the Creative Commons Attribution 4.0 License.
the Creative Commons Attribution 4.0 License.
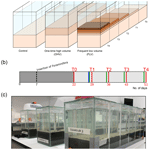
Short-term response of benthic foraminifera to fine-sediment depositional events simulated in microcosm
Corentin Guilhermic
Maria Pia Nardelli
Aurélia Mouret
Damien Le Moigne
Hélène Howa
A microcosm experiment was designed to describe how benthic foraminifera react to fine-sediment deposits varying in frequency and intensity as they may occur regularly or occasionally in coastal benthic environments, caused by discharges from (e.g.) river flooding, tidewater glacier melting in polar regions, or diverse anthropic activities linked to harbour or watershed management. The influence of seabed burial resulting from these events on the ecology of benthic ecosystems is often overlooked, and the resilience of benthic communities is poorly known. During a 51 d long experiment, a typical northeastern Atlantic intertidal foraminiferal community, mainly represented by Ammonia confertitesta and Haynesina germanica species, was subjected to two kinds of sedimentary disturbance: (1) a one-time high-volume (OHV) deposit, i.e. sediment about 3 cm thick was added at one time at the beginning of the experiment; and (2) frequent low-volume (FLV) deposits, i.e. sediment about 0.5 cm thick was added each week for 4 weeks. The geochemical environment (e.g. dissolved oxygen penetration in the sediment, salinity, temperature, and nutrient content in the supernatant water) was monitored to follow the microcosm steady state before and during the experiment. In both disturbed microcosms, H. germanica showed a significant linear decrease in abundance during the experiment, while the total abundance of foraminifera was significantly affected only by the OHV treatment, suggesting a stronger effect of a single thick deposit on standing stocks and biodiversity compared to frequent low-volume sediment supplies. Concerning the vertical migration of foraminifera after sedimentary disturbances, the two dominant species moved upwards to the water–sediment interface with migration speeds estimated to be 0.41 and 0.47 mm h−1 respectively for A. confertitesta and H. germanica. In the FLV treatment, the resilient state was already reached within 1 d following a low-thickness burial, while in the OHV, it was achieved between 1 and 7 d after the 3 cm thick deposit. These results suggest that foraminifera can migrate rapidly after a sedimentary burial to recover their preferential life position under the new sediment–water interface, but in the case of an abrupt thick burial, several days are needed to reach a resilient state.
- Article
(6525 KB) - Full-text XML
- BibTeX
- EndNote
Coastal marine environments are subject to recurrent, erratic, or rare sedimentary depositional events that abruptly bring sediment to the seafloor. Sediment depositional events in coastal marine areas occur under the influence of various drivers such as river flooding (Extence et al., 2013; Dyer, 1988; Hir et al., 2001; Jalón-Rojas et al., 2015), glacier melting in polar regions (D'Angelo et al., 2018; Fossile et al., 2022; Hodson et al., 1998; Meslard et al., 2018), storms (Bolliet et al., 2014; Budillon et al., 2006), or anthropic activities such as dredging (Wolanski and Gibbs, 1992), or land use along catchment basins (Bussi et al., 2016; Kuhnle et al., 1996).
These sediment deposits, when thick and abrupt, can asphyxiate biota and provoke long-lasting destabilization of aquatic benthic ecosystems. In particular, fine-grained-sediment deposition can lead to a decline in microhabitat quality and affect benthic ecosystems in several ways (e.g. Larson and Sundbäck, 2012; Mestdagh et al., 2018; Wood, 1997): (1) by constituting a physical barrier that disrupts the connection to the water column, thereby impeding food supply and oxygen exchange; (2) by altering the substrate's geochemical composition and thus the substrate's suitability for some taxa; and (3) by providing a highly porous, water-saturated substrate whose instability can prevent recolonization from refuge areas.
The question of the impact of sediment supply to benthic realms becomes urgent in the context of the ongoing climate change: among the most impressive consequences for coastal marine environments, there is the disruption of water cycles, including enhanced glacier melting at high latitudes and extreme oscillation of rainfall patterns at lower latitudes, both significantly affecting the sedimentary supply to coastal areas. Excessive deposition of fine sediment is generally recognized to have deleterious effects on aquatic biodiversity and is even considered to be one of the major threats to biodiversity in freshwater environments (Dudgeon, 2019; Mathers et al., 2022, 2022; Sánchez-Bayo and Wyckhuys, 2019) and in marine benthic environments (Alve, 1999; Anschutz et al., 2002). Biota burial and changes in substrate type can delay recovery of crucial benthic ecosystem function. The recovery rate is controlled by a complex combination of ecological and physical forcings (Norkko et al., 2006; Thrush et al., 2006). Among these, the ability of organisms to quickly migrate through the sediment is crucial to the recovery of their preferential habitat at the surface or inside the sediment column. Despite several studies focused on the response of mega- and macrobenthos to physical disturbances (Bolam et al., 2011; Cottrell et al., 2016; Hendrick et al., 2016; Mestdagh et al., 2018), little is known about meio- and microfauna, which represent lower steps of the trophic chain and therefore have the potential to control the ecosystem functioning through a bottom-up relationship.
