the Creative Commons Attribution 4.0 License.
the Creative Commons Attribution 4.0 License.
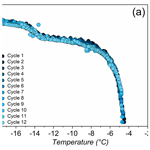
Lichen species across Alaska produce highly active and stable ice nucleators
Rosemary J. Eufemio
Ingrid de Almeida Ribeiro
Todd L. Sformo
Gary A. Laursen
Valeria Molinero
Janine Fröhlich-Nowoisky
Mischa Bonn
Konrad Meister
Forty years ago, lichens were identified as extraordinary biological ice nucleators (INs) that enable ice formation at temperatures close to 0 ∘C. By employing INs, lichens thrive in freezing environments that surpass the physiological limits of other vegetation, thus making them the majority of vegetative biomass in northern ecosystems. Aerosolized lichen INs might further impact cloud glaciation and have the potential to alter atmospheric processes in a warming Arctic. Despite the ecological importance and formidable ice nucleation activities, the abundance, diversity, sources, and role of ice nucleation in lichens remain poorly understood. Here, we investigate the ice nucleation capabilities of lichens collected from various ecosystems across Alaska. We find ice nucleating activity in lichen to be widespread, particularly in the coastal rainforest of southeast Alaska. Across 29 investigated lichen, all species show ice nucleation temperatures above −15 ∘C, and ∼30 % initiate freezing at temperatures above −6 ∘C. Concentration series of lichen ice nucleation assays in combination with statistical analysis reveal that the lichens contain two subpopulations of INs, similar to previous observations in bacteria. However, unlike the bacterial INs, the lichen INs appear as independent subpopulations resistant to freeze–thaw cycles and against temperature treatment. The ubiquity and high stability of the lichen INs suggest that they can impact local atmospheric processes and that ice nucleation activity is an essential trait for their survival in cold environments.
- Article
(1478 KB) - Full-text XML
- BibTeX
- EndNote
The formation of ice is thermodynamically favored at temperatures below 0 ∘C, but the crystallization is kinetically hindered. As a result, pure water droplets can be supercooled to temperatures as low as −38 ∘C, below which homogeneous ice nucleation occurs (Koop et al., 2000). In natural systems, water usually freezes in a heterogeneous process facilitated by the presence of particles that serve as ice nucleators (INs). INs can be of biotic and abiotic origins (Maki and Willoughby, 1978; Wilson et al., 2003; Murray et al., 2012) and play fundamental roles in high-altitude cloud formation, in triggering precipitation in mixed-phase clouds, and assist in the survival of freeze-tolerant organisms (Zachariassen and Kristiansen, 2000). Potent biological INs have been discovered in various life forms, including bacteria, fungi, algae, plants, animals, and lichen (Maki et al., 1974; Kieft and Lindow, 1988; Pouleur et al., 1992; Lundheim, 2002; Fröhlich-Nowoisky et al., 2015). The most efficient and best-characterized biological INs are the plant-associated bacteria Pseudomonas syringae (Kozloff et al., 1983; Govindarajan and Lindow, 1988). The ability of P. syringae to facilitate ice formation is attributed to specialized ice nucleating proteins (INPs) anchored to their outer bacterial cell membrane (Govindarajan and Lindow, 1988). P. syringae INPs form functional aggregates that are categorized into classes A–C, depending on their activation temperature and assembly size (Turner et al., 1990; Lukas et al., 2020). Class C consists of small clusters of INPs and is responsible for freezing at approximately −7.5 ∘C, while class A induces freezing at temperatures as warm as −2 ∘C. Class B INs are rarely observed and responsible for freezing between and ∘C (Schwidetzky et al., 2021b).
Besides bacteria, lichens have been identified as extraordinary INs (Kieft and Lindow, 1988; Honegger, 2007; Moffett et al., 2015). Given the global abundance of lichen (Honegger, 2007), airborne lichen-derived INs have been proposed to contribute to the pool of atmospheric INs and to strongly influence atmospheric processes (Moffett et al., 2015; Creamean et al., 2021). Lichens depend on the atmosphere for moisture, and the ability to facilitate ice formation has been proposed to be beneficial to scavenge water vapor in cold environments (Kieft and Lindow, 1988). Of the lichen species previously tested, the majority were ice nucleation active, indicating that ice nucleation activity in lichens is ubiquitous (Kieft and Lindow, 1988; Moffett et al., 2015). Lichen IN activity was primarily attributed to the mycobiont (fungal symbiont), and the photobiont partner was proposed to be relatively inactive (Kieft and Ahmadjian, 1989). Efforts to identify and characterize lichen INs revealed that they are active without lipids and very pH- and heat-stable (Kieft and Ahmadjian, 1989; Kieft and Ruscetti, 1990). Studies on non-lichenized fungi further suggested that proteinaceous compounds are often responsible for their ice nucleation activity (Kieft and Ruscetti, 1990; Lundheim, 2002; Kunert et al., 2019; Fröhlich-Nowoisky et al., 2015; Moffett et al., 2015). However, while non-lichenized fungal species like Fusarium have been extensively investigated for their IN activity and overall influence on the environment and atmosphere (Fröhlich-Nowoisky et al., 2015; Kunert et al., 2019; Yang et al., 2022), our understanding of the IN activity of lichenized fungi and the prevalence of ice nucleation among lichen species remains limited (Kieft and Ruscetti, 1990; Henderson-Begg et al., 2009; Moffett et al., 2015).
