases (∼23 ∘C, Goffredo et al., 2011). Previous studies on A. calycularis adult colonies have shown that this coral is tolerant of ocean warming and acidification (Movilla et al., 2016; Teixidó et al., 2020; Carbonne et al., 2021).
In the present study, we hypothesize that elevated temperature and low pH have an additive or synergistic effect on the development of the larvae and growth by linear extension and polyp budding of recruits of A. calycularis. To test this hypothesis, larvae of A. calycularis were exposed in a fully factorial design to ambient and warm temperatures (23 and 26 ∘C) and ambient and low pH (pHT 8.05 and 7.7). They were maintained in the laboratory for 9 months to study the impact of these conditions on the development of larval stages, growth of recruit polyps, and skeleton density of the 9-month-old polyps.
2.1 Sampling site and larval release
Fifty-six colonies of Astroides calycularis (∼5 cm in diameter) were collected on 1 July 2020 by scuba diving in Ischia, Italy, at the Sant'Angelo site (40∘41′31.1′′ N, 13∘53′35.0′′ E; Fig. S1 in the Supplement). They were maintained in a 30 L tank with water motion provided by a NEWA mini 606 pump and maintained in the dark. Larvae were observed by transparency in the gastrovascular cavity and tentacles of the female colonies (Fig. S2). Larvae were released from the mouth of female polyps when the body contracted or when the colonies were touched (Video S1 and Fig. 1a). The release took place on the night of 1–2 July 2020; in situ seawater temperature was around 23 ∘C. Released larvae were fully mature (Video S2). They were collected with a pipette, pooled, stored in two 300 mL airtight plastic boxes filled with seawater, and transported to the aquarium facilities of the laboratory at Villefranche-sur-Mer, France, in less than 12 h.
2.2 Experimental setup and treatments
After 72 h of acclimation to the laboratory at ambient pH (pHT=8.05) and temperature (23 ∘C), the 180 larvae were maintained in the laboratory under two constant pH and two constant temperature treatments for 9 months. pH treatments were ambient pH (pHT∼8.05) and a low pH (pHT∼7.70), corresponding to a decline in pH projected by the end of the century under the RCP8.5 CO2 emissions scenario (Kwiatkowski et al., 2020). The two temperature treatments were ambient temperature, which was the in situ temperature at the time of sampling (23 ∘C), and warm temperature, ∼26 ∘C, which was shown to induce thermal stress of benthic species in the Mediterranean Sea (Gómez-Gras et al., 2019). The larvae were divided into 12×300 mL crystallizers (Duran®) as triplicates with 15 larvae for each of the four treatment conditions (2 pH × 2 temperatures). Each crystallizer was submerged in an independent 5 L experimental tank. While larvae were still swimming, the water was changed every day, and crystallizers were covered with parafilm® to prevent changes in pH and the escape of larvae. When all larvae metamorphosed and settled, the crystallizers were covered with a 45 µm plankton net to allow water exchange with the experimental tank. The experimental tanks were gravity-fed (50 mL min−1) from six 25 L header tanks (three at each pH treatment) continuously supplied by seawater pumped from the bay of Villefranche at 5 m depth. pH was controlled using a pH controller (APEX, Neptune Systems) which regulated the delivery of pure CO2. Temperature was regulated in the experimental tanks by temperature controllers (APEX, Neptune Systems) and a 300 W ThermoControl-e heater (Eheim), and both pH and temperature were measured every week (see below).
Light was provided by 24 W Aquablue Special T5 (ATI). Irradiance was constant from 7 to 19 h at 16 µmol photons . Submersible pumps (NEWA) provided water motion in each experimental tank. When recruit polyps had tentacles (approximately after a month, from 5 August 2020), they were fed twice a week with a 10 mL solution of freshly hatched brine shrimps (Artemia sp.); 24 h after feeding, the crystallizers were cleaned with a painting brush and the water changed to remove any detritus.
2.3 Carbonate chemistry
pH in the header and experimental tanks was measured weekly using a handheld pH meter (826 pH mobile, Metrohm) calibrated with a seawater pH TRIS buffer (batch no. T33 provided by Andrew G. Dickson, Scripps Institution of Oceanography, USA) before each set of measurements. Temperature was also measured weekly in each tank with a TraceableTM digital thermometer (FisherBrand). Salinity and total alkalinity (AT) data during the experiment were obtained from the weekly measurements performed in the bay of Villefranche by the Service d'Observation Rade de Villefranche, SO-Rade, of the Institut de la mer de Villefranche and the Service d'Observation en Milieu Littoral, SOMLIT/CNRS-INSU. This was possible because we worked in an open system where seawater from the bay of Villefranche was continuously delivered. To confirm that AT was not altered by metabolic activity, it was measured in each experimental and header tank at the beginning and end of the experiment. It was determined by potentiometric titration using a Metrohm 888 Titrando following the method of Dickson et al. (2007). Titrations of certified reference material (batch no. 186) provided by Andrew G. Dickson were used to assess the accuracy of the measurements and were within 6.5 µmol kg−1 of the reference value. pHT, temperature, AT and salinity were used to calculate the other carbonate chemistry parameters using R package seacarb (Gattuso et al., 2021).
2.4 Early life-stage monitoring
The life stages of all 15 individuals (larvae) per crystallizer were recorded every 2 d when swimming larvae were present and every week after settlement. The different stages were “planula” when the larvae were still swimming (Fig. 1b, Video S3), “settled polyp” when larvae had metamorphosed and settled (Fig. 1d), “non-settled polyp” when larvae had metamorphosed but not settled or detached from the substrate after settling (Fig. 1c), and “dead”. Missing larvae were assumed to be dead as coral larvae lyse within 24 h after death (Baird et al., 2006).