Benthic foraminifera (Eukaryotes, Rhizaria) are unicellular organisms belonging to meiofauna and are highly sensitive to sedimentary and geochemical changes in their environment (e.g. Murray, 2006) and present several characteristics that make them powerful bio-indicators of marine environmental characteristics (Schönfeld et al., 2012): (i) high density in marine sediments; (ii) short lifecycles; (iii) occupation of specific ecological niches and microhabitats, including superficial, shallow, and deep infaunal sediment layers (up to 10–20 cm depth). Because of these characteristics, foraminifera have increasingly been used as biotic tools for assessing the quality status of coastal marine environments (Alve et al., 2016; Barras et al., 2014; Belart et al., 2018; Bouchet et al., 2018a, 2012; Fontanier et al., 2009, 2020; Frontalini and Coccioni, 2008; Jorissen et al., 2022; Laut et al., 2021; Martins et al., 2013, 2015, 2016; Murray, 2006; Nesbitt et al., 2015). Moreover, Bouchet et al. (2018b) showed that benthic foraminifera can be better bio-indicators than macrofauna as they can be present on a larger spectrum of environmental gradients compared to macrofauna and are generally more sensitive to (and therefore absent in) highly stressed conditions. In Arctic fjords, foraminiferal ecological response to environmental stress has been observed to mirror the ones of macrofauna, with decreasing diversity and a dominance of opportunistic taxa (Wlodarska-Kowalczuk et al., 2013). In natural marine environments, vertical and horizontal distributions of benthic foraminiferal faunas are controlled by several parameters, noticeably organic matter and oxygen content in their habitats (e.g. Contreras-Rosales et al., 2012; Goineau et al., 2012; Gooday et al., 2000; Jorissen et al., 1995; Langezaal et al., 2006; Schumacher et al., 2007). Following the conceptual model from Jorissen et al. (1995) taken over by Van der Zwaan et al. (1999) and Koho et al. (2015), foraminiferal vertical distribution is limited in eutrophic systems by oxygen concentration in bottom and sediment porewaters and by organic matter availability in the oligotrophic realm. Beyond these two geochemical drivers, a third factor seems to affect the benthic environment, namely the physical forcing by sediment supply to the bottom. Some recent studies of naturally stressed coastal environments focused on the response of foraminiferal communities to excessive fine-sediment supply due to natural processes. Various environments were prospected: turbidites in canyon channels and terraces (Bolliet et al., 2014; Dessandier et al., 2016; Duros et al., 2017; Goineau et al., 2012; Hess and Jorissen, 2009), prodelta river flooding (Goineau et al., 2012), and river-dominated shelves (Dessandier et al., 2016). Other studies concentrated on anthropic activities that directly cause massive fine-sediment supply in coastal areas and the associated effects on benthic foraminifera faunas (e.g. oil drill cutting disposal – Mojtahid et al., 2006; exacerbated land use – Fontanier et al., 2018; industrial waste – Fontanier et al., 2020). Most of these studies mainly focus on massive and sudden or occasional deposits of sediment, and the fact that they are performed in natural environments represents a limit for the interpretations. Indeed, in natural settings, sediment supply, organic matter input, and oxygen availability often covary and synergically affect benthic communities and microhabitat distribution. Experimental studies are therefore the only way to test the effect of a single parameter in a controlled setting where environmental variability can be artificially reduced. For these reasons, we designed an experiment to test, in microcosms, the effect of different patterns of fine-grained-sediment deposits on benthic foraminiferal communities without varying organic matter content and oxygen availability. Two different modes of sediment input were selected to characterize the vertical migration and survival of foraminifera under the pressure of various physical disturbances, namely a single, thick sediment deposit and thin and recurrent sediment deposits, in order to test whether or not the ecological response is affected by the amplitude and the frequency of the sedimentary disturbance. The ecological responses we observed concerned density and diversity variations at different time intervals and their vertical distribution (representing their migration ability) after the two disturbances' regimes.
Our experimental design was not intended to exactly reproduce a natural environment but rather to control a single ecological driver, i.e. the fine-grained-sediment supply.
2.1 Biological model
In our experiment, we used benthic foraminifera species that inhabit the mudflats of the French Atlantic coast. Foraminifera samples were collected at low tide, in the upper mudflat of the Bay of Bourgneuf called La Couplasse, a vast maritime bay enclosed by the island of Noirmoutier. The assemblages were largely dominated by two species, Ammonia confertitesta Zheng, 1978 (Hayward et al., 2021; often reported as Ammonia tepida in the literature) and Haynesina germanica (Ehrenberg, 1840). These two species live in similar shallow infaunal microhabitats, i.e. near the sediment–water interface on tidal mudflats at temperate latitudes. They are often associated and dominant in such natural coastal environments and are not expected to be in exclusive competition (Alve, 2001; Morvan et al., 2006; Murray and Alve, 2000; Thibault de Chanvalon et al., 2015).