Here, we surveyed a taxonomically diverse selection of lichen species from across Alaska for IN activity. We used the high-throughput twin-plate ice nucleation assay (TINA) to quantify INs of selected lichen species, and the underlying distribution of INs was determined using numerical modeling of the cumulative freezing spectra. Further, we investigated the stability of lichen INs upon exposure to freeze–thaw cycles and heat treatments, to gain insights into possible atmospheric influences of airborne lichen INs and their usage in cryostorage applications.
2.1 Sampling
Samples were obtained from four regions in Alaska, USA, between September 2021 and February 2022. Nineteen lichens were collected from rock and tree bark substrates in Juneau, three in Kodiak, five in Utqiaġvik, and two in Fairbanks. These locations encompassed a range of biomes, from coastal rainforests in Juneau and Kodiak, to Arctic tundra in Utquiġvik, and to the boreal forest in Fairbanks (Simpson et al., 2007). Lichen samples were collected based on availability in the natural environment and feasibility of access to collection sites. We exclusively sampled foliose and fruticose lichens. These species protrude off their substrates in leaf- and hair-like structures, which enabled accurate identification based on physical features. Species were identified using vegetation identification guides (Pojar and MacKinnon, 1994). Samples were collected in sterile containers and stored at −18 ∘C prior to analysis.
2.2 Purification of aqueous extracts
To prepare extracts for quantitative analysis and temperature experiments, all samples were washed with pure water (Millipore Milli-Q® Simplicity 185 Water Purification System, Merck KGaA, Darmstadt, Germany) to minimize contamination from external sources of INs. Extracts were prepared for ice nucleation assays and stability experiments using a standardized procedure adapted from Kieft and Lindow (Kieft and Lindow, 1988). First, a 5 mL aliquot of pure water was added to 2 g of lichen. Secondly, the samples were ground to a fine pulp using a mortar and pestle. This procedure was chosen to ensure that the aqueous extract contained all the molecules present in the lichen samples, both the molecules bound to the cell wall and any secreted molecules (Kieft and Lindow, 1988). Samples were then centrifuged at 5000 rpm for 10 min. The supernatants were filtered through 0.22 µm pore diameter syringe filters (Millex® Syringe Filter, Merck KGaA, Darmstadt, Germany) to remove cellular debris. The aqueous extracts were immediately tested for ice nucleation activity using the Vali-type (Vali, 1971) apparatus and stored at −18 ∘C until systematic analysis. Lichen extracts remained frozen for up to 9 months.
2.3 Initial screening for ice nucleation activity
For initial screenings of lichen ice nucleation activity, a Vali-type apparatus was used, which consists of a temperature-controlled aluminum cold plate (Linkam Scientific Instruments LTD, United Kingdom). For each sample, 20 extracted 1 µL droplets were cooled at a rate of 3 ∘C min−1, and freezing events were identified through the optical change in droplet appearance consistent with freezing. The freezing temperature for each droplet was recorded, and the temperature at which half of the droplets are frozen, T50, was used as a direct measure for the efficacy of the INs. Snomax (inactivated P. syringae; Snomax Int) was used as a positive control, resulting in a T50 of −3.5 ∘C at 1.0 mg mL−1, while pure water had a T50 of −15 ∘C.
