the Creative Commons Attribution 4.0 License.
the Creative Commons Attribution 4.0 License.
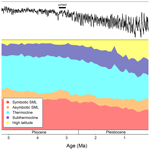
Paleoecology and evolutionary response of planktonic foraminifera to the mid-Pliocene Warm Period and Plio-Pleistocene bipolar ice sheet expansion
Adam Woodhouse
Frances A. Procter
Sophie L. Jackson
Robert A. Jamieson
Robert J. Newton
Philip F. Sexton
Tracy Aze
The Pliocene-Recent is associated with many important climatic and paleoceanographic changes, which have shaped the biotic and abiotic nature of the modern world. The closure of the Central American Seaway and the development and intensification of Northern Hemisphere ice sheets had profound global impacts on the latitudinal and vertical structure of the oceans, triggering the extinction and radiation of many marine groups. In particular, marine calcifying planktonic foraminifera, which are highly sensitive to water column structure, exhibited a series of extinctions as global temperatures fell. By analyzing high-resolution (∼ 5 kyr) sedimentary records from the Eastern Equatorial Pacific Ocean, complemented with global records from the novel Triton dataset, we document the biotic changes in this microfossil group, within which three species displayed isochronous co-extinction, and species with cold-water affinity increased in dominance as meridional temperature gradients steepened. We suggest that these changes were associated with the terminal stages of the closure of the Central American Seaway, where following the sustained warmth of the mid-Pliocene Warm Period, bipolar ice sheet expansion initiated a world in which cold- and deep-dwelling species became increasingly more successful. Such global-scale paleoecological and macroevolutionary variations between the Pliocene and the modern icehouse climate would suggest significant deviations from pre-industrial baselines within modern and future marine plankton communities as anthropogenic climate forcing continues.
- Article
(5274 KB) - Full-text XML
-
Supplement
(1862 KB) - BibTeX
- EndNote
Current observations suggest that the Earth is shifting from its pre-industrial state (Beaugrand et al., 2002; Cheung et al., 2013; Ceballos et al., 2015; Urban, 2015; Barton et al., 2016; Pinksy et al., 2018; Jonkers et al., 2019; Tierney et al. 2020; Edwards et al., 2022), and changes in the vertical and latitudinal structure of the marine realm are ultimately likely to take place (Hu et al., 2011; Rhein et al., 2013; Purich et al., 2018; Zika et al., 2018; Bindoff et al., 2019; Golledge et al., 2019). It is therefore important to understand how ecosystems such as the open ocean, which contain resources vital to human populations (Worm et al., 2003; Tittensor et al., 2010), respond to short- and long-term oceanographic shifts (e.g., Norris et al., 2013).
The timing of closure for the Isthmus of Panama at ∼ 4.7–2.7 Ma (Keigwin, 1978, 1982; Keller et al., 1989; Haug and Tiedemann, 1998; Haug et al., 2001; Groeneveld et al., 2006; Steph et al., 2006, 2010; Molnar, 2008; Fedorov et al., 2013; O'Dea et al., 2016) remains contentious, though the ultimate role of this event in late Cenozoic icehouse evolution is clear, proving to be pivotal to the intensification of Northern Hemisphere glaciations. The precise dating of the final isthmus formation is less essential than the repercussions of the gradual shoaling and restriction of the Central American Seaway (CAS), which triggered significant global paleoceanographic effects fundamental to the evolution of the present climate state. The gradual restriction of consistent throughflow between the tropical Atlantic and Indo-Pacific Oceans via isthmus formation (O'Dea et al., 2016) was coupled with bipolar cryosphere development that significantly restructured global vertical and meridional temperature gradients (Schmidt et al., 2004a, b; Mudelsee and Raymo, 2005; Boscolo-Galazzo and Crichton et al., 2021; Boscolo-Galazzo et al., 2022; Ford et al., 2022; Gaskell et al., 2022), altering marine ecosystems and trophic structure (Woodhouse and Swain et al., 2023). The recorded effects on global paleoceanography and biodiversity are of particular importance, as this ice sheet expansion was preceded by the sustainedly warmer world of the Pliocene. In particular, the mid-Pliocene Warm Period (mPWP, 3.264–3.025; Dowsett et al., 2012; Haywood et al., 2016) is increasingly imperative to our understanding of future climate change as global continental configuration, faunal and floral distributions, mean global temperatures (2–3 ∘C warmer than pre-industrial), and pCO2 (350–450 ppm) were comparable to levels expected by the closure of the twenty-first century (Chandler et al., 1994; Haywood et al., 2000, 2016; Jiang et al., 2005; Pagani et al., 2010; Seki et al., 2010; Bartoli et al., 2011; IPCC, 2022).
To assess the biological responses to these marine ecosystem changes, we look to the Cenozoic marine microfossil record, specifically the planktonic foraminifera, single-celled marine protists with a global distribution and the most complete Cenozoic species-level fossil record (Aze et al., 2011; Fenton and Woodhouse et al., 2021). Their calcareous skeletons, or tests, preserve not only their entire life history, but also a biogeochemical expression of the surrounding water column (e.g., Edgar et al., 2017). These features allow for high-resolution species-specific quantification of physiological and ecological adaptation through periods of climate variability (e.g., Knappertsbusch, 2007; Wade et al., 2008, 2016; Hull and Norris, 2009; Wade and Olsson, 2009; Edgar et al., 2013a; Aze et al., 2014; Pearson and Ezard, 2014; Weinkauf et al., 2014, 2019; Brombacher et al., 2017a, 2021; Falzoni et al., 2018; Si and Aubry, 2018; Fox et al., 2020; Todd et al., 2020; Kearns et al., 2021, 2022; Pearson and Penny, 2021; Shaw et al., 2021; Woodhouse et al., 2021; Friesenhagen, 2022; Hupp et al., 2022; Woodhouse and Swain et al., 2023).
Here, we report the high-resolution biotic response of planktonic foraminifera during the terminal stages of closure of the CAS in the Eastern Equatorial Pacific (EEP) Ocean, focusing on the co-extinction of three members of the genus Dentoglobigerina through documentation of high-resolution (∼ 5 kyr) paired single-specimen morphometric, with multi- and single-specimen geochemical analyses, and their paleoceanographic implications. Moreover, we assess the global paleoecological response of planktonic foraminifera from the Pliocene to the Recent, assessing the role of the transition from the sustained warmth of the Pliocene to the bipolar icehouse world of Pleistocene-Recent on global planktonic foraminiferal macroevolutionary dynamics.
2.1 Site selection
The integrated Ocean Drilling Program Expedition 321 Site U1338 (Hole 1338A) (2∘30.469′ N, 17∘58.162′ W), situated in the EEP, was drilled to 410 m below seafloor (mbsf) through Holocene – early Miocene pelagic sediments (Pälike et al., 2010). At ∼ 3 Ma, the site was at ∼ 2∘ N paleolatitude (Drury et al., 2014) in a deep-water pelagic environment of similar water depth to the modern (∼ 4 km). The primary lithologies represented are calcareous, diatomaceous and radiolarian nannofossil oozes and chalks. Despite the deep-water settings, and primarily calcareous nature of the sediments, excellent microfossil preservation has been recorded in planktonic foraminiferal specimens through intervals of this core (Fox and Wade, 2013; Woodhouse et al., 2021). A preliminary assessment of core U1338A was carried out to determine the approximate position of the extinction of several species of Dentoglobigerina (∼ 3 Ma) based on tropical biostratigraphy (Wade et al., 2011), and shipboard paleomagnetic data (Pälike et al., 2010).
2.2 Foraminiferal assemblage analysis
Sediment volumes of 20–40 cm3 were collected and washed with de-ionized water over a 63 µm sieve; the residues were dried in an oven at 40 ∘C and split. All samples were examined using a Zeiss Stemi 305 Compact Stereo Microscope. Planktonic foraminifers were identified following the taxonomy of Kennett and Srinivasan (1983), Schiebel and Hemleben (2017), and Wade et al. (2018). We performed assemblage counts on >63 µm splits yielding >300 individuals (Table S1 in the Supplement). Assessments of taxonomy and test preservation of foraminifera were performed via analysis with the Tescan VEGA3 XM Scanning Electron Microscope (SEM) at the University of Leeds, UK.
Species were grouped by their ecology to assess the relative abundances of taxa for paleoceanographic information, where taxa were assigned as either shallow- and warm-water taxa (the ecogroups “symbiotic” and “asymbiotic” of Aze et al., 2011), or deep- and cold-water taxa (the ecogroups “thermocline”, “subthermocline” of Aze et al., 2011, and Globigerinita glutinata, Lutz, 2010).
2.3 Morphometric analysis
Specimens of the genus Dentoglobigerina were measured to compare species size with stable isotope ratios to investigate species ecology and ontogeny. Complete specimens of Dentoglobigerina were picked and mounted in umbilical position on card slides pierced with a fine needle to accommodate the variably spired nature of species in the genus (Wade et al., 2018). Specimens were imaged umbilically using a Zeiss Axio Zoom V16 microscope with attached Canon EOS 100D camera at ×19.4 magnification. All specimens were then rotated 90∘ laterally and imaged whilst propped onto their penultimate chamber. Images were processed using the image analysis software Image Pro Premier, and the maximum test diameter, previously deemed a statistically repeatable measurement amongst Dentoglobigerina (Brombacher et al., 2017b, 2018; Woodhouse et al., 2021), was captured from both orientations, and the lower of the two values assigned as test size.
2.4 Geochemical analysis
Following morphometric analysis, well-preserved specimens of Dentoglobigerina altispira (>200 µm), Dentoglobigerina baroemoenensis (>200 µm) and Dentoglobigerina globosa (>200 µm) were picked, ultrasonicated in deionized water for 10–15 s, and dried for stable isotope analysis. This process was repeated for extant taxa representing known discrete ecological habitats through the water column to determine the ecological niche habits of the extinct dentoglobigerinids: Globigerinoides ruber (212–350 µm, surface mixed-layer (SML)), Neogloboquadrina incompta (212–350 µm, subsurface), Globorotalia tumida (>300 µm, thermocline and/or photic zone base, corrected for a 1.0 ‰ δ13C enrichment due to this species occupying the shallow oxygen minimum zone and the consequential effects of reduced ambient pH, Lohmann, 1995; Bijma et al., 1999; Uchikawa and Zeebe, 2010; Birch et al., 2013), Globorotalia scitula (212–300 µm, subthermocline), and Cibicidoides wuellerstorfi (>212 µm, bottom-water) (see Cramer et al., 2009, 2011; Rasmussen and Thomsen, 2010; Aze et al., 2011; Woodhouse et al., 2021). Single specimens of dentoglobigerinids and multiple specimens of other foraminifer species were analyzed using an Elementar IsoPrime Dual-Inlet Isotope Ratio Mass Spectrometer in the School of Earth and Environment at the University of Leeds, and data were reported to the Vienna Peedee belemnite (VPDB) scale using a Carrara marble standard (Elemental Microanalysis B2214), where analytical precision was better than 0.07 ‰ and 0.13 ‰ for δ13C and δ18O, respectively.
Specimens of Cibicidoides wuellerstorfi were also used to create a benthic foraminiferal δ18O record for this study to supplement the shipboard paleomagnetic data (Pälike et al., 2010). This record was constructed and tuned to the Ocean Drilling Program Site 849/IODP Site 1338 stack constructed by Lyle et al. (2019) using QAnalySeries software (Kotov and Pälike 2018) to better constrain the timing of events (see Woodhouse et al., 2021), where the study section is calculated to represent the Pliocene interval from ∼ 3.47–2.98 Ma.
2.5 Global data analysis
To investigate how planktonic foraminiferal macroevolution and paleoecology has developed since the Pliocene, the Triton dataset (Fenton and Woodhouse et al., 2021) was downloaded, and all macroperforate planktonic foraminiferal records occurring from 5.3–0 Ma (early Pliocene-Recent) were binned into 53-time bins with equal length (100 kyrs). Species were assigned the speciation and extinction datums in accordance with Aze et al. (2011) and Fenton and Woodhouse et al. (2021), and all species occurrences located outside of these assigned stratigraphic ranges were removed. This range trimming was applied to eliminate much of the occurrence data likely attributable to misidentification and/or reworking that may create artificial “tails” within speciation and extinction data (Liow et al., 2010; Lazarus et al., 2012; Flannery-Sutherland et al., 2022). The trimming of taxa resulted in a dataset of 239 317 planktonic foraminiferal occurrences. Furthermore, all species were assigned to their respective “ecogroups”, which represent broad ecological categories based on paleoecological and phylogenetic data (Aze et al., 2011). These ecogroups are defined as: ecogroup 1 = surface mixed layer dweller with photosymbionts, ecogroup 2 = surface mixed layer dweller without photosymbionts, ecogroup 3 = thermocline dweller, and ecogroup 4 = subthermocline dweller, ecogroup 5 = high-latitude.
3.1 Assemblage records
All sediments contain a highly abundant well-preserved (Fig. 1) open-ocean planktonic foraminifer assemblage comprising ∼ 70 morphospecies. The dominant genera through the section included Neogloboquadrina, Globigerinoides, Pulleniatina, and Globigerinita (see Supplement). The isochronous extinction of the species D. altispira, D. baroemoenensis, and D. globosa in U1338A occurs ∼ 35.50 m b.s.f. (∼ 3.037 Ma). There is also a notable influx of the species Menardella cf. exilis and Menardella cf. pertenuis occurring from ∼ 42.58–40.56 m b.s.f. (∼ 3.45–3.36 Ma), after which they are absent within the study section.