The species Ammonia confertitesta has already been used in microcosms and cultured in investigations focusing on growth and calcification processes (Bradshaw, 1957; Denoyelle et al., 2012; Geslin et al., 2014; Nardelli et al., 2014; Stouff et al., 1999), the effects of contaminants (Denoyelle et al., 2012; Le Cadre and Debenay, 2006; Suokhrie et al., 2017), or metabolical responses to stressed environments (Geslin et al., 2014; Heinz and Geslin, 2012; Jauffrais et al., 2016a; Koho et al., 2018; Nardelli et al., 2014). Therefore, this species was chosen here for its high ability to withstand experimental living conditions for long-lasting periods of time (up to several months). The second species, Haynesina germanica, has also been studied in experimental conditions for its ability to sequester chloroplasts and perform photosynthesis (Jauffrais et al., 2016b) or for its metabolic responses to stressed environments (Deldicq et al., 2021; Langlet, 2020; Seuront and Bouchet, 2015). However, previous experiments involving H. germanica only lasted several days. Although both species were never used in microcosms testing sediment input, we expected them to respond to sediment depositional events that would directly disturb the stability of their shallow infaunal microhabitat.
2.2 Experimental design
Two scenarios were implemented in two different aquaria to simulate simultaneously (1) a one-time high-volume (OHV) scenario in which the microcosm received one single sedimentary load, resulting in a thick deposit; and (2) a frequent low-volume (FLV) scenario with four successive (1-week period each) small supplies, each burying the microcosm under a thin sediment layer. In parallel, a control microcosm in a third aquarium received no sediment input during the experiment (Fig. 1).
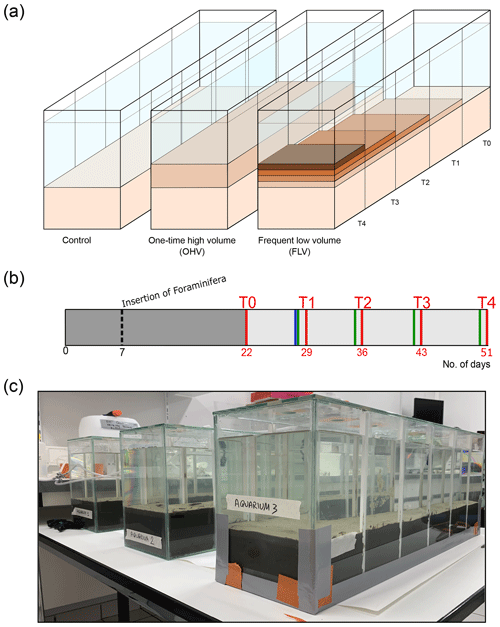
Figure 1(a) The three aquaria correspond to the control and the two sediment deposit modes. Successive deposit layers are symbolized by darker colours. The sampling times (T0 to T4) are mentioned at the level of the associated compartment which was sampled at that time and are also linked to (b) the timeline showing sediment inputs (blue bar for OHV and green bars for FLV) and core sampling times (D+2 after disturbances, red bars) as a function of the number of days of the experiment, starting from the introduction of the sediment into the aquaria (day 0). The insertion of foraminifera occurred on day 7, and the dark-grey area represents the period (22 d long) for geochemical and foraminifera equilibration. (c) Picture of the experimental set-up after the sediment substrate addition on day 5.
The three glass aquaria ( cm; 750 cm2 surface area) were designed to allow five consecutive samplings at 1-week intervals without disturbing the rest of the microcosm. For this purpose, in each aquarium, five compartments (10 cm long; 150 cm2 surface area) can be successively isolated from the rest of the microcosm by inserting plexiglass plates into four pairs of small gutters attached to the aquarium walls (Fig. 1c). At each consecutive sampling time, sediment samples and geochemical measurements were collected from the newly isolated compartment. To limit evaporation, the three aquaria were covered with a large glass plate with a hole above each compartment, allowing the introduction of a continuous bubbling system to maintain good oxygenation and mixing of the water in the aquaria.
2.3 Experimental preparation
Natural coastal seawater, with a salinity of 33 and very low turbidity, was collected and microfiltered using paper filters with a mesh size of 0.45 µm before filling a 100 L water tank. This filtration ensured the removal of organic or mineral detritus and of macro-, meso-, and micro-organisms that might have interfered with the microcosms. A closed water circuit equipped with a pump was installed to initially fill the aquaria from the water tank. On day 35, after a breakdown of the pumping system, it was decided to manually renew the water in the aquaria by replacing it completely at each sampling time and at about two-thirds of the volume twice a week with water from the tank.
The sediment used to constitute the initial sediment (Fig. 1, light beige), was collected at low tide on the Couplasse mudflat (Bourgneuf Bay – 47∘0′57′′ N, 2∘1′29′′ W) on 13 January 2021 and was stored in sealed plastic bags at −20 ∘C until the experiment was set up. The purpose of this freezing step was to preserve in situ organic matter content and freshness and to kill present meiofauna or macrofauna that might be living in this sediment and might interfere with further treatments. In this way, we were also sure that this sediment substrate was free of the in situ foraminiferal community. Grain size analysis on sediment aliquots performed using a laser diffraction particle analyser, Malvern Mastersizer 3000, revealed a unimodal distribution (mode 6 µm), with a D50 of 10 µm and a D90 of 47 µm. The proportions of silt and clay were 93 % and 7 % respectively. The material used to simulate sedimentary disturbance was prepared as follows: the sediment collected at La Couplasse was unfrozen and diluted with the microfiltered seawater in order to obtain a highly turbid solution. This dense solution was slowly introduced into the water column of the aquaria via a small-diameter plastic tube. The particles settled down on the prior sediment surface. To seed the microcosms in a controlled manner, living foraminifera were collected on 16 February 2021 at low tide at the same location as for the sediments, i.e. the Couplasse mudflat. Surface sediment was sieved in situ to recover the 125–500 µm size fraction. This size fraction included foraminifera and possibly meiofauna or juveniles of macrofauna and some organic matter detritus. Samples were conditioned in 500 mL plastic bottles with one-fifth sediment and four-fifths in situ seawater. Then, the samples were stored in the temperature-controlled room (at 14 ∘C) where the experiment was conducted and were air-bubbled until insertion into the microcosms.