Pictures of each larva from each crystallizer were taken every 2 to 3 d with a stereomicroscope (SteREO, Discovery V.12, Zeiss) coupled with a camera (D5100, Nikon) until no swimming planula was present. For each polyp attached to the glass of the crystallizer, a picture was taken every 2 weeks from below with a camera (Coolpix W300, Nikon) and a ruler used as a scale (Fig. 3a). All pictures were analyzed with ImageJ to measure the maximum length and maximum width of the larvae and the surface of the polyp base (Fig. 3a). Polyp budding, the appearance of new polyps from one initial polyp, was recorded and reported as the number of polyps in one colony (Fig. 3c).
2.5 Skeleton analysis
After 9 months, the polyps were collected and placed in 5 % sodium hypochlorite for 2 h and then rinsed with Milli-Q water to remove organic tissue from the skeleton. The skeletons were dried at ambient temperature for 2 d. The perpendicular diameters (D and d) and the height (h) were measured using a digital caliper. The volume of the skeleton was calculated using the following equation: V =π * D * d * h. To count the number of septa, a picture of each skeleton was taken from the top with a stereomicroscope (SteREO, Discovery V.12, Zeiss) mounted with a camera (D5100, Nikon).
Six skeletons of each condition (total of 24) were scanned in a micro-CT scanner (GE Healthcare, eXplore Locus RS; see the micro-CT scanner setup in Supplement Fig. S3). Micro-CT imaging produced a 3D distribution of linear attenuation coefficients that was stored as an X-ray volume image for each coral specimen, defined by the coral polyp base along the X–Y in-plane axis and the growth axis following the Z axis (Fig. 5a, Video S4). Sample visualization and analysis were performed using MicroView Standard 2.5.0–2702 (Parallax Innovations Inc., updated 2015) to reconstruct each specimen in 3D and to digitize a region of interest (ROI, delineating the region to be analyzed). Six coral specimens were imaged in every micro-CT scan, each representing one of the four laboratory treatments (temperature × pH). The ROI was plotted as a square-based prism spanning the entire specimen to crop each coral sample out for individual analysis. A threshold greyscale value of 2500 HU was carefully selected to remove pore spaces from a coral skeleton. This approach ensured consistent sampling focused on skeletal aragonite only. Voxels with greyscale values below the threshold of 2500 HU were deemed to be empty space and were excluded from the skeletal density calculation. However, small pore spaces (<20 µm) were included in the skeletal density calculation since they were unresolvable at 20 µm resolution. The skeletal density for each coral polyp (mg cm−3) was determined using the fractional mineral content of each voxel (3D pixel) above the greyscale threshold (to exclude pore spaces), averaged over all the voxels contained within each region of interest and linearly rescaled to units of pure crystal aragonite (density = 2.95 g cm−3).
2.6 Data analysis
Linear mixed models with a hierarchical structure were used to evaluate the treatment effects through time on the number of larvae in each life stage (planula, settled polyp, non-settled polyp, dead) and the length and width of the larvae. Hierarchical linear models were used since data were compiled from repeated measurements of the same pool of larvae over time. The models were fitted using function lmer of R package lme4 (Bates et al., 2015). The fixed factors of the models were temperature, pH and time, and crystallizers and time were assigned as random effects. The structure of the random term was selected by comparing models with different error structures using the Akaike information criterion (Table S1 in the Supplement). For parameters that are not time-dependent, generalized linear mixed models (GLMMs) were used with a Gaussian distribution to test for the effects of the treatments on the surface of the polyps' base, the skeletal density and volume, and a Poisson distribution for the number of polyps per colony and the number of septa. Temperature and pH were fixed factors and crystallizers of each condition random factors.
3.1 Experimental conditions
The ambient pH treatment was maintained at a mean pHT of 8.05±0.09 (mean ± SD, n=324) and the low-pH treatment at a mean pHT of 7.78±0.10 (mean ± SD, n=324, total number of weekly pHT discrete measurements in each ambient pH experimental tank, Fig. S4b, Table 1). The ambient temperature treatment was maintained at 23.5±1.6 ∘C (mean ± SD, n=342) and the warm temperature treatment at 26.6±0.8 ∘C (mean ± SD, n=342, Fig. S4a, Table 1). Salinity and total alkalinity did not vary much for the duration of the experiment, with a salinity of 37.84±0.17 (mean ± SD, n=71, Table 1) and a total alkalinity of 2548±13 µmol kg−1 (mean ± SD, n=71, Table 1).
Table 1Measured and estimated seawater physiochemical parameters of the two pH treatments in the experimental tanks for salinity (S), temperature (T), total alkalinity (AT), dissolved inorganic carbon (CT), pHT, pCO2, calcite (Ωc) and aragonite (Ωa) saturation. Values are means ± SD with the 25th and 75th percentiles. Calculated concentrations of CT, pCO2, Ωc and Ωa are shown. * Parameters measured from discrete water samples. pH and temperature conditions are the experimental pH and temperature treatments.
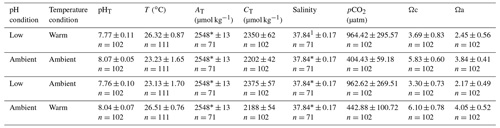
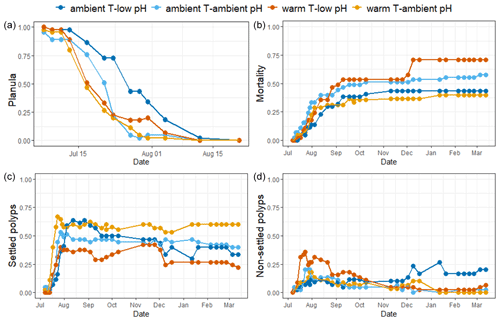
Figure 2Proportions of four different stages of A. calycularis under the two temperature treatments (23 ∘C for ambient temperature and 26 ∘C for warm temperature) and the two pH treatments (pH for ambient pH and pH for low pH) during 9 months. (a) The proportion of planulas (swimming larvae), (b) the proportion of mortality (dead larvae and polyps), (c) the proportion of settled polyps (metamorphosed and fixed), and (d) the proportion of non-settled polyps (metamorphosed but not fixed). The proportion of non-settled polyps includes larvae metamorphosed into a free polyp and detachment of a settled polyp. n=180 original larvae, three replicates per temperature and pH treatments.