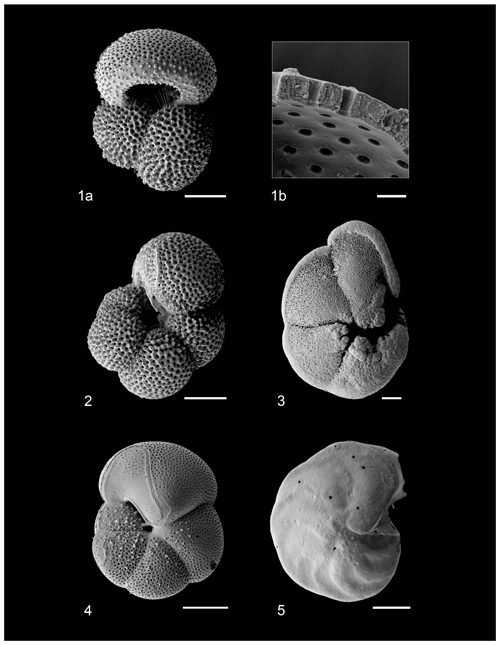
Figure 1Scanning electron micrographs of species analyzed for stable isotope geochemistry. (1a) G. ruber, (1b) G. ruber shell ultrastructure showing excellent preservation, (2) N. incompta, (3) G. tumida, (4) H. scitula, (5) C. wuellerstorfi. Scale bar for images (1a), 2–5 = 100 µm, for image (1b) = 10 µm. All specimens from sample U1338A-5H-4W-35/38.
Comparing the assemblage composition of warm and surface dwellers against cold and deep dwellers, we document generally consistent species abundances until ∼ 36.26 m b.s.f. (∼ 3.08 Ma), where the assemblage exhibits consistently greater abundances of cold and deep dwellers for the rest of the record (Fig. 2).
3.2 Dentoglobigerina stable isotope-size trends
Test preservation is excellent throughout the sampled interval, where specimen walls appear optically translucent, and SEM images (Fig. 1) indicate no observable diagenetic alteration, clean pore spaces, and spines preserved within tests (Fig. 1a). Following artificial test breaking, inspection of the wall ultrastructure (Fig. 1b) showed that no wall recrystallization had taken place, suggesting stable isotope signals remain unaltered (Sexton et al., 2006; Edgar et al., 2013b). Single-specimen δ13C values for D. altispira, D. baroemoenensis, and D. globosa vary from −0.54 ‰ to +3.53 ‰, +0.30 ‰ to +3.50 ‰, and +0.51 ‰ to +2.84 ‰ (Fig. 3, Tables S3 and S4), respectively, whilst δ18O values vary from −3.56 ‰ to −0.03 ‰, −2.99 ‰ to −0.17 ‰, and −1.92 ‰ to −1.05 ‰, respectively. In all three species, a positive correlation is calculated between test size and δ13C; however, this relationship is only significant (p<0.0001) in D. altispira and D. baroemoenensis, potentially due to the greater number of specimens analyzed for these species (Fig. 3). Regression slopes are similarly steep; however the slopes for D. altispira and D. globosa are more alike. Dentoglobigerina baroemoenensis and D. globosa show no significant correlation between δ18O and test size; however, a significant (p = 0.0006) negative relationship is recorded in D. altispira. Once again, regression slopes for D. altispira and D. globosa are similar, whereas for D. baroemoenensis, this slope is almost flat (Fig. 3).
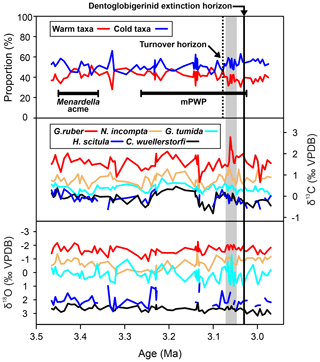
Figure 2Grouped assemblage data of warm-water taxa (ecogroups “symbiotic” and “asymbiotic”) and cold-water taxa (ecogroups “thermocline”, “subthermocline”, and Globigerinita glutinata (Lutz, 2010; Aze et al., 2011). Foraminiferal δ13C and δ18O, black = C. wuellerstorfi (bottom water), dark blue = G. scitula (subthermocline), cyan = G. tumida (thermocline), orange = N. incompta (subsurface), red = G. ruber (surface mixed-layer). The dashed line represents permanent switch to higher proportion of cold-water taxa, and the gray box indicates where isotope records exhibit high volatility, mPWP = mid-Pliocene Warm Period.
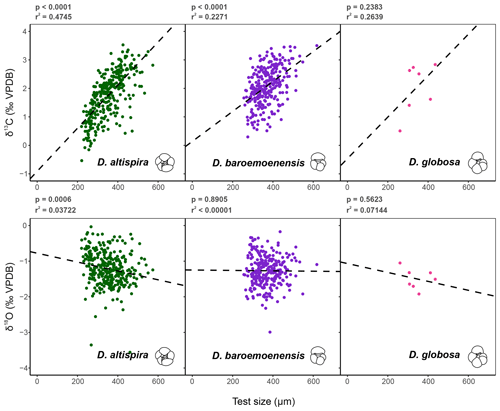
Figure 3Test size – δ13C and δ18O relationships for the three species of Dentoglobigerina that underwent extinction through the study section. Trend lines for each species represent linear regressions based on the entire per species dataset (dashed line). Significance (p) and measure of fit (r2) were calculated for each linear regression.
3.3 Extant species geochemical records
Amongst the extant species picked from strict size fractions throughout the study section, G. ruber δ13C values show the highest δ13C variability (+0.7 ‰ to +2.8 ‰) and δ18O values varying from −2.3 ‰ to −0.8 ‰ (Fig. 4, Table S2). Corrected G. tumida values show the lowest δ13C variability (0 ‰ to +0.8 ‰), and the highest variability for δ18O (-1.3 ‰ to +1.1 ‰). G. scitula δ13C values vary from −0.6 ‰ to +0.4 ‰, whilst δ18O varies from +0.8 ‰ to +2.9 ‰, whereas bottom-water C. wuellerstorfi δ13C values vary from −0.8 ‰ to +0.5 ‰, and δ18O varies +2.2 ‰ to +3.1 ‰, showing the lowest variability in δ18O (Fig. 4). The stable isotope records of extant species generally tend to show low inter-sample variability, however there is notable geochemical stochasticity within the G. tumida record ∼ 36.17–35.86 m b.s.f. (Fig. 2).
All three species of Dentoglobigerina analyzed in this study record mean stable isotopic ratios similar to the extant species G. ruber, where all species indicated δ18O ratios less negative than G. ruber, and D. baroemoenensis and D. globosa show δ13C ratios more positive than this species (Figs. 3 and 4).
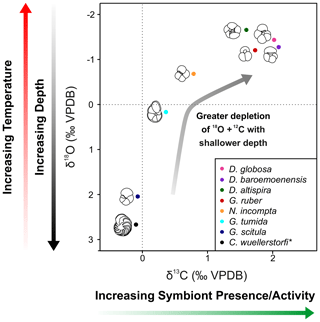
Figure 4δ13C and δ18O cross-plot 2 showing mean values of all specimens analyzed in this study. Black = C. wuellerstorfi, dark blue = G. scitula, cyan = G. tumida, orange = N. incompta, red = G. ruber, green = D. altispira, purple = D. baroemoenensis, pink = D. globosa. Species marked with an asterisk are benthic.
3.4 Global Pliocene-Recent Ecogroup trends
The global proportions of planktonic foraminiferal ecogroups within the Triton dataset (Fenton and Woodhouse et al., 2021) show that throughout the time interval studied (5.3–0 Ma), surface mixed layer dwellers without photosymbionts (orange) proportions remain relatively consistent, making up ∼ 10 % of the total global fauna (Fig. 5). From 5.3–3 Ma, global ecogroup proportions are relatively consistent, where the dominant forms are thermocline dwellers (cyan) and surface mixed layer dwellers with photosymbionts (red) (Fig. 5), making up ∼ 40 % and ∼ 35 % of the global fauna, respectively. During this interval, the proportion of subthermocline dwellers (blue) and high-latitude forms (yellow) also remain relatively consistent with ∼ 10 % and <5 % of total proportions, respectively. After ∼ 3 Ma, both thermocline dwellers and surface mixed layer dwellers with photosymbionts show a steady decline in global representation approaching the Recent, making up ∼ 20 % each in the modern ocean. At ∼ 3 Ma, both the subthermocline dwellers and high- latitude ecogroup start to show increases in their proportions, though this increase approaching the modern is much greater in the latter group. During the last 100 kyrs, subthermocline and high- latitude species have constituted ∼ 15 % and ∼ 25 %, respectively (Fig. 5).
Few major changes in the assemblage composition are observed through the study interval, other than the isochronous extinction of D. altispira, D. baroemoenensis, and D. globosa at ∼ 3.037 Ma (Fig. 2; 35.50 m b.s.f.), and the influx of the two species M. cf. exilis and M. cf. pertenuis through ∼ 3.45–3.36 Ma (42.58–40.56 m b.s.f.), defined henceforth as the “Menardella acme” (Figs. 2 and 6).
4.1 Dentoglobigerinid paleoecology
The extinction of D. altispira is a useful marker in the mid-Pliocene, previously recorded within the East Equatorial Pacific Ocean (3.46 Ma; Shackleton et al., 1995; Wade et al., 2011); however, this study and that of Woodhouse et al. (2021) provide recalibration for this event, which also includes the co-extinction of D. baroemoenensis and D. globosa. The co-extinction of the dentoglobigerinids is significant as Woodhouse et al. (2021) demonstrated that D. altispira and D. baroemoenensis show unique phenotypic responses leading up to their termination, despite their shared phylogenetic and ecological affinity. We, therefore, suggest that all three species share an ecological habit that ultimately proved inefficient to mitigate the changing abiotic conditions associated with this critical period of bipolar cryosphere development (Kleiven et al., 2002; Brierley and Fedorov, 2010; Cramer et al., 2009, 2011; Willeit et al., 2015; Hayashi et al., 2020; Westerhold et al., 2020).
Studies of extant species suggest a strong positive correlation between planktonic foraminifera δ13C and test size, paired with a lack of a strong negative significant relationship in δ18O and test size, is indicative of species bearing photosymbiotic algae (e.g., Berger et al., 1978; Bouvier-Soumagnac and Duplessey, 1985; Spero and Williams, 1988, 1989; Spero et al., 1991; Spero, 1992; Spero and Lea, 1993; Ravelo and Fairbanks, 1995; Norris, 1996; Birch et al., 2013). Our data suggests that all three species of Dentoglobigerina studied here are symbiont-bearing, displaying mean stable isotope data similarly to the symbiont-bearing surface mixed layer dwelling G. ruber (Figs. 2–4). Moreover, the regression lines for the ancestor-descendent pair D. globosa – D. altispira are remarkably similar, suggesting that the species share similar stable isotope-test size relationships, despite the speciation of D. altispira occurring ∼ 20 Myrs prior (Aze et al., 2011; Wade et al., 2011, 2018). However, the substantially fewer data for D. globosa currently limits the significance of this observation for this particular species (Fig. 3).
The results presented here contribute to the variety of interpretations from previous studies on the paleoecology of D. altispira. Spanning different ocean basins throughout the species' stratigraphic range they suggest: a shallow-dwelling (e.g., Keller and Savin, 1985; Vincent et al., 1985; Prentice and Matthews, 1988; Corfield and Cartlidge, 1991; Norris et al., 1993; Hodell and Vayavananda, 1994; Sosdian and Lear, 2020), deep-dwelling (Opdyke and Pearson, 1995; Pearson and Shackleton, 1995), or lower mixed layer/upper thermocline (Zou et al., 2022) ecological niche habit.
Woodhouse et al. (2021) suggested that prior to extinction, the D. altispira geochemical signal may signify that this species underwent adaptive ecological niche migration across multiple depth habitats. Upon further investigation, by isolating all D. altispira specimens present after the migration horizon at ∼ 3.061 Ma (Woodhouse et al., 2021), we show that these specimens do, in fact, display a steeper negative relationship between δ18O and test size (Fig. S1), consistent with asymbiosis; however, this relationship lacks statistical significance. Nevertheless, this observation lends support to the conclusions of Woodhouse et al. (2021) that this species underwent niche adaptation prior to extinction.
Another plausible scenario may be the existence of indeterminate cryptic diversity within the D. altispira morphospecies complex where multiple ecological strategies or phylogenetic expressions may be present (e.g., Huber et al., 1997; Bijma et al., 1998; de Vargas et al., 1999, 2002; Weiner et al., 2012; Schiebel and Hemleben, 2017; Nirmal et al., 2021; Pearson and Penny, 2021), and the stepwise changes observed by Woodhouse et al. (2021) may indicate the systematic loss of “cryptic genotypes” within this morphospecies complex. Indeed, Pearson and Penny (2021), hypothesized that dramatic abundance switches in the Indo-Pacific Warm Pool of ecologically distinct, alternately coiled populations of Pulleniatina morphospecies may signify replacement by distinct cryptic genotypes, and such coiling switches are noted throughout the planktonic foraminiferal fossil record (Ericson et al., 1955; Saito et al., 1975; Bossio et al., 1976; Hallock and Larsen, 1979; Hornibrook, 1982; Scott et al., 1990; Norris and Nishi, 2001; Winter and Pearson, 2001; Crundwell and Nelson, 2007; Wade et al., 2011; Pearson and Ezard, 2014; Crundwell, 2015a, b; Levin et al., 2016; Wallace et al., 2019; Crundwell and Woodhouse, 2022a, b). Therefore, the range of interpreted paleoecologies in D. altispira may, in fact, be due to the occurrence of distinct cryptic populations from across the geological record. Irrespectively, the abiotic conditions at this time appear to have become fatally detrimental to the entire ecological habit of all three morphospecies of Dentoglobigerina analyzed in the EEP, and subsequently across the entire globe (Shackleton et al., 1995; Chaisson and Pearson, 1997; Wade et al., 2011; Raffi et al., 2020; Groeneveld et al., 2021; Woodhouse et al., 2021).