On day 0 (16 February 2021; Fig. 1b), a layer of approximately 9 cm thick was placed on the bottom of each aquarium, carefully avoiding the formation of internal voids and ensuring a flat sediment surface. The required amount of sediment was thawed and homogenized just before filling the aquaria. After a few hours, the necessary time for the settling of the fine particles, filtered seawater was gently introduced to fill the aquaria with a 10 cm high water column, avoiding any disturbance at the water–sediment interface. The three aquaria, kept oxygenated by the air-bubbling system, were left to stand for 7 d prior the insertion of foraminifera to allow for sediment compaction and initial equilibration of the redox fronts.
On day 7 (23 February 2021; Fig. 1b), the sediment was seeded with living foraminifera; the major challenge was to obtain a spatial distribution of living specimens that was as homogeneous as possible over the entire sediment surface in each aquarium. Each microcosm was divided into 40 rectangles (5×3.75 cm). For this purpose, foraminiferal samples were mixed and then split into 5 mL sub-samples. The 5 mL aliquots were carefully inserted with a small syringe into each rectangle of a grid placed just above the sediment–water interface and immediately removed after insertion of the foraminifera. Then, a 15 d rest period was observed before the first sampling (T0) to let the individuals reach their preferential microhabitats in the sediment.
2.4 Experimental procedure
The sampling period began on day 22 (Fig. 1b) after filling the aquaria with sediment and water on day 0 and inserting the foraminifera on day 7 (Fig. 1b). Five successive samplings (T0 to T4) were done every week, each in one compartment of each microcosm (Fig. 1a). On day 22, a first sample (T0) was taken from the first compartment of the three microcosms before the application of any disturbance. After sampling, the compartment was closed using plexiglas plates carefully inserted into gutters placed on the side of the microcosms (vertical white lines on the glass; Fig. 1c) and was drained of its water. The water in the remaining part of the aquaria was renewed the next day with water from the 100 L tank. In the control microcosm (left aquarium in Fig. 1a), the next four samplings (T1 to T4) were done in successive compartments of the aquarium that were not subjected to any sedimentary disturbance throughout the whole experiment. In the one-time high-volume microcosm (middle aquarium in Fig. 1a), a 2.7 cm thick (after definitive particle settling) sediment layer was added at once on the day before sampling T1 (day 29, blue bar Fig. 1b). Afterwards, samplings T2 to T4 were done in successive compartments of the aquarium without further addition of sediment. After each sampling, the compartment was closed and emptied. In the frequent low-volume microcosm, a smaller amount of sediment was added each week (day 28, day 35, day 42, day 50; green bars in Fig. 1b) to stack layers of approximatively 0.3–0.5 cm thickness each. Samplings T1 to T4 were done in successive compartments of the aquarium on the day following each sediment addition. Therefore, T4 sampled a sedimentary column containing the four successive 0.3–0.5 cm layers in the last compartment.
2.5 Control of the stability of the microcosms
To monitor the stability of the microcosms, salinity and temperature measurements were performed daily with a WTW® Multi 3620 probe (measurement resolution of 0.1 and 0.1 ∘C for salinity and temperature respectively). Air-bubbling ensured a good oxygenation and mixing of water, thus preventing water stratification. A lateral view of each aquarium was photographed daily using a Nikon D3400 camera to monitor visual changes in the sediment column (e.g. colour, compaction, and bioturbation).
The effect of sediment disturbance as a physical cover of the sediment surface was followed by dissolved-oxygen profiling in the sediment, giving the oxygen penetration depth (OPD). However, no measurements were available at T3 due to experimental failure. Measurements were done the day after each sampling time (i.e. 2 d after the sedimentary disturbance), using 50 µm tip diameter Clark-type Unisense™, microelectrodes mounted on an automated micro-manipulator (Revsbech, 1989) taking measurements with a 50 µm vertical step. Significant differences among sampling times and/or microcosms were tested by ANOVA and Tukey post hoc tests to investigate further and more detailed relations; these tests were performed using R software.
Additionally, nutrient content (NH, NO, and NO in the water column was monitored and displayed as total inorganic nitrogen (TIN). Indeed, fluxes from the sediment column resulting from the degradation of organic matter can lead to very high accumulations of inorganic N in the water column, which can result in the alteration of geochemical equilibria in the sediment (Hansen and Blackburn, 1992; Kristensen and Blackburn, 1987; Silverberg et al., 1995). A total of 5 mL of water was collected at least every 3 d, filtered (0.2 µm, RC25, Sartorius ©), and stored at −20 ∘C. The concentrations of all nutrients were measured using a spectrophotometric analyser (GENESYS 20, Thermo Fisher ©). Ammonium (NH concentrations were analysed using the Berthelot method adapted for small samples and seawater samples (Metzger et al., 2019). Nitrite concentrations were measured by a colorimetric reaction with the Griess reagent (Griess, 1879). The analysis of nitrate is the second step in the sequential determination described in García-Robledo et al. (2014) and involves the use of vanadium chloride (VCl3) to reduce nitrate into nitrite. Nitrate concentrations [NO] can therefore be calculated from the measured NO + NO using the following relation (García-Robledo et al., 2014):
where Abs is the final measured absorbance, i.e. combination of [NO] and [NO]; Abs is the absorbance of VCl3 without [NO] or [NO]; and are the slope of calibration curves after VCl3 addition; and [NO] is the previously calculated concentration of nitrite in the sample.