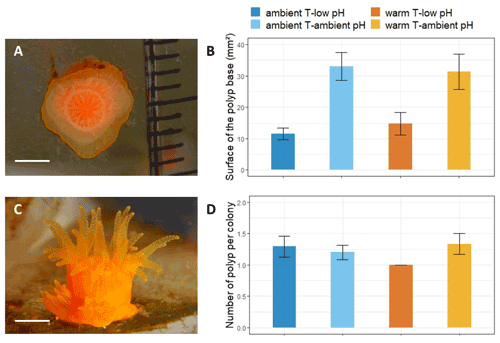
Figure 3Mean surface of the recruits' base and mean number of polyps per colony of A. calycularis under the two temperature treatments (23 ∘C for ambient temperature and 26 ∘C for warm temperature) and the two pH treatments (pH for ambient pH and pH for low pH) after 9 months. Values are means ± SE. (a) Picture of the base of a recruit through the glass of the crystallizer, (b) mean surface of the recruits' base at 9 months old, (c) picture of a recruit with two new polyps on the outskirts, and (d) mean number of polyps per colony. The colors of the bars indicate the temperature and pH treatment. n=9–20 per condition. Scale: 2 mm.
3.2 Development of early life stages
Larvae started metamorphosing into settled and non-settled polyps after 5 d under warm temperature–ambient pH, and the last larva settled after 41 d under ambient temperature–low pH. The proportion of larvae was significantly affected by the interaction of pH, temperature and time (Fig. 2a, , p=0.035, Table S2), as larvae under warm temperature conditions metamorphosed more quickly, 50 % in 15 d, while ambient temperature–ambient pH and ambient temperature–low pH conditions reached 50 % of metamorphosis after 19 and 25 d, respectively (Fig. 2a). Settlement of larvae differed between conditions: only 25 % of settlement occurred in the warm temperature–low pH condition and more than 40 % in the two ambient pH treatment conditions (Fig. 2c) due to a significant interaction among the three factors pH, temperature and time (, p<0.001, Table S2, Fig. 2c). There was a significant effect of pH, temperature and time on the proportion of metamorphosed but non-settled larvae (, p<0.001, Table S2). The two low pH treatment conditions presented an opposite outcome. The warm temperature–low pH treatment had 36 % of non-settled polyps at the beginning of the experiment and almost 0 % after 3 months (Fig. 2d). On the other hand, the ambient temperature–low pH condition presented a progressive detachment of polyps as the proportion of non-settled polyps increased from 9 % after a month, reaching 25 % after 6 months (Fig. 2d). Mortality of larvae and polyps increased for every condition during the first 2 months of the experiment and then reached a plateau around 50 % (Fig. 2b). The warm temperature–low pH condition presented higher mortality compared to the other conditions, reaching up to 71 %, with a significant effect of the combined pH, temperature and time (, p<0.001, Table S2, Fig. 2b). The treatments did not affect larval size (Fig. S7).
3.3 Growth of the recruits by linear extension
The surfaces of the polyp bases were significantly affected by pH (, Table S3). The base surfaces in the ambient pH treatment were significantly larger than under low pH, reaching 32.7±3.6 mm2 (mean ± SE, n=18) and 13±2 mm2 (n=21), respectively, after 9 months of experiment (Figs. 3b and S5).
3.4 Development of new polyps by budding
New polyps started to bud on the periphery of the recruits at the end of December 2020 (Fig. S6). The number of polyps per colony after 9 months of experiment was not significantly different across treatments (, p=0.135, Table S3). However, in contrast to all other treatments, recruits in the warm temperature–low pH treatment did not develop new polyps during the 9 months of the experiment (Fig. 3d). The recruits in the warm temperature–ambient pH treatment exhibited new polyps first as well as the highest number of polyps per colony at the end of the experiment, with a mean of 1.33 polyps per recruit.
3.5 Skeleton analysis
The size of the skeleton was significantly impacted by the pH treatment (, p=0.044, Table S3), with a mean volume of 0.12 cm3 in the ambient pH treatment and 0.1 cm3 in the low pH treatment (Fig. 4b). The number of septa per skeleton was significantly lower under low pH (, p=0.002, Table S3). The skeleton had less developed septa (mean ± SE, n=29) in the low pH treatment (8.2±0.6 septa) than under ambient pH (10.2±0.6 septa, n=23, Fig. 4c).