2.4 Freezing spectra (number of IN)
The three most active lichen species, Platismatia herrei, Sphaerophorus globosus, and Peltigera britannica, were analyzed using the high-throughput twin-plate ice nucleation assay (TINA) (Kunert et al., 2018). In TINA measurements, a liquid handling station (epMotion ep5073, Eppendorf, Hamburg, Germany) was used to serially dilute the aqueous extracts in 10-fold increments with pure water, each dilution consisting of 96 (3 µL) droplets in two 384-well plates. The 384-well plates were cooled at a continuous rate of 1 ∘C min−1 from 0 to −30 ∘C. Freezing temperatures for each droplet were extracted, and the fraction of frozen droplets was calculated for the different temperatures. The cumulative number of active INs per mass unit of sample (Nm) in each dilution was calculated using Vali's equation (Vali, 1971).
2.5 Modeling the underlying distribution of freezing temperatures
The heterogeneous underlying-based (HUB) method and associated HUB-backward numerical code (de Almeida Ribeiro et al., 2022) were used to interpret the heterogeneous ice nucleation temperatures obtained from TINA measurements of the lichen samples. The HUB method relies on the assumptions of Vali (1971); e.g., that each IN has a distinct nucleation temperature, and that the IN with the highest nucleation temperature is responsible for the freezing of the droplet in the cooling experiments. The HUB-backwards stochastic optimization procedure was used to extract the differential spectrum Nm(T) and determine the underlying distribution of ice nuclei subpopulations in the cumulative freezing spectrum Nm(T) of lichen. The interpretability of the results in terms of subpopulations provides an advantage over polynomial fitting and differentiation of Nm(T).
2.6 Stability of ice nucleators
Freeze–thaw cycles and heat treatments were performed on selected lichen extracts and Snomax, to assess the stability of INs exposed to temperature changes. For freeze–thaw cycles, aliquots of lichen extract were consecutively frozen by gradually cooling to −30 ∘C at 1 ∘C min−1 and thawed to room temperature 12 times over the course of 12 h. After each temperature cycle, the activity was determined using TINA. For heat treatment experiments, the aliquots of the lichen extracts were incubated at 98 ∘C for 1 h, and the ice nucleation activity before and after heating was determined using TINA.
3.1 IN-active lichen species
Table 1 shows the freezing temperatures of 29 lichen extracts as determined in initial studies by a Vali-type droplet freezing assay and 16 of the lichen extracts as measured by TINA. Freezing temperatures are shown as T50 values and are defined as the temperatures at which 50 % of the undiluted lichen extract droplets are frozen. We find that all Alaskan lichen species collected between September 2021 and February 2022 froze at temperatures ranging from −5 to −14.5 ∘C. These findings are consistent with previous reports of widespread ice nucleation activity in lichen (Kieft and Lindow, 1988; Henderson-Begg et al., 2009; Moffett et al., 2015; Creamean et al., 2021). The ice nucleation activity of the collected lichen does not seem to increase from warmer (e.g., Juneau) to colder (e.g., Utqiaġvik) climate zones, and none of the lichen species collected in the Arctic tundra (Utqiaġvik) showed freezing temperatures warmer than −7.6 ∘C. Moreover, lichens with the highest ice nucleation activity (e.g., Platismatia herrei) coexist in the same coastal rainforest environment and even on the same tree substratum as lichens with relatively low ice nucleation activity (e.g., Usnea longissima). Among the 29 lichen, Platismatia herrei, Peltigera britannica, and Sphaerophorus globosus showed notably high IN activity in initial screenings, with T50 values of −5.2, −5.7, and −6 ∘C, respectively. To better characterize the INs of these lichen species, we investigated their INs using the TINA setup, which allows the simultaneous measurement of a complete dilution series with robust statistics (Kunert et al., 2018).
Table 1Ice nucleation activity of undiluted lichen extracts determined using a Vali-type (initial) and high-throughput ice nucleation assay (TINA). Freezing temperatures are shown as T50 values and are defined as the temperatures at which 50 % of the undiluted lichen extract droplets are frozen. Lichen samples were collected across Alaska, with 19 lichens from coastal rainforests in Juneau (J) and three from Kodiak (K), five from the Arctic tundra in Utqiaġvik (U), and two from boreal forests in Fairbanks (F). Extracts labeled N/A were not measured using TINA. Species are listed from high to low ice nucleation activity.
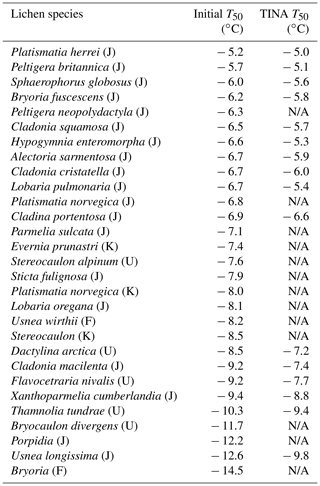
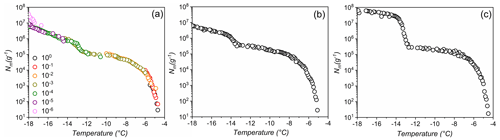
Figure 1Freezing experiments of aqueous extracts containing lichen INs from P. britannica (a), S. globosus (b), and P. herrei (c). Symbol colors in (a) indicate data from different concentrations and are identical to uncolored dilutions shown in (b) and (c). Shown are the cumulative number of IN per unit mass (Nm) for extracts containing INs from lichen.