2.6 Experimental sediment sampling procedure
At each sampling event (18 h after the physical disturbance), one compartment of the aquarium was physically separated from the rest of the aquarium; the overlying water was carefully pumped out to limit sediment resuspension; and four cores (2.9 cm internal diameter, ∼8.5 cm long) were collected using adapted syringes, acting as miniature disposable piston corers. Two cores were used for foraminiferal analyses (including one replicate): one was for porosity analysis (data not shown in this paper), and one was resin embedded for further geochemical analyses (data not shown in this paper). Foraminiferal cores were immediately sliced using inox spatulas every 0.2 cm down to 4 cm depth, then every 0.5 cm from 4 to 7 cm depth. During this process, no shell debris was observed. A specifically designed push core with a screw resolution of 1 mm per turn allowed accurate sediment extrusion.
For living foraminifera analyses, sediment slices were labelled with CellTracker Green (CTG). CTG is a dye which is hydrolysed during metabolization by living individuals, resulting in a fluorescent-green staining of the cytoplasm already in use for foraminiferal labelling (Bernhard and Bowser, 1996; Bernhard et al., 2006; Choquel et al., 2021; Geslin et al., 2014; Nardelli et al., 2014; Pucci et al., 2009; Richirt et al., 2020; Ross and Hallock, 2018). This CTG label therefore identifies foraminifera with an active metabolism and is highly reliable for detecting short temporal foraminiferal responses to disturbances. Following Bernhard and Bowser (1996) and Bernhard et al. (2006), samples for foraminiferal analyses were incubated at experiment temperature (14 ∘C) in a CTG solution (CellTracker™ Green, 1 mM final concentration) in microfiltered seawater for 24 h. After incubation, the solution was fixed in 70 % ethanol and sieved over 125 µm mesh screens (corresponding to the minimal size of the foraminifera introduced in the experiment). The counting process of living individuals was performed under epifluorescence stereomicroscopy (i.e. 470 nm excitation; Olympus SZX13). Only specimens presenting a clear and continuous fluorescence were picked and counted at the species level. Total foraminiferal abundances (per core) were calculated, taking into account the counting of all individuals living in the whole sediment column of 7 cm depth with a section of 6.60 cm2, and were expressed as the number of individuals per 10 cm2 (ind. 10 cm−2), being the sum of individuals counted in each core slice. Foraminiferal densities per core slice were expressed as individuals per 10 cm3 (ind. 10 cm−3).
Additionally, one core from the second sampling time of the one-time high-volume microcosm (OHV T1) was selected to test for an eventual correlation between vertical-migration rate and foraminiferal test size. Following the procedure of Richirt et al. (2020), high-resolution pictures (6016×4016 pixels) of the entire assemblage picked in each core slice were taken using a camera (Nikon™ D750) set on a stereomicroscope. Each specimen of the investigated assemblage was placed on its ventral or dorsal side to obtain a picture of the maximal test length. Images were processed using ImageJ software (Schneider, 2012), with which the maximum diameter of each isolated individual was measured, and the specimen area was calculated in µm2 (Richirt et al., 2020). In our study, data are presented by species and on a vertical scale corresponding to all slices of the investigated core (OHV T1). Statistical analysis was performed using R software. Univariate ANOVA tests were performed to compare the size of individuals in all core slices. Tukey post hoc test was carried out when the ANOVA was significant. Displacement speeds were estimated on the same core (OHV T1). To do so, we measured the vertical distance between the initial water–sediment surface and the level within the newly deposited sediment reached by living foraminifera. This distance was therefore travelled upwards between the time of sediment addition and the sediment sampling time (i.e. 18 h). The maximum speeds (mm h−1) were calculated by species using the maximum vertical distance travelled by individuals of the two species Ammonia confertitesta and Haynesina germanica. The accuracy of the distance measurement is 0.2 cm (core slice thickness). The mean speeds (mm h−1) were calculated by species based on the vertical distance travelled above the initial water–sediment interface, weighted by the number of living individuals found at this level.
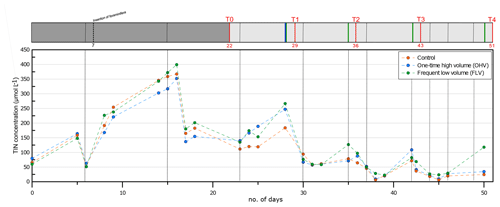
Figure 2Total inorganic nitrogen concentration in the water column (, , and ) in the three aquaria throughout the experiment. Vertical dotted black lines indicate water renewals (after each sampling time and more frequently after the pump breakdown at day 35). The header displays the timeline explained in Fig. 1b.