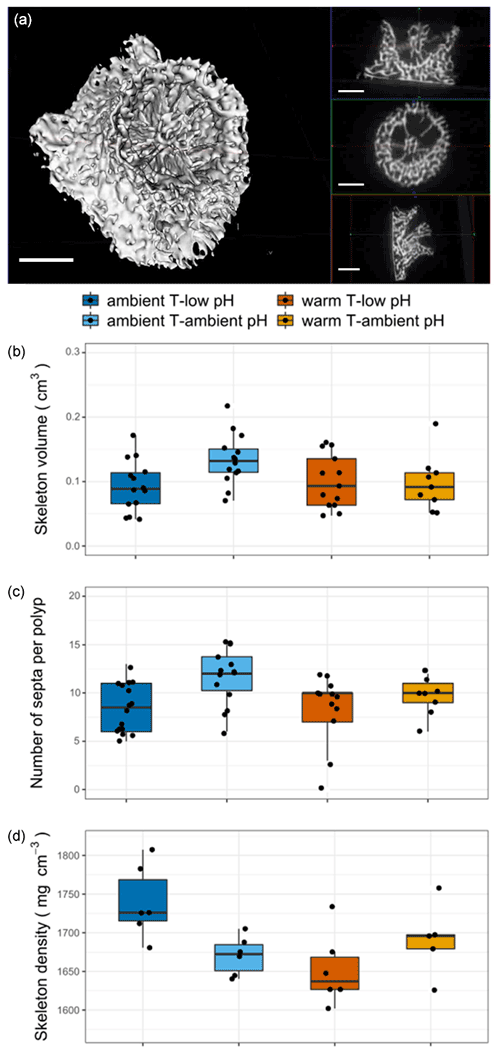
Figure 4Nine-month recruit skeletons of A. calycularis under the two temperature treatments (23 ∘C for ambient temperature and 26 ∘C for warm temperature) and the two pH treatments (pH for ambient pH and pH for low pH). (a) 3D picture of a recruit skeleton by micro-CT scanning, scale: 1 mm; see also Video S4. (b) Volume of the recruit. n=9–16 per temperature and pH treatment. (c) Number of septa per skeleton. n=9–16 per temperature and pH treatment. (d) Skeleton density. Values have been obtained by micro-CT scanning. n=6 per temperature and pH treatment. Dots represent the number of septa per recruit coral skeleton, and the density of the recruit's coral skeleton and boxes represents the median and 25 % and 75 % quartiles. The color of the boxes indicates the temperature and pH treatment.
The combined effect of pH and temperature had a significant impact on skeletal density (, p=0.012, Table S3). The skeletons of polyps exposed to the ambient temperature–low pH condition presented a higher density of 1739±19 mg cm−3 (mean ± SE, n=6) than in the other conditions, in which it was lower than 1690 mg cm−3 (n=6, Fig. 4a and d).
This experiment shows that settlement and metamorphosis of the larvae are faster under warm temperatures. In contrast, low pH delayed the metamorphosis and affected the skeletal properties of the recruits. The surface of the base of the skeleton and the number of septa per polyp were lower under low pH conditions, whereas the skeleton density was higher. Both drivers combined had a negative synergistic impact on survival and settlement and an additive impact on growth by linear extension and polyp budding. Therefore, our results suggest that both warming and acidification, individually or combined, affect the early life stages of Astroides calycularis.
Larvae were released by the female colonies at the beginning of summer (end of June), when the sea surface temperatures reached 23 ∘C. Metamorphosis and settlement were accelerated in the warm treatment. Similar results were observed in the majority of similar studies in which tropical coral larval development was noticeably increased at warmer temperatures (Edmunds et al., 2001; Nozawa and Harrison, 2007; Chua et al., 2013). In contrast, few studies reported a decline in settlement and development of tropical coral larvae under warming (e.g., Randall and Szmant, 2009), possibly due to the high temperature (31 and 33 ∘C) that reduced larval mobility and increased mortality. Higher temperatures accelerate biochemical processes by increasing the activity of enzymes until a threshold is reached (Clarke and Fraser, 2004). As a result, metabolic rates increase, shortening the duration of the larval phase and reducing the distance of dispersal by causing metamorphosis and settlement before larvae are exported from their population of origin (O'Connor et al., 2007; Figueiredo et al., 2014). Local retention of the larvae has a direct impact on connectivity and genetic diversity, which potentially reduces the resilience to disturbances and future global change (Bay and Palumbi, 2014). Warmer temperatures are also commonly associated with an increased mortality of early life stages (Baria et al., 2015). Here, the mortality of larvae and recruits was not affected by temperature. This might be because A. calycularis is a known thermophilic species, with a southwestern distribution in the Mediterranean Sea. The warm temperature used for the experiment, 26 ∘C, is the mean temperature during the summer months in Ischia (July and August, Teixido et al., 2020). The colonies of A. calycularis have been suffering mass mortalities in Ischia and north of Sicily in summer when temperatures reach 28 ∘C (Gambi et al., 2018; Bisanti et al., 2022).
The surface area of the base of the skeleton was highly impacted by acidification, decreasing by 60 % under low pH compared to ambient pH treatment. The skeletal volume was also reduced by 19 % under acidified conditions. Albright and Langdon (2011) also observed a decrease in post-settlement linear growth of 50 % in recruits of Porites astreoides, with a similar pHT treatment of 7.7. This difference in growth might be due to a decrease in metabolism under low pH (Albright and Langdon, 2011). The formation of the skeleton was also impacted by acidification as the number of septa was less abundant and did not present a radial symmetry under low pH. This is in agreement with other studies which have reported porous corallite walls, a thinner basal plate, and an asymmetric skeleton under similar low-pH conditions (Foster et al., 2016). This impact on the linear extension and skeleton structure can likely explain the increased detachment of polyps 5 months after the beginning of the experiment. A large number of recruits under low pH had the skeleton base exposed, retracting their tissue towards the upper section of the corallite (Fig. S8). While 17 out of 36 polyps exposed to low pH had an exposed skeleton at the base, none of the 33 polyps under ambient pH exhibited tissue retraction. The polyps were thus unable to extend their skeleton on the substrate, and the exposed skeleton was likely experiencing dissolution, as described before with Cladocora caespitosa by Rodolfo-Metalpa et al. (2011). This phenomenon of being more predisposed to dissolution may be compared to the loss of coenosarc (the living tissue connecting the polyps) in adult colonies of A. calycularis that naturally occur in a CO2 vent site in Ischia, where seawater is naturally acidified (Teixido et al., 2020). As a response to low pH, colonies in the CO2 vent showed a reduction of coenosarc compared to ambient pH sites. Interestingly, while the linear extension and structure of the skeleton were affected by low pH, the density of the skeleton was higher. These results are in accordance with the higher density observed in adult colonies living in the CO2 vent site in Ischia (Teixido et al., 2020). It suggests that A. calycularis responds to low pH by increasing the density of their skeleton, perhaps for greater resistance to mechanical stress, at the expense of other physiological parameters such as growth and skeleton structure. These results suggest that acidification mainly had repercussions for the calcification of the recruits, whereas larval development and survival were not impacted, as observed by Foster et al. (2015).