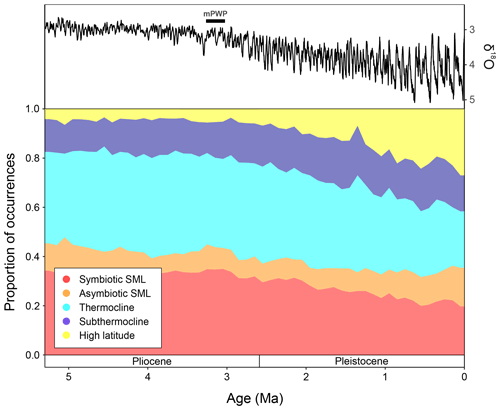
Figure 5The LR04 stack showing benthic foraminiferal δ18O (from Lisiecki and Raymo, 2005) and proportions of macroperforate planktonic foraminifera occurrences assigned to the ecogroups of Aze et al. (2011), binned to 100 kyrs from 5.3 Ma to the Recent. mPWP = mid-Pliocene Warm Period, SML = surface mixed-layer.
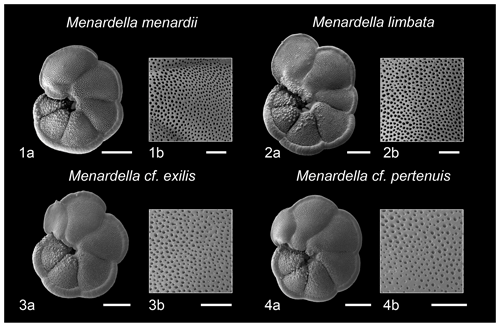
Figure 6Scanning electron micrographs of select species of Menardella and pore detail of penultimate chambers. (1a) M. menardii, (1b) M. menardii pore detail, (2a) M. limbata, (2b) M. limbata pore detail, (3a) M. cf. exilis, (3b) M. cf. exilis pore detail, (4a) M. cf. pertenuis, (4) M. cf. pertenuis pore detail. Scale bar for images (1a), (2a), (3a), (4a) = 200 microns, for image (1b), (2b), (3b), (4b) = 50 µm. Specimens 1 and 2 from sample U1338A-5H-CC-11/14 and specimens 3 and 4 from sample 1338A-5H-7W-76-79.
4.2 The Menardella acme
The transient influx and co-occurrence of M. cf. exilis and M. cf. pertenuis from ∼ 3.45–3.36 Ma may indicate a shared ecological affinity between these species (Fig. 2; Kennett and Srinivasan, 1983; Knappertsbusch, 2016). Furthermore, this association may suggest that these two similar forms exist within the same genetic species complex, though further study on internal shell ontogeny and external morphology would be required to confirm this hypothesis. Notably, however, Kaneps (1970) and Chaisson (2003) suggested that Indo-Pacific occurrences of these two species are, in fact, “aberrant” forms of Menardella limbata and Menardella menardii, with the M. exilis-pertenuis plexus being solely endemic to the Atlantic basin (Sexton and Norris, 2011).
Scanning election photomicrographs and pore density analysis of Menardella morphospecies within this study (Fig. 6; Table 1) suggests that the M. cf. exilis and M. cf. pertenuis morphotypes ascribed to “aberrant” forms may, in fact, be phylogenetically distinct from both M. menardii and M. limbata, as well as from the endemic Atlantic M. exilis-pertenuis plexus. Where the Atlantic type specimens exhibit finely perforate tests (Kennett and Srinivasan, 1983), the specimens within this study appear to show test perforation intermediate between “normally perforate” menardellids such as M. menardii and M. limbata (Fig. 6), and the “finely perforate” M. exilis-pertenuis plexus (Kennett and Srinivasan, 1983; Chaisson, 2003). Whether all Indo-Pacific occurrences of these morphotypes (e.g., Brönniman and Resig, 1971; Jenkins and Orr, 1972; Thunell, 1981; Keigwin, 1982; Thompson, 1982; Chaisson and Leckie, 1993) exhibit similar test perforation remains to be determined. However, these forms could represent either: (1) convergent evolution of a geographically isolated endemic population restricted to the Indo-Pacific triggered by vicariance, or (2) specimens within the M. exilis-pertenuis plexus exhibiting a differential phenotypic expression of pore density, potentially due to regional differences in paleoceanography between the Atlantic and Indo-Pacific basins at this time (Haug et al., 2001). Irrespective of the biological implications, this acme event may represent a regionally valuable biostratigraphic marker horizon.
4.3 Pliocene EEP paleoceanography
Comparing the assemblage composition of warm and surface dwellers against cold and deep dwellers, we document a gradual turnover from an assemblage exhibiting relatively even abundances of these two groups to one of increasing occupation of cold and deep dwellers from ∼ 3.08 Ma onwards (36.26 m b.s.f.; Fig. 2). This may reflect regional thermocline shoaling similar to patterns observed at DSDP Site 84 in the proximal Panama Basin (Lutz, 2010). Other geological intervals associated with significant global cooling such as the Eocene-Oligocene transition and late Miocene cooling exhibit similar patterns of gradual, successive turnover of warm-water dwellers by cold-water taxa potentially associated with contractions and expansions in their respective ecospaces (Keller, 1983; Boersma and Premoli Silva, 1991; Keller et al., 1992; Molina et al., 1993; Wade and Pearson, 2008; Ezard et al., 2011; Lowery and Fraass, 2019; Lowery et al., 2020; Boscolo-Galazzo and Crichton et al., 2021; Boscolo-Galazzo et al., 2022; Woodhouse and Swain et al., 2023).
The establishment of the Isthmus of Panama played a key role in Atlantic Meridional Overturning Circulation intensification by barring Caribbean inflow from the Atlantic (O'Dea et al., 2016; Hayashi et al., 2020), leading to a new paleoceanographic state defined by an equatorial Pacific thermocline exhibiting a high-angle east–west tilt (Fiedler and Talley, 2006; Yang and Wang, 2009; Zhang et al., 2012; Ford et al., 2015). Changes in the EEP water column structure are compounded within biotic and abiotic records, where global changes in ice volume, as shown by benthic δ18O (Fig. 5), and the formation of the isthmus contributed to the gradual cooling and shoaling of the thermocline (Ford et al., 2015). Isochronous records of ice-rafted debris in the North Atlantic and Pacific infer a substantial change to high-latitude global climate ∼ 3 Ma (Shackleton et al., 1984; Maslin et al., 1996; Kleiven et al., 2002; Lawrence et al., 2006). Consequently, the already restricted CAS was likely subjected to substantial eustatic sea level fluctuations (Chaisson, 2003; Bartoli et al., 2005; O'Dea et al., 2016), causing cool water within or below the thermocline to become shallow enough to be mixed by surface winds (Philander and Fedorov, 2003; Fedorov et al., 2004, 2006; Ford et al., 2012, 2015). In turn, this contributed to a dramatic increase in regional upwelling associated with further development of the EEP cold tongue (Herbert et al., 2010). We suggest that the increasing dominance of cold and deep dwellers in the EEP (Figs. 2 and 5) are a direct manifestation of the water column structure changes associated with closure of the CAS and cryosphere intensification, marking the initiation of transition from the early Pliocene “El Padre” mean state to one more similar to the modern ocean (Seki et al., 2012; Ford et al., 2012, 2015).
The geochemical fluctuations noted within G. tumida from 3.071–3.061 Ma (36.17–35.86 m b.s.f.; Fig. 2) are coeval with the interval assigned to the “phase transition” by Woodhouse et al. (2021), in which D. altispira and D. baroemoenensis exhibit dramatic fluctuations in size and shape preceding rapid ecological changes. Here, the δ18O signal of G. tumida exhibits substantial and rapid changes up to ∼ 2.1 ‰, switching from typical thermocline values to those indicative of the subsurface, and back again, whereas all other water column δ18O value changes are nominal (Fig. 2).
If the fluctuations within the δ18O signal of G. tumida were related to fluctuations in its depth habitat, we would expect the inverse pattern to occur in its δ13C signature, as δ13C values decrease with depth (Cannariato and Ravelo, 1997; Ford et al., 2012; Birch et al., 2013); however, this is not the case (Fig. 2, Table S2). The maximum δ18O excursion value (∼ 2.1 ‰) is equivalent to a temperature change of ∼ 10 ∘C, based on a mid-Pliocene SMOW value of −0.3 ‰ (Williams et al., 2005; Medina-Elizalde, Lea and Fantle, 2008; Tindall and Haywood, 2015) and the paleotemperature equations of Kim and O'Neil (1997). Previous studies from EEP sites show Mg Ca-derived thermocline temperature fluctuations of ∼ 3 ∘C (Site 1241; Steph et al., 2006) to ∼ 5 ∘C (Sites 848, 849, and 853; Ford et al., 2012) across the same interval, and modern ocean seasonal deviations within EEP surface waters are minor at ± 1 ∘C (Fiedler, 1992). However, these can be intensified by El Niño conditions by up to +3.8 ∘C (Pérez-Angel and Molnar, 2017).
Modern regional evaporation–precipitation balance within the EEP also shows very little variation, wherein δ18Osw values average 0.26 ‰ (Fairbanks et al., 1992), ranging from ∼ 0.2 ‰–0.5 ‰ for the entire tropical Pacific (Ravelo and Hillaire-Marcel, 2007). Factors other than temperature and salinity (e.g., chlorophyll a, the lunar cycle) that trigger species-specific habit changes (Rebotim et al., 2017) could be at least partially responsible for these dramatic changes in the G. tumida δ18O records; however, this species is known to calcify at the base of the photic zone (Ravelo and Shackleton, 1995), irrespective of thermocline depth (Ravelo and Fairbanks, 1992; Rincon-Martinez et al., 2011).
Despite the present low range in Pacific equatorial δ18Osw values, modern Atlantic-Caribbean surface waters record a salinity value ∼ 3 ‰ less than the modern EEP (Haug et al., 2001; Garcia et al., 2006; Schmidt et al., 2016; Öğretmen et al., 2020), equating to ∼ 1.5 ‰ lower in mean δ18Osw values (Ravelo and Hillaire-Marcel, 2007). This salinity contrast was fully established by ∼ 4.2 Ma (Haug et al., 2001), and consistent breaching of Atlantic-Caribbean waters over the still-submerged isthmus may have occurred as late as 1.9 Ma (Coates and Obando, 1996; Keller et al., 1989; Schmidt et al., 2016). Therefore, a breaching event is the most probable cause for the substantial variations in the δ18O record of G. tumida from 3.071–3.061 Ma, where this apparently thermocline-restricted (Fig. 2) disturbance may have contributed to disruption of the Dentoglobigerina ecological niche habit at this time (Woodhouse et al., 2021).
4.4 Pliocene-Recent global ecological and evolutionary patterns
It appears that the faunal turnover documented in the EEP at ∼ 3.08 Ma (Fig. 5) may represent a critical point in the global development of the bipolar cryosphere, which typifies the Pleistocene and Holocene (Kleiven et al., 2002; Mudelsee and Raymo, 2005; Brierly and Fedorov, 2010; Cramer et al., 2009, 2011; Willeit et al., 2015; Hayashi et al., 2020; Westerhold et al., 2020). Major Antarctic ice sheet expansion (Shevenell et al., 2004; Holbourn et al., 2015; Frigola et al., 2018; Westerhold et al., 2020) following the Miocene Climatic Optimum (∼ 17–15 Ma; Methner et al., 2020) initiated global cooling, intensifying meridional gradients (Gaskell et al., 2022). This triggered unprecedented mean size increases in low-latitude planktonic foraminifera (Schmidt et al., 2004a, b) and higher efficiency of the biological carbon pump, promoting greater endemism and exploitation of new deep-water niches in calcifying plankton (Olsson, 1982; Scott, 1982; Keller, 1985; Malmgren and Berggren, 1987; Scott et al., 1990; Norris et al., 1993, 1994, 1996; Norris, 1999, 2000; Rögl, 1999; Chaisson, 2003; Kucera and Schönfeld, 2007; Ezard et al., 2011; Crundwell, 2018; Rosenthal et al., 2018; Spezzaferri et al., 2018; Lam and Leckie, 2020; Boscolo-Galazzo and Crichton et al., 2021; Boscolo-Galazzo et al., 2022; Woodhouse and Swain et al., 2023).
From ∼ 6.5 Ma, the equatorial Pacific shows meridionally distinct planktonic foraminiferal faunal provinces that display a turnover in dominance from Miocene species to more Recent taxa ∼ 4 Ma (Chaisson, 1995; Chaisson and Ravelo, 2000) linked with the closure of the CAS (Haug et al., 2001). Global temperatures record a gradual, stable decline towards ∼ 3 Ma (Fig. 5; Westerhold et al., 2020), after which planktonic foraminiferal morphospecies diversity shows a notable decline (Aze et al., 2011; Ezard et al., 2011; Fraass et al., 2015; Lowery et al., 2020), whilst ecogroups (Fig. 5) exhibit the expansion of cold-water forms coincident with the development and intensification of Northern Hemisphere ice sheets (Kleiven et al., 2002; Brierly and Fedorov, 2010; Cramer et al., 2009, 2011; Willeit et al., 2015; Hayashi et al., 2020). The greater proportion of cold and deep dwellers in the EEP at ∼ 3.08 Ma (Fig. 2) coincides with global ecogroup patterns (Fig. 5), signifying the initiation of increasing global dominance of planktonic foraminiferal species with cold-water affinity, alongside many other phylogenetic groups (Slater et al., 2017; Steinthorsdottir et al., 2020), as the world descended into a bipolar icehouse state.