3.1 Geochemical stability of the microcosms
Temperature and salinity were kept constant during the whole experiment in the three microcosms (salinity 32.9±0.50; T14.7±0.18 ∘C). The monitoring of TIN concentrations in the water column throughout the whole experiment is presented in Fig. 2. From the filling of the aquaria (day 0) until day 15, a strong addition of 340 µmol L−1 of TIN was observed, with the concentration increasing from 60 µmol L−1 to the maximum of 400 µmol L−1. This peak of approximately 3 d (day 14 to day 16) was immediately followed (day 17) by an abrupt strong decrease to a concentration of about 160 µmol L−1. TIN then showed a progressive decrease with oscillations of about 100 µmol L−1 in amplitude, with the maximum of these oscillations occurring just before water renewal. From T1 until T4, the concentration remained below 100 µmol L−1, except for relative peaks of about 110–150 µmol L−1 observed just after each sediment disturbance. Variations in TIN concentrations were relatively synchronized in all three aquaria, except just after the sediment disturbance at T4 in the FLV microcosm.
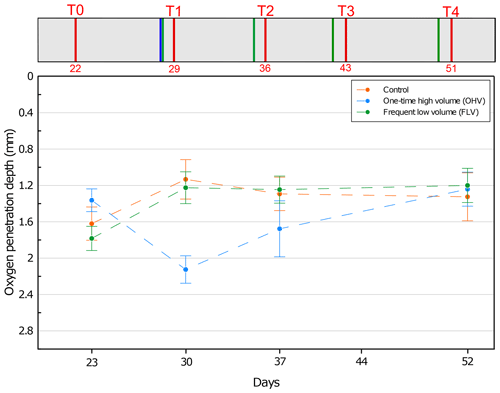
Figure 3Mean oxygen penetration depth with associated standard deviation for each microcosm and at each sampling time. No data available for T3. On the y axis, 0 at the top represents the sediment–water interface. The header displays the timeline explained in Fig. 1b.
At the first sampling time T0, OPD varied between 1.3 and 1.8 mm in the three aquaria (Fig. 3). This variation has a lower range than the 2 mm resolution (size of the upper core slices) used in our foraminifera analysis. From T0 to T1, in both the control and FLV microcosms, oxygen penetration showed a significant shallowing (p values < 0.05) to a depth of 1.2±0.2 mm and then remained stable until T4. At T1 and within the OHV microcosm, OPD deepened to 2.1±0.1 mm after the massive deposit. After T1 and until the end of the experiment, oxygen penetration presented a shallowing trend, reaching the same depth as in the other two microcosms at T4 (1.3±0.3 mm for the control microcosm, 1.2±0.2 mm for the OHV microcosm, and 1.2±0.2 mm for the FLV microcosm).
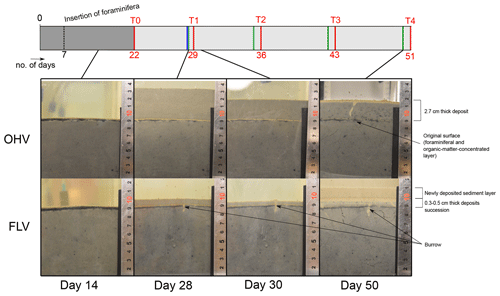
Figure 4Lateral views of the one-time high-volume (OHV) and frequent low-volume (FLV) sedimentary disturbances at four different times during the experiment. The header displays the timeline explained in Fig. 1b.
Lateral views of the OHV and FLV aquaria show the sedimentary column at four different moments of the experiment (Fig. 4), allowing us to track sediment compaction and colour changes during and after the sedimentary deposits. At day 14 (before any disturbance), the sediment column (i.e. the substratum of the experiment) was homogeneous in the three aquaria. It was already compacted, and no more fine sediment was visible in suspension in the overlying water column. A few millimetric black spots, scattered within the sediment matrix, were most likely microniches of organic matter anaerobic remineralization (Jørgensen, 1977; Lehto et al., 2017; Widerlund et al., 2012). At 9 cm height in the aquaria, the initial water–sediment interface was clearly visible as a doublet of yellowish and black millimetric layers (2–3 mm), constituted by the material (foraminifera and associated particulate organic matter) introduced on day 7. The upper yellowish layer corresponded to the well-oxygenated layer of this material. Its thickness was consistent with measured OPD (Fig. 3). The underlying black layer corresponded to the anaerobic degradation of the introduced organic matter.
On day 28, the first sediment addition occurred in two aquaria. A thick layer (about 4.3 cm) of beige sediment in the OHV microcosm and a thin layer (about 1 cm) in the FLV one were deposited above the former water—sediment interface that was still very clearly visible. On day 30, the sediment layer thickness in both aquaria was already reduced to 2.7 cm in the OHV microcosm and to 0.5 cm in the FLV one. This rapid compaction of about one-third of the newly deposited sediment occurred within 2 d. In both aquaria, the first T1 deposit was well marked between the initial surface (yellow–black doublet) and a very thin (<1 mm) yellowish layer at the new water–sediment interface. This light colour underlined the good oxygenation of the superficial sediment less than 2 d after the deposit.
On day 50, in the FLV microcosm, it was possible to detect the four successive supplies of sediment by observing the layering of yellow–black doublets in the final 2 cm thick layer. As a final important observation, we noticed the rare development of small vertical burrows (Ø<1 mm, a few centimetres long) in all three aquaria. In our experiment, the bioturbation was limited by the freezing of the sediment used to fill the aquaria and the initial sieving (<500 µm) of the biological material introduced on day 7.