The proportion of settlement of A. calycularis larvae was significantly lower in the warm temperature and low-pH treatment. In contrast, other studies reported no effect of such a treatment (e.g., Anlauf et al., 2011; Foster et al., 2015). This difference can be explained by the higher magnitude of the warming and acidification treatments used in the present study compared to other studies (+3 ∘C vs. +1 to 2 ∘C and −0.3 pH unit vs. −0.2 pH unit) (Anlauf et al., 2011; Chua et al., 2013; Bahr et al., 2020). Furthermore, all previous studies focused on tropical zooxanthellate corals, which obtain additional energy by photosynthetic products translocated from their symbionts (Davy et al. 2012) and are not subject to high seasonal variability of temperature like Mediterranean species (around 14 ∘C in winter to 26 ∘C in summer in Ischia). The low settlement rate of A. calycularis under the warm and acidified treatment is related to the high number of larvae experiencing metamorphosis with no settlement during the first days of the experiment. In the first 15 d, 36 % of the larvae metamorphosed without settling. After metamorphosing, the individuals floated for a week before sinking, and then calcification started and the tentacles appeared. Some of them eventually attached to the bottom after 3 months. This phenomenon could also explain the high mortality rate observed in the warm temperature–low pH condition as non-settled polyps could not feed properly. Recruits were therefore less abundant, and their size was smaller than in any other condition. Warming and acidification presented an additive effect on the surface base of the recruits as the combined effects of warm and acidified waters led to a 63 % reduction in linear growth, while the low-pH treatment caused a 55 % decrease and the warm treatment a 6 % decrease compared to the control condition. Anlauf et al. (2011) found a synergistic effect of warming and acidification on the calcification of Porites panamensis recruits. The calcification of the recruits was 30 % smaller in a combined warm and low-pH treatment, while only a 3 % decrease was observed under acidification alone and 0 % under warming. For Acropora spicifera recruits, warming mitigated the impact of acidification as calcification was lower (−60 %) under sole acidification than under both warming and acidification (−48 %, Foster et al., 2015). The impact of stressors on calcification is considered species-specific (Comeau et al., 2013). However, methodological differences could also explain the range of responses observed. The duration of our experiment (9 months) was longer than previous ones (6 weeks, Anlauf et al., 2011, and 5 weeks, Foster et al., 2015). For example, in our study, the different responses to the treatments on A. calycularis recruit surface bases were only observed 2 months post-settlement. Finally, under the warm temperature–low pH condition, 63 % of the recruits presented an exposed skeleton at the base, more than under the ambient temperature–low pH condition. However, new polyps of A. calycularis bud from the tissue at the base of the recruit periphery. This particular characteristic explains why the recruits under the double-stress condition were the only one not presenting new polyps. Thus, warm temperature and low pH together harmed the development of recruits into colonies.
Our findings highlight that warm temperatures and acidification have distinct impacts on the early life stages of A. calycularis. Temperature acts on larval development, while pH acts on the linear growth and calcification of the recruits, as observed in previous studies on tropical species (e.g., Albright and Langdon, 2011; Chua et al., 2013). The combined effect of warming and acidification in early life stages will negatively impact the resilience and resistance of the Mediterranean populations by decreasing dispersion, recruitment and post-settlement growth. In order to better predict the future of A. calycularis populations at the end of the century under global change, further research needs to be done to evaluate whether acclimatization or adaptation to warming or acidification can occur in early life stages.
The data used in this paper are available from PANGAEA: https://doi.org/10.1594/PANGAEA.948263 (Carbonne et al., 2022a).
-
Video 1. Spawning of a larva from the mouth of an Astroides calycularis female polyp: https://doi.org/10.5446/58541 (Carbonne et al., 2022b);
-
Video 2. One-day-old swimming larvae of Astroides calycularis: https://doi.org/10.5446/58542 (Carbonne et al., 2022c);
-
Video 3. Sequence of early life stage development of Astroides calycularis: https://doi.org/10.5446/58543 (Carbonne et al., 2022d).
The supplement related to this article is available online at: https://doi.org/10.5194/bg-19-4767-2022-supplement.
CC, SC, NT and JPG designed the study. CC and NT were involved with fieldwork. CC, PC and KP performed the experiments. CC, NT and KP analyzed the data. CC wrote the first draft of the manuscript, which was then finalized by all the co-authors.
The contact author has declared that none of the authors has any competing interests.
Publisher's note: Copernicus Publications remains neutral with regard to jurisdictional claims in published maps and institutional affiliations.
This research was supported by the French government through the National Research Agency Investments for the Future (“4Oceans-Make Our Planet Great Again” grant, ANR-17-MOPGA-0001) and internal funds from the Stazione Zoologica Anton Dohrn (FOE-Teixido). The Service d'Observation Rade de Villefranche (SO-Rade) of the Institut de la mer de Villefranche and the Service d'Observation en Milieu Littoral (SOMLIT/CNRS-INSU) gave kind permission to use the Point B data. Christopher J. D. Norley and David W. Holdsworth from Robarts Research Institute of the University of Western Ontario (Canada) provided the use and expertise of their micro-CT scanner. The Western University Earth Sciences Department's Dana Minerals Collection provided the aragonite crystal used as a standard for the micro-CT scanning. We thank Samir Alliouane for assistance in the laboratory, Laura Tamburello for advice with statistical analysis, and Pietro Sorvino (ANS Diving, Ischia) and Alice Mirasole for assistance in the field.
This research has been supported by the Agence Nationale de la Recherche (4Oceans, MOPGA, grant no. ANR-17-MOPGA-0001).
This paper was edited by Andrew Thurber and reviewed by two anonymous referees.
Adjeroud, M., Kayal, M., and Penin, L.: Importance of recruitment processes in the dynamics and resilience of coral reef assemblages, in: Marine Animal Forests, Springer International Publishing, Cham, 1–21, https://doi.org/10.1007/978-3-319-17001-5_12-1, 2016.