Despite the reduction in morphospecies diversity from ∼ 3 Ma, the late Cenozoic closure of the Tethyan and Central American Seaways (Crame and Rosen, 2002; Brierly and Fedorov, 2010; Hamon et al., 2013; Matthews et al., 2016) may have contributed to the notable rise in diversity through the Neogene (Aze et al., 2011; Ezard et al., 2011; Peters et al., 2013; Fraass et al., 2015; Lowery et al., 2020), due to a significantly more heterogenous ocean structure via longitudinal obstruction of tropical-subtropical waters by continental reconfiguration, and latitudinal partitioning caused by the steepening of global meridional temperature gradients (Haug et al., 2001; Schmidt et al., 2004a, b; Knappertsbusch, 2016; Ford et al., 2022; Friesenhagen, 2022; Gaskell et al., 2022). Moreover, this intensified, heterogenous icehouse climate may have played a significant role in shaping the incredible diversity observed within modern planktonic foraminiferal cryptic genotypes (Darling and Wade, 2008; Aurahs et al., 2009; Morard et al., 2009, 2013, 2019; Ujiié et al., 2010; Norris and Hull, 2012; Weiner et al., 2012, 2014; André et al., 2014; Ujiié and Ishitani, 2016), though further work is required on the quantification of planktonic foraminiferal cryptic diversity within deep time (André et al., 2013).
It should be noted however, that there is a prominent increase in sampling of Quaternary high latitudes that is yet to be replicated in deep-time marine records (Lazarus, 1994; Diepenbroek et al., 2002; Sellén et al., 2010; O'Regan, 2011; Siccha and Kučera 2017; Waelbroeck et al., 2019; Renaudie et al., 2020; Fenton and Woodhouse et al., 2021). This is due to the difficulties encountered in deep-sea scientific drilling of higher latitudes through the combined impacts of unpredictable and detrimental oceanographic conditions, and ephemeral sea ice (Barker et al., 1977; Backman et al., 2006; Lamy et al., 2019; McKay et al., 2019). Consequently, the substantial increase in cold-water forms observed from ∼ 3 Ma to the Recent (Fig. 5) may be at least partly driven by sampling efforts.
The high-resolution planktonic foraminiferal biotic record at IODP Site U1338 chronicles important changes in late Cenozoic development of global climate and the evolutionary history of this microfossil group. The co-extinction of three species of Dentoglobigerina and the acme of M. cf. exilis and M. cf. pertenuis signify useful regional biostratigraphic markers within the Pacific chronological framework, where the former appears to be associated with abiotic changes in water column structure associated with the closure of the Central American Seaway, and intensification of bipolar cryosphere development. These three extinct dentoglobigerinids appear to exhibit a symbiotic, mixed layer ecological niche habit; however, the compilation of results from previous studies may suggest a high degree of unknown cryptic diversity within dentoglobigerinid morphospecies complexes. The abiotic extinction mechanism is likely signified by the initiation of a critical stage in the formation of Northern Hemisphere ice sheets and the accompanying changes to global paleoceanography and water column structure, where cold and deep dwelling species started to become more dominant across the globe.
Globally, planktonic foraminiferal ecological and macroevolutionary patterns were fundamentally different prior to bipolar ice sheet expansion, and past intervals with climatic conditions analogous to future-warming scenarios such as the mid-Pliocene Warm Period require further prospection at multiple spatiotemporal scales to better predict potential changes in global marine biodiversity as the Earth continues to shift away from its pre-industrial state. Furthermore, anthropogenic forcing has the capability to melt modern continental-scale ice sheets, which hold the potential to dramatically disrupt global ocean circulation patterns, nutrient distributions, and water column structure. Based on past changes in such systems, this may signify impending repercussions for planktonic foraminiferal biodiversity and other planktonic groups whose survival depends on the vertical structure of the oceanic water column.
All data included in the Supplement.
All samples available upon request.
The supplement related to this article is available online at: https://doi.org/10.5194/bg-20-121-2023-supplement.
AW, FAP, SLJ, RAJ, and RJN generated the data. All authors contributed to the interpretation of the data. AW plotted the figures and wrote the R code to perform the statistical analysis. FAP collected the SEM images. AW and TA contributed to the writing and editing of the manuscript.
The contact author has declared that none of the authors has any competing interests.
Publisher's note: Copernicus Publications remains neutral with regard to jurisdictional claims in published maps and institutional affiliations.
This research used samples provided by the International Ocean Discovery Program (IODP). This work was supported by the Natural Environmental Research Council (Studentship grants NE/L002574/1 and NE/S007458/1), the Yorkshire Geological Society Fearnsides Award, and the University of Texas Institute for Geophysics. We would also like to extend our thanks to Chris Lowery for comments, the Associate Editor, and the two anonymous reviewers for their comments and feedback, which greatly helped us to improve the manuscript.
This research has been supported by the Natural Environment Research Council (grant nos. NE/L002574/1 and NE/S007458/1).
This paper was edited by Petr Kuneš and reviewed by two anonymous referees.
André, A., Weiner, A., Quillévéré, F., Aurahs, R., Morard, R., Douady, C. J., de Garidel-Thoron, T., Escarguel, G., de Vargas, C., and Kucera, M.: The cryptic and the apparent reversed: lack of genetic differentiation within the morphologically diverse plexus of the planktonic foraminifer Globigerinoides sacculifer, Paleobiology, 39, 21–39, 2013.
André, A., Quillévéré, F., Morard, R., Ujiié, Y., Escarguel, G., De Vargas, C., de Garidel-Thoron, T., and Douady, C. J.: SSU rDNA divergence in planktonic foraminifera: molecular taxonomy and biogeographic implications, PLoS One, 9, e104641, https://doi.org/10.1371/journal.pone.0104641, 2014.
Aurahs, R., Grimm, G. W., Hemleben, V., Hemleben, C., and Kucera, M.: Geographical distribution of cryptic genetic types in the planktonic foraminifer Globigerinoides ruber, Mol. Ecol., 18, 1692–1706, 2009.
Aze, T., Ezard, T. H., Purvis, A., Coxall, H. K., Stewart, D. R., Wade, B. S., and Pearson, P. N.: A phylogeny of Cenozoic macroperforate planktonic foraminifera from fossil data, Biol. Rev., 86, 900–927, 2011.
Backman, J., Moran, K., McInroy, D. B., Mayer, L. A., and the Expedition 302 Scientists: Arctic Coring Expedition (ACEX), Proc. Integr. Ocean Drill. Program, 302, Edinburgh, Integrated Ocean Drilling Program Management International, Inc., https://doi.org/10.2204/iodp.proc.302.2006, 2006.
Barker, P. F., Dalziel, I. W. D., Dinkelman, M. G., Elliot, D. H., Gombos, A. M., Lonardi, A., Plafker, G., Tarney, J., Thompson, R. W., Tjalsma, R. C., and Von der Borch, C. C.: Evolution of the southwestern Atlantic Ocean Basin: results of Leg 36, Deep Sea Drilling Project, Initial Reports of the Deep Sea Drilling Project, 36, 993–1014, 1977.
Bartoli, G., Sarnthein, M., Weinelt, M., Erlenkeuser, H., Garbe-Schönberg, D., and Lea, D. W.: Final closure of Panama and the onset of northern hemisphere glaciation, Earth Planet. Sc. Lett., 237, 33–44, 2005.
Bartoli, G., Hönisch, B., and Zeebe, R. E.: Atmospheric CO2 decline during the Pliocene intensification of Northern Hemisphere glaciations, Paleoceanography, 26, 4213, https://doi.org/10.1029/2010PA002055, 2011.
Barton, A. D., Irwin, A. J., Finkel, Z. V., and Stock, C. A.: Anthropogenic climate change drives shift and shuffle in North Atlantic phytoplankton communities, P. Natl. Acad. Sci. USA, 113, 2964–2969, 2016.
Beaugrand, G., Reid, P. C., Ibanez, F., Lindley, J. A., and Edwards, M.: Reorganization of North Atlantic marine copepod biodiversity and climate, Science, 296, 1692–1694, 2002.
Berger, W. H., Killingley, J. S., and Vincent, E.: Stable isotopes in deep-sea carbonates-box core erdc-92, west equatorial pacific, Oceanol. Acta, 1, 203–216, 1978.
Bijma, J., Hemleben, C., Huber, B. T., Erlenkeuser, H., and Kroon, D.: 1998. Experimental determination of the ontogenetic stable isotope variability in two morphotypes of Globigerinella siphonifera (d'Orbigny), Mar. Micropaleontol., 35, 141–160, 1998.
Bijma, J., Spero, H. J., and Lea, D. W.: Reassessing foraminiferal stable isotope geochemistry: Impact of the oceanic carbonate system (experimental results), in: Use of proxies in paleoceanography, Springer, Berlin, Heidelberg, 489–512, 1999.
Bindoff, N. L., Cheung, W. W., Kairo, J. G., Arístegui, J., Guinder, A., Hallberg, R., Hilmi, N. J. M., Jiao, N., Karim, M. S., Levin, L., and O'Donoghue, S.: Changing ocean, marine ecosystems, and dependent communities, IPCC special report on the ocean and cryosphere in a changing climate, 477–587, 2019.
Birch, H., Coxall, H. K., Pearson, P. N., Kroon, D., and O'Regan, M.: Planktonic foraminifera stable isotopes and water column structure: Disentangling ecological signals, Mar. Micropaleontol., 101, 127–145, https://doi.org/10.1016/j.marmicro.2013.02.002, 2013.
Boersma, A. and Silva, I. P.: Distribution of Paleogene planktonic foraminifera – analogies with the Recent?, Palaeogeogr. Palaeocl., 83, 29–47, 1991.
Boscolo-Galazzo, F., Crichton, K. A., Ridgwell, A., Mawbey, E. M., Wade, B. S., and Pearson, P. N.: Temperature controls carbon cycling and biological evolution in the ocean twilight zone, Science, 371, 1148–1152, 2021.
Boscolo-Galazzo, F., Jones, A., Dunkley Jones, T., Crichton, K. A., Wade, B. S., and Pearson, P. N.: Late Neogene evolution of modern deep-dwelling plankton, Biogeosciences, 19, 743–762, https://doi.org/10.5194/bg-19-743-2022, 2022.
Bossio, A., Rakich El-Bied, K., Gianelli, L., Mazzei, R., Russo, A., and Salvatorini, G.: Corrélation de quelques sections stratigraphiques du Mio-Pliocene de la zone Atlantique du Maroc avec les stratotypes du Bassin Méditerranéen sur la base de foraminifères planctoniques, nannoplancton calcaire et Ostracodes, Atti della Societa Toscana Scienze Naturali Memorie Pisa, 83, 121–137, 1976.
Bouvier-Soumagnac, Y. and Duplessy, J. C.: Carbon and oxygen isotopic composition of planktonic foraminifera from laboratory culture, plankton tows and recent sediment; implications for the reconstruction of paleoclimatic conditions and of the global carbon cycle, J. Foramin. Res., 15 , 302–320, 1985.
Brierley, C. M. and Fedorov, A. V.: Relative importance of meridional and zonal sea surface temperature gradients for the onset of the ice ages and Pliocene-Pleistocene climate evolution, Paleoceanography, 25, PA2214, https://doi.org/10.1029/2009PA001809, 2010.
Brombacher, A., Wilson, P. A., Bailey, I., and Ezard, T. H.: The breakdown of static and evolutionary allometries during climatic upheaval, Am. Natural., 190, 350–362, 2017a.
Brombacher, A., Wilson, P. A., and Ezard, T. H.: Calibration of the repeatability of foraminiferal test size and shape measures with recommendations for future use, Mar. Micropaleontol., 133, 21–27, 2017b.
Brombacher, A., Elder, L. E., Hull, P. M., Wilson, P. A., and Ezard, T. H.: Calibration of test diameter and area as proxies for body size in the planktonic foraminifer Globoconella puncticulata, J. Foramin. Res., 48, 241–245, 2018.
Brombacher, A., Wilson, P. A., Bailey, I., and Ezard, T. H.: The dynamics of diachronous extinction associated with climatic deterioration near the Neogene/Quaternary boundary, Paleoceanogr. Paleocl., 36, e2020PA004205, https://doi.org/10.1029/2020PA004205, 2021.
Brönniman, P. and Resig, J.: A Neogene Globigerinacean biochronologic time-scale for the southwestern Pacific: DSDP Leg 7, in: edited by: Winterer, E. L., Riedel, W. R., Broennimann, P., Gealy, E. L., Heath, G. R., Kroenke, L. W., Martini, E., Moberly, R., Jr., Resig, J. M., and Worsley, T. R., Initial reports of the Deep Sea Drilling Project, Vol. 7, Part 2: Washington, D.C., U.S. Government Printing Office, 1235–1470, 1971
Cannariato, K. G. and Ravelo, A. C.: Pliocene-Pleistocene evolution of eastern tropical Pacific surface water circulation and thermocline depth, Paleoceanography, 12, 805–820, 1997.
Ceballos, G., Ehrlich, P. R., Barnosky, A. D., García, A., Pringle, R. M., and Palmer, T. M.: Accelerated modern human-induced species losses: Entering the sixth mass extinction, Sci. Adv., 1, e1400253, https://doi.org/10.1126/sciadv.1400253, 2015.