Figure 1 shows the results of TINA measurements of untreated aqueous lichen extracts of P. britannica, S. globosus, and P. herrei. The initial solutions had a concentration of 0.4 g mL−1 and were then serially diluted 10-fold. The cumulative IN number concentration (Nm) was calculated using Vali's formula and represents the number of INs per unit weight that are active above a certain temperature (Vali, 1971). We find that for the three lichen species, the freezing spectra show two strong increases in Nm(T) around −4.5 and −13 ∘C, with plateaus between and ∘C and below ∘C. The two rises in the spectra suggest the presence of two classes of INs with different activation temperatures, while the plateaus indicate that fewer INs are active within those temperature ranges (Budke and Koop, 2015; Lukas et al., 2020). The presence of two IN subpopulations is notable, given that quantitative measurements of fungi-associated INs typically display only one IN population (Fröhlich-Nowoisky et al., 2015; Kunert et al., 2019). Two rises and subsequent plateaus in the freezing spectra are more characteristic of those observed in the spectrum of P. syringae (Fig. 3b). For P. syringae, the increases at and −7.5 ∘C were assigned to two classes of INs that consist of different aggregate sizes of the same INPs (Turner et al., 1990; Qiu et al., 2019; Schwidetzky et al., 2021a). The classes and molecular composition of lichen INs have not been identified, but experimental results of Kieft and Ahmadjian (1989) have previously identified the lichen mycobiont as responsible for freezing above −5 ∘C, while the photobiont has much lower activity. We therefore tentatively assign the two increases in the freezing spectra of the lichens to INs of the mycobiont and photobiont partners. However, we cannot exclude the possibility that lichen INs are exclusively mycobiont-derived macromolecules that aggregate similarly to bacterial INs, and thus induce freezing at different activation temperatures depending on the aggregate size. Given the uncertainty, we refer to the first IN subpopulation active at −4.5 ∘C as class 1 and the second class active at −13 ∘C as class 2.
3.2 Underlying distribution of lichen IN
We employed the heterogeneous underlying-based (HUB) stochastic optimization analysis (de Almeida Ribeiro et al., 2022) to better identify and characterize the underlying number of IN subpopulations in lichen. Figure 2a shows the experimentally obtained cumulative spectrum Nm(T) of P. herrei, together with the differential spectrum predicted by HUB from the optimized distribution of nucleation temperatures (e.g., the differential spectrum), shown in Fig. 2b. The two IN subpopulations in the differential spectrum are centered at − 6.8 and − 13.8 ∘C (Fig. 2b). We assign the mode at − 6.8 ∘C to the class 1 INs and the mode at − 13.8 ∘C to class 2 INs. The differential spectrum shows that freezing at − 6.8 ∘C is two orders of magnitude less likely to happen than freezing at − 13.8 ∘C. The robustness of these signals and their physical nature require further investigation.
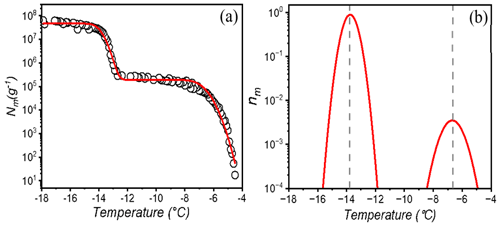
Figure 2Freezing experiments of aqueous extracts containing lichen INs from P. herrei. (a) Cumulative number of INs per unit mass of P. herrei (Nm) for extracts containing INs from lichen. The red line represents the optimized cumulative spectrum obtained through the HUB-backward code. (b) Differential spectrum that represents the underlying distribution of heterogenous freezing temperatures that produces the cumulative number of INs per unit mass (shown with the red line in panel a). The grey dashed lines indicate the temperatures that give the modes of the distribution. The mode, spread, and weight of the class 1 IN subpopulation are − 6.8 and 0.67 ∘C and 0.95 %, respectively. For the class 2 IN subpopulation, the mode, spread, and weight are − 13.8 and 0.29 ∘C and 99.05 %.