Albright, R.: Reviewing the effects of ocean acidification on sexual reproduction and early life history stages of reef-building corals, J. Mar. Biol., 2011, 1–14, https://doi.org/10.1155/2011/473615, 2011.
Albright, R. and Langdon, C.: Ocean acidification impacts multiple early life history processes of the Caribbean coral Porites astreoides, Global Change Biol., 17, 2478–2487, https://doi.org/10.1111/j.1365-2486.2011.02404.x, 2011.
Anlauf, H., D'Croz, L., and O'Dea, A.: A corrosive concoction: The combined effects of ocean warming and acidification on the early growth of a stony coral are multiplicative, J. Exp. Mar. Biol. Ecol., 397, 13–20, https://doi.org/10.1016/j.jembe.2010.11.009, 2011.
Bahr, K. D., Tran, T., Jury, C. P., and Toonen, R. J.: Abundance, size, and survival of recruits of the reef coral Pocillopora acuta under ocean warming and acidification, PLoS One, 15, 1–13, https://doi.org/10.1371/journal.pone.0228168, 2020.
Baird, A. H., Gilmour, J. P., Kamiki, T. M., Nonaka, M., Pratchett, M. S., Yamamoto, H. H., and Yamasaki, H.: Temperature tolerance of symbiotic and non-symbiotic coral larvae, in: Proceeding of 10th International coral reef symposium, 28 June–2 July 2004, Okinawa, Japan, 38–42, 2006.
Baria, M. V. B., Kurihara, H., and Harii, S.: Tolerance to elevated temperature and ocean acidification of the larvae of the solitary corals Fungia fungites (Linnaues, 1758) and Lithophyllon repanda (Dana, 1846), Zoolog. Sci., 32, 447–454, https://doi.org/10.2108/zs150036, 2015.
Bates, D., Mächler, M., Bolker, B., and Walker, S.: Fitting Linear Mixed-Effects Models Using lme4, J. Stat. Softw., 67, 1–48, https://doi.org/10.18637/jss.v067.i01, 2015.
Bay, R. A. and Palumbi, S. R.: Multilocus adaptation associated with heat resistance in reef-building corals, Curr. Biol., 24, 2952–2956, https://doi.org/10.1016/j.cub.2014.10.044, 2014.
Bisanti, L., Sabata, E., Visconti, G., and Chemello, R.: Towards a local mass mortality of the Mediterranean orange coral Astroides calycularis (Pallas, 1766) in the Pelagie Islands Marine Protected Area (Italy), Aquat. Conserv. Mar. Freshw. Ecosyst., 32, 551–557, https://doi.org/10.1002/aqc.3772, 2022.
Carbonne, C., Teixidó, N., Moore, B., Mirasole, A., Guttierez, T., Gattuso, J., and Comeau, S.: Two temperate corals are tolerant to low pH regardless of previous exposure to natural CO2 vents, Limnol. Oceanogr., 66, 4046–4061, https://doi.org/10.1002/lno.11942, 2021.
Carbonne, C., Comeau, S., Chan, P. T. W., Plichon, K., Gattuso, J.-P., and Teixidó, N.: Seawater carbonate chemistry and the larval and juvenile development of the Mediterranean azooxanthellate coral Astroides calycularis, PANGAEA [data set], https://doi.org/10.1594/PANGAEA.948263, 2022a.
Carbonne, C., Comeau, S., Chan, P., Plichon, K., Gattuso, J.-P., and Teixidó, N.: Spawning of a larva from the mouth of Astroides calycularis female polyp, TIB AV-PORTAL [video supplement], https://doi.org/10.5446/58541, 2022b.
Carbonne, C., Comeau, S., Chan, P., Plichon, K., Gattuso, J.-P., and Teixidó, N.: One-day old swimming larvae of Astroides calycularis, TIB AV-PORTAL [video supplement], https://doi.org/10.5446/58542, 2022c.
Carbonne, C., Comeau, S., Chan, P., Plichon, K., Gattuso, J.-P., and Teixidó, N.: Sequence of early life stages development of Astroides calycularis, TIB AV-PORTAL [video supplement], https://doi.org/10.5446/58543, 2022d.
Caroselli, E., Gizzi, F., Prada, F., Marchini, C., Airi, V., Kaandorp, J., Falini, G., Dubinsky, Z., and Goffredo, S.: Low and variable pH decreases recruitment efficiency in populations of a temperate coral naturally present at a CO2 vent, Limnol. Oceanogr., 64, 1059–1069, https://doi.org/10.1002/lno.11097, 2019.
Chua, C. M., Leggat, W., Moya, A., and Baird, A.: Temperature affects the early life history stages of corals more than near future ocean acidification, Mar. Ecol. Prog. Ser., 475, 85–92, https://doi.org/10.3354/meps10077, 2013.
Clarke, A. and Fraser, K. P. P.: Why does metabolism scale with temperature?, Funct. Ecol., 18, 243–251, 2004.
Comeau, S., Edmunds, P. J., Spindel, N. B., and Carpenter, R. C.: The responses of eight coral reef calcifiers to increasing partial pressure of CO2 do not exhibit a tipping point, Limnol. Oceanogr., 58, 388–398, https://doi.org/10.4319/lo.2013.58.1.0388, 2013.
Cornwall, C. E., Comeau, S., Kornder, N. A., Perry, C. T., van Hooidonk, R., DeCarlo, T. M., Pratchett, M. S., Anderson, K. D., Browne, N., Carpenter, R., Diaz-Pulido, G., D'Olivo, J. P., Doo, S. S., Figueiredo, J., Fortunato, S. A. V., Kennedy, E., Lantz, C. A., McCulloch, M. T., González-Rivero, M., Schoepf, V., Smithers, S. G., and Lowe, R. J.: Global declines in coral reef calcium carbonate production under ocean acidification and warming, P. Natl. Acad. Sci. USA, 118, e2015265118, https://doi.org/10.1073/pnas.2015265118, 2021.