Chaisson, W.: Planktonic foraminiferal assemblages and palaeoceanographic change in the transtropical Pacific Ocean: a comparison of west (Leg 130) and east (Leg 138), latest Miocene to Pleistocene, Proc. ODP Sci. Res., 138, 555–597, 1995.
Chaisson, W. P.: Vicarious living: Pliocene menardellids between an isthmus and an ice sheet, Geology, 31, 1085–1088, 2003.
Chaisson, W. P. and Leckie, R. M.: High resolution Neogene planktonic foraminifer biostratigraphy of Site 806, Ontong Java Plateau (western equatorial Pacific), in: Proceedings of the Ocean Drilling Program, edited by: Janecek, T., Backman, J., Bassinot, F., and Borfield, R. M., Scientific results, Vol. 130, College Station, Texas, Ocean Drilling Program, 137–178, 1993.
Chaisson, W. P. and Pearson, P. N.: Planktonic foraminifer biostratigraphy at Site 925: Middle Miocene–Pleistocene, edited by: Shackleton, N. J., Curry, W. B., Richter, C., and Bralower, T. J., Proceeding of the Ocean Drilling Program, Scientific Results, 154, 3–31, 1997.
Chaisson, W. P. and Ravelo, A. C.: Pliocene development of the east-west hydrographic gradient in the equatorial Pacific, Paleoceanography, 15, 497–505, 2000.
Chandler, M., Rind, D., and Thompson, R.: Joint investigations of the middle Pliocene climate II: GISS GCM Northern Hemisphere results, Glob. Planet. Change, 9, 197–219, 1994.
Cheung, W. W., Watson, R., and Pauly, D.: Signature of ocean warming in global fisheries catch, Nature, 497, 365–368, 2013.
Coates, A. G., and Obando, J. A.: The geologic evolution of the Central American isthmus, in: Evolution and environment in tropical America, edited by: Jackson, J. B. C., Budd, A. F., and Coates, A. G., The University of Chicago Press, Chicago, Illinois, 21–56, 1996.
Corfield, R. M. and Cartlidge, J. E.: Isotopic evidence for the depth stratification of fossil and recent Globigerinina: a review, Hist. Biol., 5, 37–63, 1991.
Crame, J. A., and Rosen, B. R.: Cenozoic palaeogeography and the rise of modern biodiversity patterns, Geol. Soc. Lond. Spec. Publ., 194, 153–168, 2002.
Cramer, B. S., Toggweiler, J. R., Wright, J. D., Katz, M. E., and Miller, K. G.: Ocean overturning since the Late Cretaceous: Inferences from a new benthic foraminiferal isotope compilation, Paleoceanography, 24, PA4216, https://doi.org/10.1029/2008PA001683, 2009.
Cramer, B. S., Miller, K. G., Barrett, P. J., and Wright, J. D.: Late Cretaceous–Neogene trends in deep ocean temperature and continental ice volume: Reconciling records of benthic foraminiferal geochemistry (δ18O and Mg Ca) with sea level history, J.Geophys. Res.-Ocean., 116, C12023, https://doi.org/10.1029/2011JC007255, 2011.
Crundwell, M. P.: Pliocene and early Pleistocene planktic foraminifera: Important taxa and bioevents in ODP Hole 1123B, Chatham Rise, New Zealand, GNS Science Report 2015/51, August 2015, 67, 2015a.
Crundwell, M. P.: Revised Pliocene and early Pleistocene planktic foraminiferal biostratigraphy, DSDP Site 284, Challenger Plateau, New Zealand, GNS Science Internal Report 2015/22, December 2015, 36, 2015b.
Crundwell, M. P.: Globoconella pseudospinosa, N. Sp.: a New Early Pliocene Planktonic Foraminifera from the Southwest Pacific, J. Foramin. Res., 48, 288–300, 2018.
Crundwell, M. P. and Nelson, C. S.: A magnetostratigraphically-constrained chronology for late Miocene bolboformids and planktic foraminifers in the temperate Southwest Pacific, Stratigraphy, 4, 1–34, 2007.
Crundwell, M. P. and Woodhouse, A.: A detailed biostratigraphic framework for 0–1.2 Ma Quaternary sediments of north-eastern Zealandia, New Zealand J. Geol. Geophys., 1–14, https://doi.org/10.1080/00288306.2022.2054828, 2022a.
Crundwell, M. P. and Woodhouse, A.: Biostratigraphically constrained chronologies for Quaternary sequences from the Hikurangi margin of north-eastern Zealandia, New Zeal. J. Geol. Geop., 1–21, https://doi.org/10.1080/00288306.2022.2101481, 2022b.
Darling, K. F. and Wade, C. M.: The genetic diversity of planktic foraminifera and the global distribution of ribosomal RNA genotypes, Mar. Micropaleontol., 67, 216–238, 2008.
de Vargas, C., Norris, R., Zaninetti, L., Gibb, S. W., and Pawlowski, J.: Molecular evidence of cryptic speciation in planktonic foraminifers and their relation to oceanic provinces, P. Natl. Acad. Sci. USA, 96, 2864–2868, 1999.
de Vargas, C., Bonzon, M., Rees, N. W., Pawlowski, J., and Zaninetti, L.: A molecular approach to biodiversity and biogeography in the planktonic foraminifer Globigerinella siphonifera (d'Orbigny), Mar. Micropaleontol., 45, 101–116, 2002.
Diepenbroek, M., Grobe, H., Reinke, M., Schindler, U., Schlitzer, R., Sieger, R., and Wefer, G.: PANGAEA – an information system for environmental sciences, Comput. Geosci., 28, 1201–1210, 2002.
Dowsett, H. J., Robinson, M. M., Haywood, A. M., Hill, D. J., Dolan, A. M., Stoll, D. K., Chan, W. L., Abe-Ouchi, A., Chandler, M. A., Rosenbloom, N. A., and Otto-Bliesner, B. L.: Assessing confidence in Pliocene sea surface temperatures to evaluate predictive models, Nat. Clim. Change, 2, 365–371, 2012.
Drury, A. J., Lee, G. P., Pennock, G. M., and John, C. M.: Data report: Late Miocene to early Pliocene coccolithophore and foraminiferal preservation at Site U1338 from scanning electron microscopy, in: Proceedings of the Integrated Ocean Drilling Program, 320, Integrated Ocean Drilling Program, 1–14, 2014.
Edgar, K. M., Bohaty, S. M., Gibbs, S. J., Sexton, P. F., Norris, R. D., and Wilson, P. A.: Symbiont “bleaching” in planktic foraminifera during the Middle Eocene Climatic Optimum, Geology, 41, 15–18, 2013a.
Edgar, K. M., Pälike, H., and Wilson, P. A.: Testing the impact of diagenesis on the δ18O and δ13C of benthic foraminiferal calcite from a sediment burial depth transect in the equatorial Pacific, Paleoceanography, 28, 468–480, 2013b.
Edgar, K. M., Hull, P. M., and Ezard, T. H.: Evolutionary history biases inferences of ecology and environment from δ13C but not δ18O values, Nat. Commun., 8, 1–9, 2017.
Edwards, M., Beaugrand, G., Kléparski, L., Hélaouët, P., and Reid, P. C.: Climate variability and multi-decadal diatom abundance in the Northeast Atlantic, Commun. Earth Environ., 3, 1–8, 2022.
Ericson, D. B., Wollin, G., and Wollin, J.: Coiling direction of Globorotalia truncatulinoides in deep-sea cores, Deep-Sea Res., 2, 152–158, 1955.
Ezard, T. H., Aze, T., Pearson, P. N., and Purvis, A.: Interplay between changing climate and species' ecology drives macroevolutionary dynamics, Science, 332, 349–351, 2011.
Fairbanks, R. G., Charles, C. D., and Wright, J. D., Origin of global meltwater pulses, in: Radiocarbon after four decades, Springer, New York, NY, 473–500, 1992.
Falzoni, F., Petrizzo, M. R., and Valagussa, M.: A morphometric methodology to assess planktonic foraminiferal response to environmental perturbations: the case study of Oceanic Anoxic Event 2, Late Cretaceous, 2018.
Fedorov, A. V., Pacanowski, R. C., Philander, S. G., and Boccaletti, G.: The effect of salinity on the wind-driven circulation and the thermal structure of the upper ocean, J. Phys. Oceanogr., 34, 1949–1966, 2004.
Fedorov, A., Dekens, P. S., McCarthy, M., Ravelo, A. C., DeMenocal, P. B., Barreiro, M., Pacanowski, R. C., and Philander, S. G.: The Pliocene paradox (mechanisms for a permanent El Niño), Science, 312, 1485–1489, 2006.
Fedorov, A. V., Brierley, C. M., Lawrence, K. T., Liu, Z., Dekens, P. S., and Ravelo, A. C.: Patterns and mechanisms of early Pliocene warmth, Nature, 496, 43–49, 2013.
Fenton, I. S., Woodhouse, A., Aze, T., Lazarus, D., Renaudie, J., Dunhill, A. M., Young, J. R., and Saupe, E. E.: Triton, a new species-level database of Cenozoic planktonic foraminiferal occurrences, Sci. Data, 8, 1–9, 2021.
Fiedler, P. C.: Seasonal climatologies and variability of the eastern tropical Pacific surface waters Technical Reports of the U.S. National Marine Fishery Service, 109, 1–65, 1992.
Fiedler, P. C. and Talley, L. D.: Hydrography of the eastern tropical Pacific: A review, Prog. Oceanogr., 69, 143–180, 2006.
Flannery-Sutherland, J. T., Raja, N. B., Kocsis, Á, T., and Kiessling, W.: fossilbrush: An R package for automated detection and resolution of anomalies in palaeontological occurrence data, Method. Ecol. Evol., 13, 2404–2418, 2022.
Ford, H. L., Ravelo, A. C., and Hovan, S.: A deep Eastern Equatorial Pacific thermocline during the early Pliocene warm period, Earth Planet. Sc. Lett., 355, 152–161, 2012.
Ford, H. L., Ravelo, A. C., Dekens, P. S., LaRiviere, J. P., and Wara, M. W.: The evolution of the equatorial thermocline and the early Pliocene El Padre mean state, Geophys. Res. Lett., 42, 4878–4887, 2015.
Ford, H. L., Burls, N. J., Jacobs, P., Jahn, A., Caballero-Gill, R. P., Hodell, D. A., and Fedorov, A. V.: Sustained mid-Pliocene warmth led to deep water formation in the North Pacific, Nat. Geosci., 15, 658–663, 2022.
Fox, L., Stukins, S., Hill, T., and Miller, C. G.: Quantifying the effect of anthropogenic climate change on calcifying plankton, Sci. Rep., 10, 1–9, 2020.
Fox, L. R. and Wade, B. S.: Systematic taxonomy of early–middle Miocene planktonic foraminifera from the equatorial Pacific Ocean: Integrated Ocean Drilling Program, Site U1338, J. Foramin. Res., 43, 374–405, 2013.
Fraass, A. J., Kelly, D. C., and Peters, S. E.: Macroevolutionary history of the planktic foraminifera, Ann. Rev. Earth Planet. Sc., 43, 139–166, 2015.
Friesenhagen, T.: Test-size evolution of the planktonic foraminifer Globorotalia menardii in the eastern tropical Atlantic since the Late Miocene, Biogeosciences, 19, 777–805, https://doi.org/10.5194/bg-19-777-2022, 2022.
Frigola, A., Prange, M., and Schulz, M.: Boundary conditions for the Middle Miocene Climate Transition (MMCT v1.0), Geosci. Model Dev., 11, 1607–1626, https://doi.org/10.5194/gmd-11-1607-2018, 2018.
Garcia, H. E., Locarnini, R. A., Boyer, T. P., and Antonov, J. I.: World Ocean Atlas 2005, U.S. Government Printing Office, Washington, 2006.
Gaskell, D. E., Huber, M., O'Brien, C. L., Inglis, G. N., Acosta, R. P., Poulsen, C. J., and Hull, P. M.: The latitudinal temperature gradient and its climate dependence as inferred from foraminiferal δ18O over the past 95 million years, P. Natl. Acad. Sci. USA, 119, e2111332119, https://doi.org/10.1073/pnas.2111332119, 2022.
Golledge, N. R., Keller, E. D., Gomez, N., Naughten, K. A., Bernales, J., Trusel, L. D., and Edwards, T. L.: Global environmental consequences of twenty-first-century ice-sheet melt, Nature, 566, 65–72, 2019.
Groeneveld, J., Steph, S., Tiedemann, R., Garbe-Schönberg, C., Nürnberg, D., and Sturm, A.: Pliocene mixed-layer oceanography for Site 1241, using combined Mg Ca and δ18O analyses of Globigerinoides sacculifer, in: Proceedings of the Ocean Drilling Program: Scientific Results, 202, Texas AandM University, 1–27, 2006.
Groeneveld, J., De Vleeschouwer, D., McCaffrey, J. C., and Gallagher, S. J.: Dating the northwest shelf of Australia since the Pliocene, Geochem. Geophy. Geosy., 22, e2020GC009418, https://doi.org/10.1029/2020GC009418, 2021.
Hallock, P. and Larsen, A. R.: Coiling direction in Amphistegina, Mar. Micropaleontol., 4, 33–44, 1979.
Hamon, N., Sepulchre, P., Lefebvre, V., and Ramstein, G.: The role of eastern Tethys seaway closure in the Middle Miocene Climatic Transition (ca. 14 Ma), Clim. Past, 9, 2687–2702, https://doi.org/10.5194/cp-9-2687-2013, 2013.
Haywood, A. M., Sellwood, B. W., and Valdes, P. J.: Regional warming: Pliocene (3 Ma) paleoclimate of Europe and the Mediterranean, Geology, 28, 1063–1066, 2000.