3.3 Stability of lichen IN
The lichen INs are exposed to physical stressors such as low, high, or quickly changing temperatures in the natural environment or as aerosolized particles. To investigate the stability of lichen INs, we performed freeze–thaw cycles and heat treatments. Figure 3 displays ice nucleation measurements of aqueous extracts of P. herrei and a solution of Snomax (inactivated P. syringae) in water during 12 consecutive freeze–thaw cycles. We find that the freezing spectra of P. herrei look similar across all cycles, with nucleation events occurring consistently at and −13 ∘C and plateaus between and −13 ∘C. In contrast to lichen INs, the freezing spectra of P. syringae show a systematic trend in which the final cycle differs markedly from the first. We find that the rise at ∘C in the bacterial Nm spectrum is reduced and shifted to slightly lower temperatures with progressing cycles. The second rise at ∘C shifts not only to lower temperatures but also increases over time. Evidently, repetitive freeze–thaw cycles reduce the activity of the class A INs (larger INP aggregates active at ∘C) and transform them into the less active class C INs (smaller aggregates active at ∘C). These findings are consistent with the apparent instability of bacterial INs (Polen et al., 2016), and contrast the high stability of the lichen INs.
The repetitive freezing measurements further reveal that the highest activity of lichen IN was already developed during the initial preparation of the extracts and that no equilibration time was required. The storage of aqueous extracts at −18 ∘C prior to freeze–thaw cycles also did not impact the ice nucleation activity of the highly active class 1 INs, given that T50 values of the initial measurements and up to 9 months later are similar. These findings indicate that lichen INs can undergo multiple freeze–thaw cycles without losing significant activity and would be reusable in freeze-tolerant lichens and able to influence cloud glaciation.
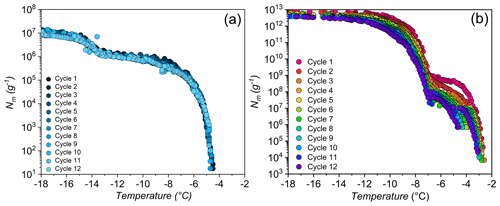
Figure 3Effects of freeze–thaw cycles on bacterial and lichen IN activity. Shown are the cumulative numbers of INs per unit mass Nm of P. herrei (a) and Snomax (P. syrinage) (b).
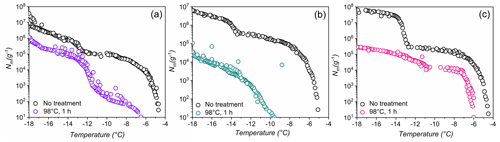
Figure 4Effects of high-temperature treatment on the ice nucleation activity and corresponding distributions of class 1 and class 2 INs of selected lichens.Shown are the cumulative number of INs (Nm) per gram of lichen sample of (a) P. britannica, (b) S. globosus, and (c) P. herrei.
As a second test of stability, Fig. 4 shows ice nucleation measurements of aqueous lichen extracts before and after 1 h of exposure to 98 ∘C. We find that the freezing spectra of all three lichen species are altered substantially after heat treatment. For P. britannica, we observe that the first rise at is lowered to −6.5 ∘C, while the second rise at −13 ∘C remains and becomes more apparent. Moreover, the total cumulative number of P. britannica INs decreases moderately from 107 to ∼106 g−1. For S. globosus, we observe a markedly different behavior: the first rise at decreases to −9.5 ∘C, while the second rise at −13 ∘C completely disappears. In addition, the total cumulative number of INs decreases significantly from 107 g−1 to less than 105g−1. For P. herrei, we find that the first rise at is only slightly lowered to −6 ∘C, while the second rise at −13 ∘C is absent. Clearly, the lichen INs are affected differently by heat treatment, suggesting distinct variation across the biomolecules responsible for ice nucleation in lichens. We conclude that the macromolecular composition of INs of class 1 and class 2 likely differ both within individual lichen and among lichen species.
The high stability of the class 2 INs after the 98 ∘C treatment points toward some non-proteinaceous ice nucleating molecules, and it has been proposed that some biological INs may consist of polysaccharides (Pummer et al., 2012; Dreischmeier et al., 2017). Given the lower stability of the class 1 INs upon heating, it is reasonable to assume that the ice nucleation activity is primarily due to proteinaceous compounds that denature under high temperatures. However, future experiments, including chemical analyses, are required to adequately characterize the macromolecules responsible for ice nucleation activity in lichen.