Dickson, A. G., Sabine, C. L., and Christian, J. R.: SOP 3a Determination of total alkalinity in sea water using an open-cell titration, in: Guide to best practices for ocean CO2 measurements, vol. 3, North Pacific Marine Science Organization, 1–15, 2007.
Edmunds, P. . J., Gates, R. D., and Gleason, D. F.: The biology of larvae from the reef coral Porites astreoides, and their response to temperature disturbances, Mar. Biol., 139, 981–989, https://doi.org/10.1007/s002270100634, 2001.
Figueiredo, J., Baird, A. H., Harii, S., and Connolly, S. R.: Increased local retention of reef coral larvae as a result of ocean warming, Nat. Clim. Change, 4, 498–502, https://doi.org/10.1038/nclimate2210, 2014.
Foster, T., Gilmour, J. P., Chua, C. M., Falter, J. L., and McCulloch, M. T.: Effect of ocean warming and acidification on the early life stages of subtropical Acropora spicifera, Coral Reefs, 34, 1217–1226, https://doi.org/10.1007/s00338-015-1342-7, 2015.
Foster, T., Falter, J. L., McCulloch, M. T., and Clode, P. L.: Ocean acidification causes structural deformities in juvenile coral skeletons, Sci. Adv., 2, e1501130, https://doi.org/10.1126/sciadv.1501130, 2016.
Gambi, M. C., Sorvino, P., Tiberti, L., and Teixidó, N.: Mortality Events of Benthic Organisms Along the Coast of Ischia in Summer 2017, Biol. Mar. Mediterr., 25, 212–213, 2018.
Garrabou, J., Gómez-Gras, D., Medrano, A., Cerrano, C., Ponti, M., Schlegel, R., Bensoussan, N., Turicchia, E., Sini, M., Gerovasileiou, V., Teixido, N., Mirasole, A., Tamburello, L., Cebrian, E., Rilov, G., Ledoux, J.-B., Ben Souissi, J., Khamassi, F., Ghanem, R., Benabdi, M., Grimes, S., Ocaña, O., Bazairi, H., Hereu, B., Linares, C., Kersting, D. K., la Rovira, G., Ortega, J., Casals, D., Margarit, N., Pagès-Escolà, M., Capdevila, P., Verdura, J., Ramos, A., Izquierdo, A., Barbera, C., Rubio-Portillo, E., Anton, I., López-Sendino, P., Díaz, D., Vázquez-Luis, M., Duarte, C., Marbà, N., Aspillaga, E., Espinosa, F., Grech, D., Guala, I., Azzurro, E., Farina, S., Gambi, M. C., Chimienti, G., Montefalcone, M., Azzola, A., Pulido Mantas, T., Fraschetti, S., Ceccherelli, G., Kipson, S., Bakran-Petricioli, T., Petricioli, D., Jimenez, C., Katsanevakis, S., Tuney Kizilkaya, I., Kizilkaya, Z., Sartoretto, S., Elodie, R., Ruitton, S., Comeau, S., Gattuso, J.-P., and Harmelin, J.-G.: Marine heatwaves drive recurrent mass mortalities in the Mediterranean Sea, Global Change Biol., https://doi.org/10.1111/gcb.16301, in press, 2022.
Gattuso, J.-P. and Hansson, L.: Ocean acidification: background and history, in: Ocean Acidification, edited by: Gattuso, J. and Hansson, L., Oxford University Press Oxford, 1–20, ISBN 978-0-19-959108-4, 2011.
Gattuso, J.-P., Epitalon, J.-M., Lavigne, H., and Orr, J.: seacarb: Seawater carbonate chemistry, R package version 3.3.0, https://cran.r-project.org/package=seacarb (last access: 20 September 2022), 2021.
Goffredo, S., Gasparini, G., Marconi, G., Putignano, M. T., Pazzini, C., Airi, V., and Zaccanti, F.: Sexual reproduction in the Mediterranean endemic orange coral Astroides calycularis (Scleractinia: Dendrophylliidae), Bull. Mar. Sci., 87, 589–604, https://doi.org/10.5343/bms.2010.1068, 2011.
Gómez-Gras, D., Linares, C., de Caralt, S., Cebrian, E., Frleta-Valić, M., Montero-Serra, I., Pagès-Escolà, M., López-Sendino, P., and Garrabou, J.: Response diversity in Mediterranean coralligenous assemblages facing climate change: Insights from a multispecific thermotolerance experiment, Ecol. Evol., 9, 4168–4180, https://doi.org/10.1002/ece3.5045, 2019.
Hughes, T. P., Kerry, J. T., Baird, A. H., Connolly, S. R., Chase, T. J., Dietzel, A., Hill, T., Hoey, A. S., Hoogenboom, M. O., Jacobson, M., Kerswell, A., Madin, J. S., Mieog, A., Paley, A. S., Pratchett, M. S., Torda, G., and Woods, R. M.: Global warming impairs stock–recruitment dynamics of corals, Nature, 568, 387–390, https://doi.org/10.1038/s41586-019-1081-y, 2019.
Kleypas, J. A., Buddemeier, R. W., and Gattuso, J.-P.: The future of coral reefs in an age of global change, Int. J. Earth Sci., 90, 426–437, https://doi.org/10.1007/s005310000125, 2001.
Kornder, N. A., Riegl, B. M., and Figueiredo, J.: Thresholds and drivers of coral calcification responses to climate change, Global Change Biol., 24, 5084–5095, https://doi.org/10.1111/gcb.14431, 2018.