Haywood, A. M., Dowsett, H. J., and Dolan, A. M.: Integrating geological archives and climate models for the mid-Pliocene warm period, Nat. Commun., 7, 1–14, 2016.
Haug, G. H. and Tiedemann, R.: Effect of the formation of the Isthmus of Panama on Atlantic Ocean thermohaline circulation, Nature, 393, 673–676, 1998.
Haug, G. H., Tiedemann, R., Zahn, R., and Ravelo, A. C.: Role of Panama uplift on oceanic freshwater balance, Geology, 29, 207–210, 2001.
Hayashi, T., Yamanaka, T., Hikasa, Y., Sato, M., Kuwahara, Y., and Ohno, M. Latest Pliocene Northern Hemisphere glaciation amplified by intensified Atlantic meridional overturning circulation, Commun. Earth Environ., 1, 1–10, 2020.
Herbert, T. D., Peterson, L. C., Lawrence, K. T., and Liu, Z.: Tropical ocean temperatures over the past 3.5 million years, Science, 328, 1530–1534, 2010.
Hodell, D. A. and Vayavananda, A.: Middle Miocene paleoceanography of the western equatorial Pacific (DSDP site 289) and the evolution of Globorotalia (Fohsella), Mar. Micropaleontol., 22, 279–310, 1993.
Holbourn, A., Kuhnt, W., Kochhann, K. G., Andersen, N., and Sebastian Meier, K. J.: Global perturbation of the carbon cycle at the onset of the Miocene Climatic Optimum, Geology, 43, 123–126, 2015.
Hornibrook, N. D.: Late Miocene to Pleistocene Globorotalia (Foraminiferida) from DSDP Leg 29, Site 284, Southwest Pacific, New Zeal. J. Geol. Geophys., 25, 83–99, 1982.
Hu, A., Meehl, G. A., Han, W., and Yin, J.: Effect of the potential melting of the Greenland Ice Sheet on the Meridional Overturning Circulation and global climate in the future, Deep-Sea Res. Pt. II, 58, 1914–1926, 2011.
Huber, B. T., Bijma, J., and Darling, K.: Cryptic speciation in the living planktonic foraminifer Globigerinella siphonifera (d'Orbigny), Paleobiology, 23, 33–62, 1997.
Hull, P. M. and Norris, R. D.: Evidence for abrupt speciation in a classic case of gradual evolution, P. Natl. Acad. Sci. USA, 106, 21224–21229, 2009.
Hupp, B. N., Kelly, D. C., and Williams, J. W.: Isotopic filtering reveals high sensitivity of planktic calcifiers to Paleocene–Eocene thermal maximum warming and acidification, P. Natl. Acad. Sci. USA, 119, e2115561119, https://doi.org/10.1073/pnas.2115561119, 2022.
IPCC: Climate Change 2022: Impacts, Adaptation, and Vulnerability. Contribution of Working Group II to the Sixth Assessment Report of the Intergovernmental Panel on Climate Change, edited by: Pörtner, H.-O., Roberts, D. C., Tignor, M., Poloczanska, E. S., Mintenbeck, K., Alegría, A., Craig, M., Langsdorf, S., Löschke, S., Möller, V., Okem, A., Rama, B., Cambridge University Press. Cambridge University Press, Cambridge, UK and New York, NY, USA, 3056 pp., https://doi.org/10.1017/9781009325844, 2022.
Jenkins, D. G. and Orr, W. N.: Planktonic foraminiferal biostratigraphy of the eastern equatorial Pacific: DSDP 9, in: Initial reports of the Deep Sea Drilling Project, Vol. 9, edited by: Hays, J. D., Washington, D.C., U.S. Government Printing Office, 1057–1196, 1972.
Jiang, D., Wang, H., Ding, Z., Lang, X., and Drange, H.: Modeling the middle Pliocene climate with a global atmospheric general circulation model, J. Geophys. Res.-Atmos., 110, https://doi.org/10.1029/2004JD005639, 2005.
Jonkers, L., Hillebrand, H., and Kucera, M.: Global change drives modern plankton communities away from the pre-industrial state, Nature, 570, 372–375, 2019.
Kaneps, A. G.: Cenozoic planktonic foraminifera from Antarctic deep-sea sediments, Leg 28, DSDP, Initial Reports of the Deep Sea Drilling Project, 28, 573–583, 1975.
Kearns, L. E., Bohaty, S. M., Edgar, K. M., Nogué, S., and Ezard, T. H.: Searching for Function: Reconstructing Adaptive Niche Changes Using Geochemical and Morphological Data in Planktonic Foraminifera, Front. Ecol. Evol., 9, 679722, https://doi.org/10.3389/fevo.2021.679722, 2021.
Kearns, L. E., Bohaty, S. M., Edgar, K. M., and Ezard, T. H. G.: Small but mighty: how overlooked small species maintain community structure through middle Eocene climate change, Paleobiology, https://doi.org/10.1017/pab.2022.24, 1–22, 2022.
Keigwin Jr., L. D.: Neogene planktonic foraminifers from Deep Sea Drilling Project sites 502 and 503, Initial Reports of the Deep Sea Drilling Project, 68, 269–288, 1982.
Keigwin Jr., L. D.: Pliocene closing of the Isthmus of Panama, based on biostratigraphic evidence from nearby Pacific Ocean and Caribbean Sea cores, Geological Society of America (GSA), Boulder, CO, United States, Geology, 6, 630–634, 1978.
Keller, G.: Biochronology and paleoclimatic implications of middle Eocene to Oligocene planktic foraminiferal faunas, Mar. Micropaleontol., 7, 463–486, 1983.
Keller, G.: Depth stratification of planktonic foraminifers in the Miocene ocean, The Miocene ocean: Paleoceanography and Biogeography, 163, 177–196, 1985.
Keller, G. and Savin, S. M.: Evolution of the Miocene ocean in the eastern North Pacific as inferred from oxygen and carbon isotopic ratios of foraminifera, The Miocene Ocean: Paleoceanography and Biogeography, 163, 83, 1985.
Keller, G., Zenker, C. E., and Stone, S. M.: Late Neogene history of the Pacific-Caribbean gateway, J. South Am. Earth Sci., 2, 73–108, 1989.
Keller, G., MacLeod, N., and Barrera, E.: Eocene-Oligocene faunal turnover in planktic foraminifer, and Antarctic glaciation, in: Eocene-Oligocene climatic and biotic evolution, edited by: Prothero, D. R. and Berggren, W. A., Princeton University Press, Princeton, 218–244, 1992.
Kennett, J. P. and Srinivasan, M. S.: Neogene planktonic foraminifera, A phylogenetic atlas, Hutchinson Ross Pub. Co., 265, 1983.
Kim, S. T. and O'Neil, J. R.: Equilibrium and nonequilibrium oxygen isotope effects in synthetic carbonates, Geochim. Cosmochim. Ac., 61, 3461–3475, 1997.
Kleiven, H. F., Jansen, E., Fronval, T., and Smith, T. M.: Intensification of Northern Hemisphere glaciations in the circum Atlantic region (3.5–2.4 Ma)–ice-rafted detritus evidence, Palaeogeogr. Palaeocl., 184, 213–223, 2002.
Knappertsbusch, M.: Evolutionary prospection in the Neogene planktic foraminifer Globorotalia menardii and related forms from ODP Hole 925B (Ceara Rise, western tropical Atlantic): evidence for gradual evolution superimposed by long distance dispersal?, Swiss J. Palaeontol., 135, 205–248, 2016.
Knappertsbusch, M.: Morphological variability of Globorotalia menardii (planktonic foraminifera) in two DSDP cores from the Caribbean Sea and the Eastern Equatorial Pacific, Carnets de géologie, 7, 1–34, https://doi.org/10.4267/2042/8455, 2007.
Kotov, S. and Pälike, H.: QAnalySeries-a cross-platform time series tuning and analysis tool, in: AGU Fall Meeting Abstracts, Vol. 2018, PP53D-1230, 2018.
Kucera, M. and Schönfeld, J.: The origin of modern oceanic foraminiferal faunas and Neogene climate change, Deep-Time Perspectives on Climate Change: Marrying the Signal from Computer Models and Biological Proxies, London, Geol. Soc., 2, 409–426, 2007.
Lam, A. R. and Leckie, R. M.: Late Neogene and Quaternary diversity and taxonomy of subtropical to temperate planktic foraminifera across the Kuroshio Current Extension, northwest Pacific Ocean, Micropaleontology, 66, 177–268, 2020.
Lamy, F., Winckler, G., and Alvarez Zarikian, C. A.: Expedition 383 Preliminary Report: Dynamics of the Pacific Antarctic Circumpolar Current (DYNAPACC), Preliminary Report, 383, 2019.
Lawrence, K. T., Liu, Z., and Herbert, T. D.: Evolution of the eastern tropical Pacific through Plio-Pleistocene glaciation, Science, 312, 79–83, 2006.
Lazarus, D.: Neptune: a marine micropaleontology database, Math. Geol., 26, 817–832, 1994.
Lazarus, D., Weinkauf, M., and Diver, P.: Pacman profiling: a simple procedure to identify stratigraphic outliers in high-density deep-sea microfossil dataPACMAN PROFILING, Paleobiology, 38, 144–161, 2012.
Levin, M., Klar, A. J., and Ramsdell, A. F.: Introduction to provocative questions in left–right asymmetry, Philos. T. R. Soc. B, 371, 20150399, https://doi.org/10.1098/rstb.2015.0399, 2016.
Liow, L. H., Skaug, H. J., Ergon, T., and Schweder, T.: Global occurrence trajectories of microfossils: environmental volatility and the rise and fall of individual species, Paleobiology, 36, 224–252, 2010.
Lohmann, G. P.: A model for variation in the chemistry of planktonic foraminifera due to secondary calcification and selective dissolution, Paleoceanography, 10, 445–457, 1995.
Lowery, C. M. and Fraass, A. J.: Morphospace expansion paces taxonomic diversification after end Cretaceous mass extinction, Nat. Ecol. Evol., 3, 900–904, 2019.
Lowery, C. M., Bown, P. R., Fraass, A. J., and Hull, P. M.: Ecological response of plankton to environmental change: thresholds for extinction, Ann. Rev. Earth Planet. Sc., 48, 403–429, 2020.
Lutz, B. P.: Low-latitude northern hemisphere oceanographic and climatic responses to early shoaling of the Central American Seaway, Stratigraphy, 7, 151–176, 2010.
Lyle, M., Drury, A. J., Tian, J., Wilkens, R., and Westerhold, T.: Late Miocene to Holocene high-resolution eastern equatorial Pacific carbonate records: stratigraphy linked by dissolution and paleoproductivity, Clim. Past, 15, 1715–1739, https://doi.org/10.5194/cp-15-1715-2019, 2019.
Malmgren, B. A. and Berggren, W. A.: Evolutionary changes in some Late Neogene planktonic foraminiferal lineages and their relationships to paleoceanographic changes, Paleoceanography, 2, 445–456, 1987.
Maslin, M. A., Haug, G. H., Sarnthein, M., and Tiedemann, R.: The progressive intensification of northern hemisphere glaciation as seen from the North Pacific, Geol. Rundsch., 85, 452–465, 1996.
Matthews, K. J., Maloney, K. T., Zahirovic, S., Williams, S. E., Seton, M., and Mueller, R. D.: Global plate boundary evolution and kinematics since the late Paleozoic, Glob. Planet. Change, 146, 226–250, 2016.
McKay, R. M., De Santis, L., Kulhanek, D. K., Ash, J. L., Beny, F., Browne, I. M., Cortese, G., Cordeiro de Sousa, I. M., Dodd, J. P., Esper, O. M., and Gales, J. A.: Expedition 374 summary, Proceedings of the International Ocean Discovery Program, 2019.
Medina-Elizalde, M., Lea, D. W., and Fantle, M. S.: Implications of seawater Mg Ca variability for Plio-Pleistocene tropical climate reconstruction, Earth Planet. Sc. Lett., 269, 585–595, 2008.
Methner, K., Campani, M., Fiebig, J., Löffler, N., Kempf, O., and Mulch, A.: Middle Miocene long-term continental temperature change in and out of pace with marine climate records, Sci. Rep., 10, 1–10, 2020.
Molina, E., Gonzalvo, C., and Keller, G.: The Eocene-Oligocene planktic foraminiferal transition: extinctions, impacts and hiatuses, Geol. Mag., 130, 483–499, 1993.
Molnar, P.: Closing of the Central American Seaway and the Ice Age: A critical review, Paleoceanography, 23, https://doi.org/10.1029/2007PA001574, 2008.
Morard, R., Quillevere, F., Escarguel, G., Ujiie, Y., de Garidel-Thoron, T., Norris, R. D., and de Vargas, C.: Morphological recognition of cryptic species in the planktonic foraminifer Orbulina universa, Mar. Micropaleontol., 71, 148–165, 2009.
Morard, R., Quillevere, F., Escarguel, G., de Garidel-Thoron, T., de Vargas, C., and Kucera, M.: Ecological modeling of the temperature dependence of cryptic species of planktonic Foraminifera in the Southern Hemisphere, Palaeogeogr. Palaeocl., 391, 13–33, 2013.
Morard, R., Füllberg, A., Brummer, G. J. A., Greco, M., Jonkers, L., Wizemann, A., Weiner, A. K., Darling, K., Siccha, M., Ledevin, R., and Kitazato, H.: Genetic and morphological divergence in the warm-water planktonic foraminifera genus Globigerinoides, PloS one, 14, e0225246, https://doi.org/10.1371/journal.pone.0225246, 2019.