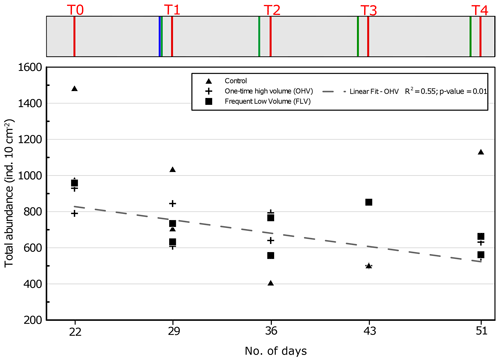
Figure 5Total foraminiferal abundances (>125 µm) per core sampled in each microcosm at each sampling time. The displayed values are the abundances of two replicate cores (n=1 for control T3, FLV T0, and FLV T3). The regression line is shown for OHV with R2 and the associated p value (other regression lines are not drawn because they are not significant). Days from the start of the experiment are indicated on the x axis. The header displays the timeline explained in Fig. 1b.
3.2 Effects of sedimentary disturbances on total foraminiferal abundances
Variations in total foraminiferal abundances were analysed during the experiment for the three aquaria. At T0, before any sedimentary disturbance, the total foraminiferal abundances varied in the three aquaria between 790 and 1483 ind. 10 cm−2 (Fig. 5). In the OHV microcosm, a linear regression demonstrates a significant (R2=0.55; p value = 0.01) decreasing trend in foraminiferal abundances over time, with an average loss of about 300 ind. 10 cm−2 (863±73 ind. 10 cm−2 at T0 and 582±31 ind. 10 cm−2 at T4).
There is no such significant trend in foraminiferal abundances with time, neither in the control microcosm (R2=0.15; p value = 0.29) nor in the FLV microcosm (R2=0.23; p value = 0.22). In the case of the control microcosm, the high variability between replicates was at its maximum at T4, ranging from 650 to 1100 ind. 10 cm−2.
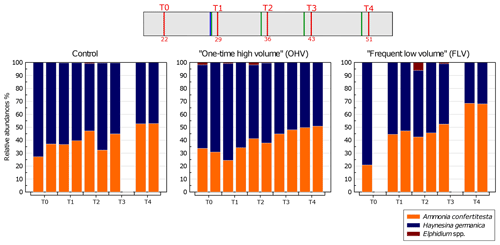
Figure 6Relative abundances (%) of each species (>125 µm) per core – with replicates – sampled in each microcosm at each sampling time. The displayed values are the relative abundances of two replicate cores (n=1 for control T3, FLV T0, and FLV T3). The header displays the timeline explained in Fig. 1b. Note the absence of a second replicate in the control T3 and FLV T0 and T3 due to sampling failures.
3.3 Effects of sedimentary disturbances on assemblage composition
Variations in relative species abundances per core were analysed for the three microcosms over the course of the experiment (Fig. 6). The foraminiferal assemblage used in this experiment was mainly composed of Ammonia confertitesta and Haynesina germanica. At T0, in all the aquaria, H. germanica was dominant, accounting for 63 % to 79 % of the assemblage. Thereafter, the abundances of A. confertitesta and H. germanica balanced out to become equally distributed at T3. At T4, A. confertitesta exceeded 50 % in all aquaria and became particularly dominant in the FLV microcosm, where it accounted for 68 % of the assemblage. Relative abundances showed a clear shift from an initial domination of H. germanica over A. confertitesta to a more balanced assemblage. A few specimens of Elphidium spp., another species known to live in low abundances in the upper mudflat of Bourgneuf Bay in winter (Choquel, 2021), were occasionally found in the sediment samples of the three aquaria. They represented, at maximum, 6 % of the total assemblage (31 individuals counted out of 506 individuals) in only one core (FLV T2 replicate) but were mostly absent from the other cores or were present at less than 2 %.
Variations in the abundances of A. confertitesta and H. germanica per core analysed for the duration of the experiment in the three aquaria were therefore examined more specifically (Fig. 7). In the control microcosm, the total abundances of A. confertitesta and especially H. germanica were very variable between replicates throughout the experiment.
Concerning Ammonia confertitesta, abundances in the OHV microcosm did not show any significant trend in time and were found in the narrow range of 220–300 ind. 10 cm−2, except at T1 just after the single thick sedimentary disturbance, when abundances dropped to 150 ind. 10 cm−2. In the FLV microcosm, a significant increasing trend occurred (p value < 0.05), doubling total abundances from T0 to T3, and then abundances remained stable between T3 and T4. However, the lack of a second replicate at T0 did not provide information on the initial variability. Concerning H. germanica, total abundances significantly (p value < 0.05) decreased throughout the experiment, from ∼600 to ∼300 ind. 10 cm−2 in the OHV and from ∼750 to ∼200 ind. 10 cm−2 in the FLV. In the control microcosm, abundances decreased from 1100 ind. 10 cm−2 at T0 to 300 ind. 10 cm−2 at T4. The high variability between replicates, particularly at T0 and T4, partially concealed the decreasing trend and resulted in a relatively bad correlation, with an R2 of 0.37 and a p value of 0.08.