The abundance and distribution of ice nucleation activity within lichens were investigated in a study of 29 different species collected across Alaska. Among the tested lichen species, all showed IN activity above −15 ∘C and ∼30 % displayed ice nucleation activity above −6 ∘C, illustrating the wide distribution of IN activity within lichens in Alaska. Concentration series of the most active lichen INs in combination with statistical analysis revealed the presence of two clearly distinguishable classes of INs which we categorized as class 1 and class 2. We tentatively assigned class 1 IN activity to the fungal component of lichen and class 2 IN activity to the photobiont.
Freeze–thaw cycles and heat treatments revealed a high stability of some lichen INs, demonstrating the suitability of lichen for potential usage in cryostorage applications. The abundance of lichens in nature and the wide distribution of ice nucleation activity across species, together with the extraordinary stability of the INs under atmospherically relevant conditions, indicates that these IN may have considerable impact on precipitation patterns in northern environments. We speculate that lichenized fungi and their efficient INs are likely abundant members of atmospheric communities (Fröhlich-Nowoisky et al., 2009; Womack et al., 2015), especially over coastal rainforest canopies (Fröhlich-Nowoisky et al., 2012).
Our results further demonstrate that there are significant differences among lichen INs and that their molecular composition leads to variations in stability. Additional research is needed to characterize the lichen IN to evaluate the nature of the macromolecules responsible for ice nucleation and the abundance of lichen INs in environmental samples on a global scale.
All data are available from the corresponding author upon request.
KM and RJE designed the experiments. TLS provided lichen samples. TLS, RJE, and GAL identified lichen. RJE performed the experiments. IER and VM performed stochastic optimization analysis. RJM, KM, JFN, MB, TLS, and VM discussed the results. RJE and KM wrote the paper with contributions from all co-authors.
The contact author has declared that none of the authors has any competing interests.
Publisher’s note: Copernicus Publications remains neutral with regard to jurisdictional claims in published maps and institutional affiliations.
We are grateful to the MaxWater initiative from the Max Planck Society. Konrad Meister acknowledges support by the National Science Foundation under grant no. NSF 2116528 and from the Institutional Development Awards (IDeA) from the National Institute of General Medical Sciences of the National Institutes of Health under grant nos. P20GM103408 and P20GM109095. Ingrid de Almeida Ribeiro and Valeria Molinero gratefully acknowledge support by AFOSR through MURI award no. FA9550-20-1-0351. We thank Ashley Murphy, Louise Tymrak, Nadine Bothen, and Ralph Schwidetzky for helping with sample collection and TINA measurements, Michail Shurbenko for sample identification, and Thomas Hill and Bruce Moffett for exciting initial discussions.
This research has been supported by the Directorate for Biological Sciences, Division of Molecular and Cellular Biosciences (grant no. 2116528) and the Air Force Office of Scientific Research (grant no. FA9550-20-1-0351).
The article processing charges for this open-access publication were covered by the Max Planck Society.
This paper was edited by Paul Stoy and reviewed by two anonymous referees.
Budke, C. and Koop, T.: BINARY: an optical freezing array for assessing temperature and time dependence of heterogeneous ice nucleation, Atmos. Meas. Tech., 8, 689–703, https://doi.org/10.5194/amt-8-689-2015, 2015.
Creamean, J. M., Ceniceros, J. E., Newman, L., Pace, A. D., Hill, T. C. J., DeMott, P. J., and Rhodes, M. E.: Evaluating the potential for Haloarchaea to serve as ice nucleating particles, Biogeosciences, 18, 3751–3762, https://doi.org/10.5194/bg-18-3751-2021, 2021.
de Almeida Ribeiro, I., Meister, K., and Molinero, V.: HUB: A method to model and extract the distribution of ice nucleation temperatures from drop-freezing experiments, ChemRxiv, Cambridge, https://doi.org/10.26434/chemrxiv-2022-ddzv8, 2022.
Dreischmeier, K., Budke, C., Wiehemeier, L., Kottke, T., and Koop, T.: Boreal pollen contain ice-nucleating as well as ice-binding “antifreeze” polysaccharides, Sci. Rep., 7, 1–13, https://doi.org/10.1038/srep41890, 2017.
Fröhlich-Nowoisky, J., Pickersgill, D. A., Despré, V. R., and Pöschl, U.: High diversity of fungi in air particulate matter, P. Natl. Acad. Sci. USA, 106, 12814–12819, 2009.