Mudelsee, M. and Raymo, M. E.: Slow dynamics of the Northern Hemisphere glaciation, Paleoceanography, 20, https://doi.org/10.1029/2005PA001153, 2005.
Nirmal, B., Mohan, K., Prakasam, M., Tripati, A., Mortyn, P. G., and Rodríguez-Sanz, L.: Pleistocene surface-ocean changes across the Southern subtropical front recorded by cryptic species of Orbulina universa, Mar. Micropaleontol., 168, 102056, https://doi.org/10.1016/j.marmicro.2021.102056 2021.
Norris, R. D.: Symbiosis as an evolutionary innovation in the radiation of Paleocene planktic foraminifera, Paleobiology, 22, 461–480, 1996.
Norris, R. D.: Hydrographic and tectonic control of plankton distribution and evolution, in: Reconstructing Ocean History, Springer, Boston, MA, 173–193, 1999.
Norris, R. D.: Pelagic species diversity, biogeography, and evolution, Paleobiology, 26, 236–258, 2000.
Norris, R. D. and Hull, P. M.: The temporal dimension of marine speciation, Evol. Ecol., 26, 393–415, 2012.
Norris, R. D. and Nishi, H.: Evolutionary trends in coiling of tropical Paleogene planktic foraminifera, Paleobiology, 27, 327–347, 2001.
Norris, R. D., Corfield, R. M., and Cartlidge, J. E.: Evolution of depth ecology in the planktic foraminifera lineage Globorotalia (Fohsella), Geology, 21, 975–978, 1993.
Norris, R. D., Corfield, R. M., and Cartlidge, J. E.: Evolutionary ecology of Globorotalia (Globoconella) (planktic foraminifera), Mar. Micropaleontol., 23, 121–145, 1994.
Norris, R. D., Corfield, R. M., and Cartlidge, J.: What is gradualism? Cryptic speciation in globorotaliid foraminifera, Paleobiology, 22, 386–405, 1996.
Norris, R. D., Turner, S. K., Hull, P. M., and Ridgwell, A.: Marine ecosystem responses to Cenozoic global change, Science, 341, 492–498, 2013.
O'Dea, A., Lessios, H. A., Coates, A. G., Eytan, R. I., Restrepo-Moreno, S. A., Cione, A. L., Collins, L. S., De Queiroz, A., Farris, D. W., Norris, R. D., and Stallard, R. F.: Formation of the Isthmus of Panama, Sci. Adv., 2, e1600883, https://doi.org/10.1126/sciadv.1600883, 2016.
Öğretmen, N., Schiebel, R., Jochum, K. P., Stoll, B., Weis, U., Repschläger, J., Jentzen, A., Galer, S., and Haug, G. H.: Deep thermohaline circulation across the closure of the Central American Seaway, Paleoceanogr. Paleocl., 35, e2020PA004049, https://doi.org/10.1029/2020PA004049, 2020.
Olsson, R. K.: Cenozoic planktonic Foraminifera: a paleobiogeographic summary, Series in Geology, Notes for Short Course, Foraminifera, 6, 127–147, 1982.
Opdyke, B. N. and Pearson, P. N.: Data report: Geochemical analysis of multiple planktonic foraminifer species at discrete time intervals, in: Proceedings of the Ocean Drilling Program, Scientific Results, 144, 993–995, 1995.
Pagani, M., Liu, Z., LaRiviere, J., and Ravelo, A. C.: High Earth-system climate sensitivity determined from Pliocene carbon dioxide concentrations, Nat. Geosci., 3, 27–30, 2010.
Pälike, H., Lyle, M., Nishi, H., Raffi, I., Gamage, K., Klaus, A., and the Expedition 320/321 Scientists: Site 1338: Proceedings of the Integrated Ocean Drilling Program, Vol. 320/321, https://doi.org/10.2204/iodp.proc.320321.101.2010, 2010.
Pearson, P. N. and Ezard, T. H.: Evolution and speciation in the Eocene planktonic foraminifer Turborotalia, Paleobiology, 40, 130–143, 2014.
Pearson, P. N. and Penny, L.: Coiling directions in the planktonic foraminifer Pulleniatina: A complex eco-evolutionary dynamic spanning millions of years, PloS one, 16, e024911, https://doi.org/10.1371/journal.pone.0249113, 2021.
Pearson, P. N. and Shackleton, N. J.: Neogene multispecies planktonic foraminifer stable isotope record, Site 871, Limalok Guyot, in: Proceedings of the Ocean Drilling Program, Scientific Results, 144, 401–410, 1995.
Pérez-Angel, L. C. and Molnar, P.: Sea surface temperatures in the Eastern Equatorial Pacific and surface temperatures in the Eastern Cordillera of Colombia during El Niño: Implications for Pliocene conditions, Paleoceanography, 32, 1309–1314, 2017.
Peters, S. E., Kelly, D. C., and Fraass, A. J.: Oceanographic controls on the diversity and extinction of planktonic foraminifera, Nature, 493, 398–401, 2013.
Philander, S. G. and Fedorov, A. V.: Role of tropics in changing the response to Milankovich forcing some three million years ago, Paleoceanography, 18, 1045, https://doi.org/10.1029/2002PA000837, 2003.
Pinsky, M. L., Reygondeau, G., Caddell, R., Palacios-Abrantes, J., Spijkers, J., and Cheung, W. W.: Preparing ocean governance for species on the move, Science, 360, 1189–1191, 2018.
Prentice, M. L. and Matthews, R. K.: Cenozoic ice-volume history: development of a composite oxygen isotope record, Geology, 16, 963–966, 1988.
Purich, A., England, M. H., Cai, W., Sullivan, A., and Durack, P. J.: Impacts of broad-scale surface freshening of the Southern Ocean in a coupled climate model, J. Clim., 31, 2613–2632, 2018.
Raffi, I., Wade, B. S., Pälike, H., Beu, A. G., Cooper, R., Crundwell, M. P., Krijgsman, W., Moore, T., Raine, I., Sardella, R., and Vernyhorova, Y. V.: The Neogene period, in: Geologic time scale 2020, Elsevier, 1141–1215, 2020.
Rasmussen, T. L. and Thomsen, E.: Holocene temperature and salinity variability of the Atlantic Water inflow to the Nordic seas, The Holocene, 20, 1223–1234, 2010.
Ravelo, A. C. and Fairbanks, R. G.: Oxygen isotopic composition of multiple species of planktonic foraminifera: Recorders of the modern photic zone temperature gradient, Paleoceanography, 7, 815–831, 1992.
Ravelo, A. C. and Fairbanks, R. G.: Carbon isotopic fractionation in multiple species of planktonic foraminifera from core-tops in the tropical Atlantic, Oceanographic Literature Review, 10, 854, 1995.
Ravelo, A. C. and Shackleton, N. J.: Evidence for surface-water circulation changes at site 851 in the eastern tropical Pacific Ocean, Proceedings of the Ocean Drilling Program Scientific Results, 138, 503–516, 1995.
Ravelo, A. C. and Hillaire-Marcel, C.: Chapter Eighteen: The use of oxygen and carbon isotopes of foraminifera in Paleoceanography, Dev. Mar. Geol., 1, 735–764, 2007.
Rebotim, A., Voelker, A. H. L., Jonkers, L., Waniek, J. J., Meggers, H., Schiebel, R., Fraile, I., Schulz, M., and Kucera, M.: Factors controlling the depth habitat of planktonic foraminifera in the subtropical eastern North Atlantic, Biogeosciences, 14, 827–859, https://doi.org/10.5194/bg-14-827-2017, 2017.
Renaudie, J., Lazarus, D., and Diver, P.: NSB (Neptune Sandbox Berlin): an expanded and improved database of marine planktonic microfossil data and deep-sea stratigraphy, Palaeontol. Electron., 23, a11, https://doi.org/10.26879/1032, 2020.
Rhein, M., Rintoul, S. R., Aoki, S., Campos, E., Chambers, D., Feely, R. A., Gulev, S., Johnson, G. C., Josey, S. A., Kostianoy, A., and Mauritzen, C.: Observations: ocean, in: Climate Change 2013: the Physical Science Basis. Contribution of Working Group I to the Fifth Assessment Report of the Intergovernmental Panel on Climate Change, Cambridge University Press, Cambridge, United Kingdom and New York, NY, USA, 2013.
Rincón-Martínez, D., Steph, S., Lamy, F., Mix, A., and Tiedemann, R.: Tracking the equatorial front in the eastern equatorial Pacific Ocean by the isotopic and faunal composition of planktonic foraminifera, Mar. Micropaleontol., 79, 24–40, 2011.
Rögl, F.: Mediterranean and Paratethys. Facts and hypotheses of an Oligocene to Miocene paleogeography (short overview), Geol. Carpath., 50, 339–349, 1999.
Rosenthal, Y., Holbourn, A. E., Kulhanek, D. K., Aiello, I. W., Babila, T. L., Bayon, G., Beaufort, L., Bova, S. C., Chun, J.-H., Dang, H., Drury, A. J., Dunkley Jones, T., Eichler, P. P. B., Fernando, A. G. S., Gibson, K. A., Hatfield, R. G., Johnson, D. L., Kumagai, Y., Li, T., Linsley, B. K., Meinicke, N., Mountain, G. S., Opdyke, B. N., Pearson, P. N., Poole, C. R., Ravelo, A. C., Sagawa, T., Schmitt, A., Wurtzel, J. B., Xu, J., Yamamoto, M., and Zhang, Y. G.: Site U1482, Western Pacific Warm Pool, edited by: Rosenthal, Y., Holbourn, A. E., and Kulhanek, D. K., Proceedings of the International Ocean Discovery Program, 363: College Station, TX (International Ocean Discovery Program), https://doi.org/10.14379/iodp.proc.363.103.2018, 2018.
Saito T., Burckle L. H., and Hays J. D.: Late Miocene to Pleistocene biostratigraphy of equatorial Pacific sediments, in: Late Neogene Epoch Boundaries, edited by: Saito, T., New York, American Museum of Natural History, 226–244, 1975.
Schiebel, R. and Hemleben, C.: Planktic foraminifers in the modern ocean, Berlin, Springer, 1–358, 2017.
Schmidt, D. N., Thierstein, H. R., Bollmann, J., and Schiebel, R.: Abiotic forcing of plankton evolution in the Cenozoic, Science, 303, 207–210, 2004a.
Schmidt, D. N., Thierstein, H. R., and Bollmann, J.: The evolutionary history of size variation of planktic foraminiferal assemblages in the Cenozoic, Palaeogeogr. Palaeocl., 212, 159–180, 2004b.
Schmidt, D. N., Caromel, A. G. M., Seki, O., Rae, J. W. B., and Renaud, S.: Morphological response of planktic foraminifers to habitat modifications associated with the emergence of the Isthmus of Panama, Mar. Micropaleontol., 128, 28–38, 2016.
Scott, G. H.: Tempo and stratigraphic record of speciation in Globorotalia puncticulata, J. Foramin. Res., 12, 1–12, 1982.
Scott, G. H., Bishop, S., and Burt, B. J.: Guide to some Neogene Globorotalids (Foraminiferida) from New Zealand, New Zealand Geological Survey, 1990.
Seki, O., Foster, G. L., Schmidt, D. N., Mackensen, A., Kawamura, K., and Pancost, R. D.: Alkenone and boron-based Pliocene pCO2 records, Earth Planet. Sc. Lett., 292, 201–211, 2010.
Seki, O., Schmidt, D. N., Schouten, S., Hopmans, E. C., Sinninghe Damsté, J. S., and Pancost, R. D.: Paleoceanographic changes in the Eastern Equatorial Pacific over the last 10 Myr, Paleoceanography, 27, 2012.
Sellén, E., O'Regan, M., and Jakobsson, M.: Spatial and temporal Arctic Ocean depositional regimes: a key to the evolution of ice drift and current patterns, Quaternary Sci. Rev., 29, 3644–3664, 2010.
Sexton, P. F. and Norris, R. D.: High latitude regulation of low latitude thermocline ventilation and planktic foraminifer populations across glacial–interglacial cycles, Earth Planet. Sc.e Lett., 311, 69–81, 2011.
Sexton, P. F., Wilson, P. A., and Pearson, P. N.: Microstructural and geochemical perspectives on planktic foraminiferal preservation: “Glassy” versus “Frosty”, Geochem. Geophy. Geosy., 7, Q12P19, https://doi.org/10.1029/2006GC001291, 2006.
Shackleton, N. J., Backman, J., Zimmerman, H. T., Kent, D. V., Hall, M. A., Roberts, D. G., Schnitker, D., Baldauf, J. G., Desprairies, A., Homrighausen, R., and Huddlestun, P.: Oxygen isotope calibration of the onset of ice-rafting and history of glaciation in the North Atlantic region, Nature, 307, 620–623, 1984.
Shackleton, N. J., Hall, M. A., and Pate, D.: 15. Pliocene stable isotope stratigraphy of Site 846, in: Proc. Ocean Drill. Program Sci. Results, 138, 337–355, 1995.
Shaw, J. O., D'haenens, S., Thomas, E., Norris, R. D., Lyman, J. A., Bornemann, A., and Hull, P. M.: Photosymbiosis in planktonic foraminifera across the Paleocene–Eocene thermal maximum, Paleobiology, 47, 632–647, 2021.
Shevenell, A. E., Kennett, J. P., and Lea, D. W.: Middle Miocene southern ocean cooling and Antarctic cryosphere expansion, Science, 305, 1766–1770, 2004.
Si, W. and Aubry, M. P.: Vital effects and ecologic adaptation of photosymbiont-bearing planktonic foraminifera during the Paleocene-Eocene thermal maximum, implications for paleoclimate, Paleoceanogr. Paleocl., 33, 112–125, 2018.