Kwiatkowski, L., Torres, O., Bopp, L., Aumont, O., Chamberlain, M., Christian, J. R., Dunne, J. P., Gehlen, M., Ilyina, T., John, J. G., Lenton, A., Li, H., Lovenduski, N. S., Orr, J. C., Palmieri, J., Santana-Falcón, Y., Schwinger, J., Séférian, R., Stock, C. A., Tagliabue, A., Takano, Y., Tjiputra, J., Toyama, K., Tsujino, H., Watanabe, M., Yamamoto, A., Yool, A., and Ziehn, T.: Twenty-first century ocean warming, acidification, deoxygenation, and upper-ocean nutrient and primary production decline from CMIP6 model projections, Biogeosciences, 17, 3439–3470, https://doi.org/10.5194/bg-17-3439-2020, 2020.
McClanahan, T. R., Weil, E., Cortés, J., Baird, A. H., and Ateweberhan, M.: Consequences of coral bleaching for sessile reef organisms, in: Coral Bleaching, edited by: van Oppen, M. J. and Lough, J. M., Springer, Berlin, Heidelberg, 121–138, https://doi.org/10.1007/978-3-540-69775-6_8, 2009.
Movilla, J., Calvo, E., Coma, R., Serrano, E., López-Sanz, À., and Pelejero, C.: Annual response of two Mediterranean azooxanthellate temperate corals to low-pH and high-temperature conditions, Mar. Biol., 163, 135, https://doi.org/10.1007/s00227-016-2908-9, 2016.
Nakamura, M., Ohki, S., Suzuki, A., and Sakai, K.: Coral larvae under ocean acidification: survival, metabolism, and metamorphosis, PLoS One, 6, e14521, https://doi.org/10.1371/journal.pone.0014521, 2011.
Nozawa, Y. and Harrison, P. L.: Effects of elevated temperature on larval settlement and post-settlement survival in scleractinian corals, Acropora solitaryensis and Favites chinensis, Mar. Biol., 152, 1181–1185, https://doi.org/10.1007/s00227-007-0765-2, 2007.
O'Connor, M. I., Bruno, J. F., Gaines, S. D., Halpern, B. S., Lester, S. E., Kinlan, B. P., and Weiss, J. M.: Temperature control of larval dispersal and the implications for marine ecology, evolution, and conservation, P. Natl. Acad. Sci. USA, 104, 1266–1271, https://doi.org/10.1073/pnas.0603422104, 2007.
Orr, J. C., Fabry, V. J., Aumont, O., Bopp, L., Doney, S. C., Feely, R. A., Gnanadesikan, A., Gruber, N., Ishida, A., Joos, F., Key, R. M., Lindsay, K., Maier-Reimer, E., Matear, R., Monfray, P., Mouchet, A., Najjar, R. G., Plattner, G.-K., Rodgers, K. B., Sabine, C. L., Sarmiento, J. L., Schlitzer, R., Slater, R. D., Totterdell, I. J., Weirig, M.-F., Yamanaka, Y., and Yool, A.: Anthropogenic ocean acidification over the twenty-first century and its impact on calcifying organisms, Nature, 437, 681–686, https://doi.org/10.1038/nature04095, 2005.
Randall, C. J. and Szmant, A. M.: Elevated temperature reduces survivorship and settlement of the larvae of the Caribbean scleractinian coral, Favia fragum (Esper), Coral Reefs, 28, 537–545, https://doi.org/10.1007/s00338-009-0482-z, 2009.
Ritson-Williams, R., Arnold, S., Fogarty, N., Steneck, R. S., Vermeij, M., and Paul, V. J.: New perspectives on ecological mechanisms affecting coral recruitment on reefs, Smithson. Contrib. Mar. Sci., 437–457, https://doi.org/10.5479/si.01960768.38.437, 2009.
Rodolfo-Metalpa, R., Houlbrèque, F., Tambutté, É., Boisson, F., Baggini, C., Patti, F. P., Jeffree, R., Fine, M., Foggo, A., Gattuso, J.-P., and Hall-Spencer, J. M.: Coral and mollusc resistance to ocean acidification adversely affected by warming, Nat. Clim. Change, 1, 308–312, https://doi.org/10.1038/nclimate1200, 2011.
Sully, S., Burkepile, D. E., Donovan, M. K., Hodgson, G., and van Woesik, R.: A global analysis of coral bleaching over the past two decades, Nat. Commun., 10, 1264, https://doi.org/10.1038/s41467-019-09238-2, 2019.
Suwa, R., Nakamura, M., Morita, M., Shimada, K., Iguchi, A., Sakai, K., and Suzuki, A.: Effects of acidified seawater on early life stages of scleractinian corals (Genus Acropora), Fish. Sci., 76, 93–99, https://doi.org/10.1007/s12562-009-0189-7, 2010.
Teixidó, N., Caroselli, E., Alliouane, S., Ceccarelli, C., Comeau, S., Gattuso, J., Fici, P., Micheli, F., Mirasole, A., Monismith, S. G., Munari, M., Palumbi, S. R., Sheets, E., Urbini, L., De Vittor, C., Goffredo, S., and Gambi, M. C.: Ocean acidification causes variable trait-shifts in a coral species, Global Change Biol., 26, 6813–6830, https://doi.org/10.1111/gcb.15372, 2020.
Varnerin, B., Hopkinson, B., and Gleason, D.: Recruits of the temperate coral Oculina arbuscula mimic adults in their resilience to ocean acidification, Mar. Ecol. Prog. Ser., 636, 63–75, https://doi.org/10.3354/meps13228, 2020.
Woolsey, E., Byrne, M., and Baird, A.: The effects of temperature on embryonic development and larval survival in two scleractinian corals, Mar. Ecol. Prog. Ser., 493, 179–184, https://doi.org/10.3354/meps10499, 2013.
Zibrowius, H.: The “Southern” Astroides calycularis in the Pleistocene of the northern Mediterranean – An indicator of climatic changes (Cnidaria, scleractinia), Geobios, 28, 9–16, https://doi.org/10.1016/S0016-6995(95)80201-0, 1995.