Fröhlich-Nowoisky, J., Burrows, S. M., Xie, Z., Engling, G., Solomon, P. A., Fraser, M. P., Mayol-Bracero, O. L., Artaxo, P., Begerow, D., Conrad, R., Andreae, M. O., Després, V. R., and Pöschl, U.: Biogeography in the air: fungal diversity over land and oceans, Biogeosciences, 9, 1125–1136, https://doi.org/10.5194/bg-9-1125-2012, 2012.
Fröhlich-Nowoisky, J., Hill, T. C. J., Pummer, B. G., Yordanova, P., Franc, G. D., and Pöschl, U.: Ice nucleation activity in the widespread soil fungus Mortierella alpina, Biogeosciences, 12, 1057–1071, https://doi.org/10.5194/bg-12-1057-2015, 2015.
Govindarajan, A. G. and Lindow, S. E.: Size of bacterial ice-nucleation sites measured in situ by radiation inactivation analysis, P. Natl. Acad. Sci. USA, 85, 1334–1338, https://doi.org/10.1073/PNAS.85.5.1334, 1988.
Henderson-Begg, S. K., Hill, T., Thyrhaug, R., Khan, M., and Moffett, B. F.: Terrestrial and airborne non-bacterial ice nuclei, Atmos. Sci. Lett., 10, 215–219, https://doi.org/10.1002/ASL.241, 2009.
Honegger, R.: Water relations in lichens, in: Fungi in the Environment, edited by: Gadd, G. M., Watkinson, S. C., Dyer, P, Cambridge University Press, Cambridge, 185–200, https://doi.org/10.1017/CBO9780511541797.010, 2007.
Kieft, T. L. and Ahmadjian, V.: Biological Ice Nucleation Activity in Lichen Mycobionts and Photobionts, The Lichenologist, 21, 355–362, https://doi.org/10.1017/S0024282989000599, 1989.
Kieft, T. L. and Lindow, S.: Ice Nucleation Activity in Lichens, Appl. Environ. Microbiol., 54, 1678–1681, 1988.
Kieft, T. L. and Ruscetti, T.: Characterization of Biological Ice Nuclei from a Lichen, J. Bacteriol., 172, 3519–3523, 1990.
Koop, T., Luo, B., Tsias, A., and Peter, T.: Water activity as the determinant for homogeneous ice nucleation in aqueous solutions, Nature, 406, 611–614, https://doi.org/10.1038/35020537, 2000.
Kozloff, L. M., Schofield, M. A., and Lute, M.: Ice nucleating activity of Pseudomonas syringae and Erwinia herbicola, J. Bacteriol., 153, 222–231, https://doi.org/10.1128/JB.153.1.222-231.1983, 1983.
Kunert, A. T., Lamneck, M., Helleis, F., Pöschl, U., Pöhlker, M. L., and Fröhlich-Nowoisky, J.: Twin-plate Ice Nucleation Assay (TINA) with infrared detection for high-throughput droplet freezing experiments with biological ice nuclei in laboratory and field samples, Atmos. Meas. Tech., 11, 6327–6337, https://doi.org/10.5194/amt-11-6327-2018, 2018.
Kunert, A. T., Pöhlker, M. L., Tang, K., Krevert, C. S., Wieder, C., Speth, K. R., Hanson, L. E., Morris, C. E., Schmale III, D. G., Pöschl, U., and Fröhlich-Nowoisky, J.: Macromolecular fungal ice nuclei in Fusarium: effects of physical and chemical processing, Biogeosciences, 16, 4647–4659, https://doi.org/10.5194/bg-16-4647-2019, 2019.
Lukas, M., Schwidetzky, R., Kunert, A. T., Pöschl, U., Fröhlich-Nowoisky, J., Bonn, M., and Meister, K.: Electrostatic Interactions Control the Functionality of Bacterial Ice Nucleators, J. Am. Chem. Soc., 142, 6842–6846, https://doi.org/10.1021/jacs.9b13069, 2020.
Lundheim, R.: Physiological and ecological significance of biological ice nucleators, Philos. Trans. R. Soc. Lond. Series B, Biol. Sci., 357, 937–943, https://doi.org/10.1098/rstb.2002.1082, 2002.
Maki, L. R. and Willoughby, K. J.: Bacteria as biogenic sources of freezing nuclei, J. Appl. Meteorol. Climatol., 17, 1049–1053, 1978.
Maki, L. R., Galyan, E. L., Chang-Chien, M. M., and Caldwell, D. R.: Ice Nucleation Induced by Pseudomonas syringae, Appl. Microbiol., 28, 456–459, https://doi.org/10.1128/AM.28.3.456-459.1974, 1974.