Siccha, M. and Kucera, M.: ForCenS, a curated database of planktonic foraminifera census counts in marine surface sediment samples, Sci. Data, 4, 1–12, 2017.
Slater, G. J., Goldbogen, J. A., and Pyenson, N. D.: Independent evolution of baleen whale gigantism linked to Plio-Pleistocene ocean dynamics, Proc. Roy. Soc. B, 284, 20170546, https://doi.org/10.1098/rspb.2017.0546, 2017.
Sosdian, S. M. and Lear, C. H.: Initiation of the western Pacific warm pool at the middle Miocene climate transition?, Paleoceanogr. Paleocl., 35, e2020PA003920, https://doi.org/10.1029/2020PA003920, 2020.
Spero, H. J.: Do planktic foraminifera accurately record shifts in the carbon isotopic composition of seawater ΣCO2?, Mar. Micropaleontol., 19, 275–285, 1992.
Spero, H. J. and Lea, D. W.: Intraspecific stable isotope variability in the planktic foraminifera Globigerinoides sacculifer: Results from laboratory experiments, Mar. Micropaleontol., 22, 221–234, 1993.
Spero, H. J. and Williams, D. F.: Extracting Environmental information from planktonic foraminiferal delta-C-13 data, Nature, 335, 717–719, 1988.
Spero, H. J. and Williams, D. F.: Opening the carbon isotope “vital effects” black box, 1. Seasonal temperatures in the euphotic zone, Paleoceanography, 4, 593–601, 1989.
Spero, H. J., Leche, I., and Williams, D. F.: Opening the carbon isotope “vital affects” box, 2, quantitative model for interpreting foraminiferal carbon isotope data, Paleoceanography 6, 639–655, 1991.
Spezzaferri, S., Coxall, H. K., Olsson, R. K., Hemleben, C., and Wade, B.: Taxonomy, biostratigraphy, and phylogeny of Oligocene Globigerina, Globigerinella, and Quiltyella n. gen., in: Atlas of Oligocene Planktonic Foraminifera, edited by: Wade, B. S., Olsson, R. K., Pearson, P. N., Huber, B. T., and Berggren, W. A., 179–214, 2018.
Steinthorsdottir, M., Coxall, H. K., De Boer, A. M., Huber, M., Barbolini, N., Bradshaw, C. D., Burls, N. J., Feakins, S. J., Gasson, E., Henderiks, J., and Holbourn, A. E.: The Miocene: the future of the past, Paleoceanography and Paleoclimatology, 36, e2020PA004037, https://doi.org/10.1029/2020PA004037, 2021.
Steph, S., Tiedemann, R., Groeneveld, J., Sturm, A., and Nürnberg, D.: Pliocene changes in tropical east Pacific upper ocean stratification: Response to tropical gateways?, in: Proceedings of the Ocean Drilling Program: Scientific Results, 202, 1–51, 2006.
Steph, S., Tiedemann, R., Prange, M., Groeneveld, J., Schulz, M., Timmermann, A., Nürnberg, D., Rühlemann, C., Saukel, C., and Haug, G. H.: Early Pliocene increase in thermohaline overturning: A precondition for the development of the modern equatorial Pacific cold tongue, Paleoceanography, 25, PA2202, https://doi.org/10.1029/2008PA001645, 2010.
Thompson, P.: Foraminifers from the Middle America Trench, in: Initial reports of the Deep Sea Drilling Project, 67, Washington, D.C., U.S. Government Printing Office, 351–381, 1982.
Thunell, R.: Late Miocene–early Pliocene planktonic foraminiferal biostratigraphy and paleoceanography of low-latitude marine sequences, Mar. Micropaleontol., 6, 71–90, 1981.
Tierney, J. E., Poulsen, C. J., Montañez, I. P., Bhattacharya, T., Feng, R., Ford, H. L., Hönisch, B., Inglis, G. N., Petersen, S. V., Sagoo, N., and Tabor, C. R.: Past climates inform our future, Science, 370, eaay3701, https://doi.org/10.1126/science.aay3701, 2020.
Tindall, J. C. and Haywood, A. M.: Modeling oxygen isotopes in the Pliocene: Large-scale features over the land and ocean, Paleoceanography, 30, 1183–1201, 2015.
Tittensor, D. P., Mora, C., Jetz, W., Lotze, H. K., Ricard, D., Berghe, E. V., and Worm, B.: Global patterns and predictors of marine biodiversity across taxa, Nature, 466, 1098–1101, 2010.
Todd, C. L., Schmidt, D. N., Robinson, M. M., and De Schepper, S.: Planktic foraminiferal test size and weight response to the late Pliocene environment, Paleoceanogr. Paleocl., 35, e2019PA003738, https://doi.org/10.1029/2019PA003738, 2020.
Uchikawa, J. and Zeebe, R. E.: Examining possible effects of seawater pH decline on foraminiferal stable isotopes during the Paleocene-Eocene Thermal Maximum, Paleoceanography, 25, PA2216, https://doi.org/10.1029/2009PA001864, 2010.
Ujiié, Y. and Ishitani, Y.: Evolution of a planktonic foraminifer during environmental changes in the tropical oceans, PLoS One, 11, e0148847, https://doi.org/10.1371/journal.pone.0148847, 2016.
Ujiié, Y., de Garidel-Thoron, T., Watanabe, S., Wiebe, P., and de Vargas, C.: Coiling dimorphism within a genetic type of the planktonic foraminifer Globorotalia truncatulinoides, Mar. Micropaleontol., 77, 145–153, 2010.
Urban, M. C.: Accelerating extinction risk from climate change, Science, 348, 571–573, https://doi.org/10.1126/science.aaa4984, 2015.
Vincent, E., Killingley, J. S., and Berger, W. H.: Miocene oxygen and carbon isotope stratigraphy of the tropical Indian Ocean, in: The Miocene Ocean: Paleoceanography and Biogeography, 163, 103–130, Geological Society of America Memoir 163, 1985.
Wade, B. S. and Olsson, R. K.: Investigation of pre-extinction dwarfing in Cenozoic planktonic foraminifera, Palaeogeogr. Palaeocl., 284, 39–46, 2009.
Wade, B. S. and Pearson, P. N.: Planktonic foraminiferal turnover, diversity fluctuations and geochemical signals across the Eocene/Oligocene boundary in Tanzania, Mar. Micropaleontol., 68, 244–255, 2008.
Wade, B. S., Al-Sabouni, N., Hemleben, C., and Kroon, D.: Symbiont bleaching in fossil planktonic foraminifera, Evol. Ecol., 22, 253–265, 2008.
Wade, B. S., Pearson, P. N., Berggren, W. A., and Pälike, H.: Review and revision of Cenozoic tropical planktonic foraminiferal biostratigraphy and calibration to the geomagnetic polarity and astronomical time scale, Earth-Sci. Rev., 104, 111–142, 2011.
Wade, B. S., Poole, C. R., and Boyd, J.: Giantism in Oligocene planktonic foraminifera Paragloborotalia opima: Morphometric constraints from the equatorial Pacific Ocean, Newsl. Stratigr., 49, 421–444, 2016.
Wade, B. S., Pearson, P. N., Olsson, R. K., Fraass, A. J., Leckie, R. M., and Hemleben, C.: Taxonomy, biostratigraphy, and phylogeny of Oligocene and lower Miocene Dentoglobigerina and Globoquadrina, in: Atlas of Oligocene Planktonic Foraminifera, edited by: Wade, B. S., Olsson, R. K., Pearson, P. N., Huber, B. T., and Berggren, W. A., 331–384, 2018.
Waelbroeck, C., Lougheed, B. C., Riveiros, N. V., Missiaen, L., Pedro, J., Dokken, T., Hajdas, I., Wacker, L., Abbott, P., Dumoulin, J. P., and Thil, F.: Consistently dated Atlantic sediment cores over the last 40 thousand years, Sci. Data, 6, 1–12, 2019
Wallace, L. M., Saffer, D. M., Barnes, P. M., Pecher, I. A., Petronotis, K. E., LeVay, L. J., Bell, R. E., Crundwell, M. P., Engelmann de Oliveira, C. H., Fagereng, A., Fulton, P. M., Greve, A., Harris, R. N., Hashimoto, Y., Hüpers, A., Ikari, M. J., Ito, Y., Kitajima, H., Kutterolf, S., Lee, H., Li, X., Luo, M., Malie, P. R., Meneghini, F., Morgan, J. K., Noda, A., Rabinowitz, H. S., Savage, H. M., Shepherd, C. L., Shreedharan, S., Solomon, E.A., Underwood, M. B., Wang, M., Woodhouse, A. D., Bourlange, S. M., Brunet, M. M. Y., Cardona, S., Clennell, M. B., Cook, A. E., Dugan, B., Elger, J., Gamboa, D., Georgiopoulou, A., Han, S., Heeschen, K. U., Hu, G., Kim, G. Y., Koge, H., Machado, K. S., McNamara, D. D., Moore, G. F., Mountjoy, J. J., Nole, M. A., Owari, S., Paganoni, M., Rose, P. S., Screaton, E. J., Shankar, U., Torres, M. E., Wang, X., and Wu, H.-Y.: Expedition 372B/375 methods, in: Hikurangi Subduction Margin Coring, Logging, and Observatories, edited by: Wallace, L. M., Saffer, D. M., Barnes, P. M., Pecher, I. A., Petronotis, K. E., LeVay, L. J., and the Expedition 372/375 Scientists, Proceedings of the International Ocean Discovery Program, 372B/375: College Station, TX (International Ocean Discovery Program), To investigate how planktonic 2019.
Weiner, A., Aurahs, R., Kurasawa, A., Kitazato, H., and Kucera, M.: Vertical niche partitioning between cryptic sibling species of a cosmopolitan marine planktonic protist, Mol. Ecol., 21, 4063–4073, 2012.
Weiner, A. K., Weinkauf, M. F., Kurasawa, A., Darling, K. F., Kucera, M., and Grimm, G. W.: Phylogeography of the tropical planktonic foraminifera lineage Globigerinella reveals isolation inconsistent with passive dispersal by ocean currents, PLoS One, 9, e92148, https://doi.org/10.1371/journal.pone.0092148, 2014.
Weinkauf, M. F., Moller, T., Koch, M. C., and Kučera, M.: Disruptive selection and bet-hedging in planktonic Foraminifera: Shell morphology as predictor of extinctions, Front. Ecol. Evol., 2, 64, https://doi.org/10.3389/fevo.2014.00064, 2014.
Weinkauf, M. F., Bonitz, F. G., Martini, R., and Kučera, M.: An extinction event in planktonic Foraminifera preceded by stabilizing selection, PloS one, 14, e0223490, https://doi.org/10.1371/journal.pone.0223490, 2019.
Westerhold, T., Marwan, N., Drury, A. J., Liebrand, D., Agnini, C., Anagnostou, E., Barnet, J. S., Bohaty, S. M., De Vleeschouwer, D., Florindo, F., and Frederichs, T.: An astronomically dated record of Earth's climate and its predictability over the last 66 million years, Science, 369, 1383–1387, 2020.
Willeit, M., Ganopolski, A., Calov, R., Robinson, A., and Maslin, M.: The role of CO2 decline for the onset of Northern Hemisphere glaciation, Quaternary Sci. Rev., 119, 22–34, 2015.
Williams, M., Haywood, A. M., Hillenbrand, C. D., and Wilkinson, I. P.: Efficacy of δ18O data from Pliocene planktonic foraminifer calcite for spatial sea surface temperature reconstruction: comparison with a fully coupled ocean–atmosphere GCM and fossil assemblage data for the mid-Pliocene, Geol. Mag., 142, 399–417, 2005.
Winter, C. J. and Pearson, P. N.: Coiling directions in some Miocene planktonic Foraminifera, J. Micropalaeontol., 20, 29–30, 2001.
Woodhouse, A., Jackson, S. L., Jamieson, R. A., Newton, R. J., Sexton, P. F., and Aze, T.: Adaptive ecological niche migration does not negate extinction susceptibility, Sci. Rep., 11, 1–10, 2021.
Woodhouse, A., Swain, A., Fagan, W. F., Fraass, A. J., and Lowery, C. M.: Late Cenozoic cooling restructured global marine plankton communities, Nature, in press, 2023.
Worm, B., Lotze, H. K., and Myers, R. A.: Predator diversity hotspots in the blue ocean, P. Natl. Acad. Sci. USA, 100, 9884–9888, 2003.
Yang, H. and Wang, F.: Revisiting the thermocline depth in the equatorial Pacific, J. Clim., 22, 3856–3863, 2009.
Zhang, X., Prange, M., Steph, S., Butzin, M., Krebs, U., Lunt, D.J., Nisancioglu, K. H., Park, W., Schmittner, A., Schneider, B., and Schulz, M.: Changes in equatorial Pacific thermocline depth in response to Panamanian seaway closure: Insights from a multi-model study, Earth Planet. Sc. Lett., 317, 76–84, 2012.
Zika, J. D., Skliris, N., Blaker, A. T., Marsh, R., Nurser, A. G., and Josey, S. A.: Improved estimates of water cycle change from ocean salinity: the key role of ocean warming, Environ. Res. Lett., 13, 074036, https://doi.org/10.1088/1748-9326/aace42, 2018.
Zou, S., Groeneveld, J., Giosan, L., and Steinke, S.: Determining the habitat depth of the planktic foraminifera Dentoglobigerina altispira in the eastern Arabian Sea during the middle Miocene, Mar. Micropaleontol., 170, 102075, https://doi.org/10.1016/j.marmicro.2021.102075, 2022.