Moffett, B. F., Getti, G., Henderson-Begg, S. K., and Hill, T. C. J.: Ubiquity of ice nucleation in lichen – possible atmospheric implications, Lindbergia, 38, 39–43, https://doi.org/10.25227/linbg.01070, 2015.
Murray, B. J., O'sullivan, D., Atkinson, J. D., and Webb, M. E.: Ice nucleation by particles immersed in supercooled cloud droplets, Chem. Soc. Rev., 41, 6519–6554, https://doi.org/10.1039/c2cs35200a, 2012.
Pojar, J. and MacKinnon, A. (Eds.): Plants of the Pacific Northwest Coast: Washington, Oregon, British Columbia, & Alaska, Lone Pine Publishing, Canada, 484–504, ISBN 1-55105-040-4, 1994.
Polen, M., Lawlis, E., and Sullivan, R. C.: The unstable ice nucleation properties of Snomax® bacterial particles, J. Geophys. Res. Atmos., 121, 11666–11678, https://doi.org/10.1002/2016JD025251, 2016.
Pouleur, S., Richard, C., Martin, J., and Antoun, H.: Ice Nucleation Activity in Fusarium acuminatum and Fusarium avenaceum, Appl. Environ. Microbiol., 58, 2960–2964, https://doi.org/10.1128/AEM.58.9.2960-2964.1992, 1992.
Pummer, B. G., Bauer, H., Bernardi, J., Bleicher, S., and Grothe, H.: Suspendable macromolecules are responsible for ice nucleation activity of birch and conifer pollen, Atmos. Chem. Phys., 12, 2541–2550, https://doi.org/10.5194/acp-12-2541-2012, 2012.
Qiu, Y., Hudait, A., and Molinero, V.: How size and aggregation of ice-binding proteins control their ice nucleation efficiency, J. Am. Chem. Soc., 141, 7439–7452, 2019.
Schwidetzky, R., Sudera, P., Backes, A. T., Pöschl, U., Bonn, M., Fröhlich-Nowoisky, J., and Meister, K.: Membranes Are Decisive for Maximum Freezing Efficiency of Bacterial Ice Nucleators, J. Phys. Chem. Lett., 12, 10783–10787, https://doi.org/10.1021/acs.jpclett.1c03118, 2021a.
Schwidetzky, R., Lukas, M., YazdanYar, A., Kunert, A. T., Pöschl, U., Domke, K. F., Fröhlich-Nowoisky, J., Bonn, M., Koop, T., Nagata, Y., and Meister, K.: Specific Ion–Protein Interactions Influence Bacterial Ice Nucleation, Chem.-A Eur. J., 27, 7402–7407, https://doi.org/10.1002/CHEM.202004630, 2021b.
Simpson, J. J., Stuart, M. C., and Daly, C.: A discriminant analysis model of Alaskan biomes based on spatial climatic and environmental data, Arctic, 60, 341–369, 2007.
Turner, M. A., Arellano, F., and Kozloff, L. M.: Three separate classes of bacterial ice nucleation structures, J. Bacteriol., 172, 2521–2526, https://doi.org/10.1128/JB.172.5.2521-2526.1990, 1990.
Vali, G.: Quantitative evaluation of experimental results and the heterogeneous freezing nucleation of supercooled liquids, J. Atmos. Sci., 28, 402–409, 1971.
Wilson, P. W., Heneghan, A. F., and Haymet, A. D. J.: Ice nucleation in nature: supercooling point (SCP) measurements and the role of heterogeneous nucleation, Cryobiology, 46, 88–98, https://doi.org/10.1016/S0011-2240(02)00182-7, 2003.
Womack, A. M., Artaxo, P. E., Ishida, F. Y., Mueller, R. C., Saleska, S. R., Wiedemann, K. T., Bohannan, B. J. M., and Green, J. L.: Characterization of active and total fungal communities in the atmosphere over the Amazon rainforest, Biogeosciences, 12, 6337–6349, https://doi.org/10.5194/bg-12-6337-2015, 2015.
Yang, S., Rojas, M., Coleman, J. J., and Vinatzer, B. A.: Identification of Candidate Ice Nucleation Activity (INA) Genes in Fusarium avenaceum by Combining Phenotypic Characterization with Comparative Genomics and Transcriptomics, Journal of Fungi, 8, 958, https://doi.org/10.3390/JOF8090958/S1, 2022.
Zachariassen, K. E. and Kristiansen, E.: Ice Nucleation and Antinucleation in Nature, Cryobiology, 41, 257–279, https://doi.org/10.1006/CRYO.2000.2289, 2000.