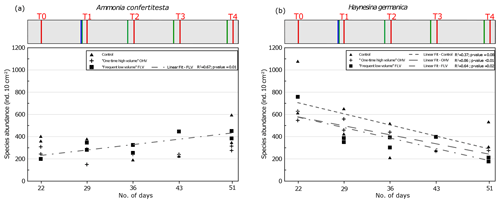
Figure 7Foraminiferal abundances of the two main species in sampled replicates, (a) Ammonia confertitesta and (b) Haynesina germanica, at each sampling time. Replicates are missing at FLV T0 and T3 and control T3. The regression line is shown for FLV with R2 and associated p value (other regression lines are not drawn because they are not significant). The header displays the timeline explained in Fig. 1b.
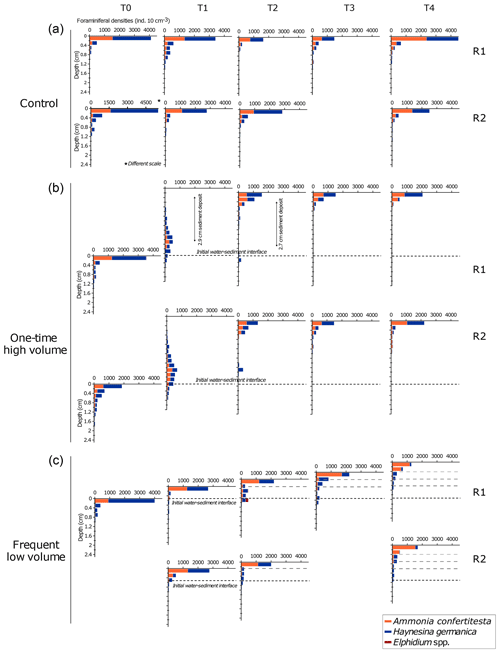
Figure 8Vertical distribution of specific densities of living foraminifera in replicates at each sampling time displayed for each microcosm. The water–sediment interface of each plot is aligned with the previous one to illustrate the added sediment layers in the OHV and the FLV microcosms. The scale of the x axis of the control T0 replicate 2 is different to the others. Note the absence of a second replicate in the control T3 and FLV T0 and T3 due to sampling failures.
3.4 Effects of sedimentary disturbances on vertical distributions
In the control microcosm (Fig. 8a), vertical distributions of both species, Ammonia confertitesta and Haynesina germanica, showed the highest densities of individuals in the uppermost 0.2 cm of sediment throughout the whole experiment. The uppermost 0.2 cm layer contained between 58 % and 81 % of the total assemblage found in the 7 cm sediment column. For both species, a similar exponential decrease with depth occurred down to 0.8 to 1.4 cm. Below this depth, no living individuals were found. Concerning the OHV treatment, a vertical profile similar to the one of the control microcosm occurred at T0, with maximum densities in the uppermost 0.2 cm and an exponential decreasing profile with depth down to about 2 cm depth (Fig. 8b). At T1, 18 h after the addition of about 2.9 cm of sediment (before full compaction) above the initial water–sediment interface (dotted line in Fig. 8b), the foraminiferal vertical distribution displayed unimodal profiles with modes or maximum densities situated 2.3 cm below the new surface or 0.6 mm above the initial water–sediment interface. Densities then showed quite a symmetrical decrease up and down the density peak. Approximately 71 % of the fauna was found between 2 and 3 cm depth, where the specific composition of the assemblage was equally represented by A. confertitesta and H. germanica. No living foraminifera were detected above 0.2 cm depth and below 3.4–3.6 cm depth in both replicates. The few individuals that reached the upper sediment layers (from 2.2 to 0.8 cm depth) and those that remained at depth below the mode were identified as belonging to the species H. germanica. At T2, after full compaction, giving a total sediment height of 2.7 cm above the initial water–sediment interface (Fig. 4), the assemblages had shifted toward the new surface to concentrate in the upper layers of the sediment column (0 to 1.2 cm depth maximum below the new sediment surface). Vertical profiles showed exponential decreasing with depth. Only a few specimens, belonging exclusively to H. germanica, were found in layers deeper than 1.2 cm (Fig. 8b). This distribution remained quite similar in the successive sampling times T3 and T4, with a slight increase (30 %) of A. confertitesta in the topmost layer (0–0.2 cm) and a decrease in the deeper layers (below 0.4 cm depth). In Fig. 8c, assemblage profiles in the frequent low-volume (FLV) microcosm are drawn to be shifted upwards from the initial water–sediment interface. The distance between the new and former interfaces illustrates the thickness of the sediment supplied before each sampling time. On the day before each sampling time (T1 to T4), successive 0.3–0.5 cm thick sediment deposits were added, and thus the ancient surface (dotted black line in Fig. 8c) was further buried. At T0, assemblages displayed a similar vertical distribution profile to the other microcosms (Fig. 8c). However, the assemblage was not balanced. Ammonia confertitesta was only present above 0.2 cm depth, with ∼900 ind. 10 cm−3, whereas H. germanica was present up to 1.2 cm depth and was 75 % dominant in the surface layer, with ∼3100 ind. 10 cm−3. At T1, the vertical distribution of foraminifera in both replicates was back to the original profile of T0, with a maximum foraminiferal density above 0.4 cm depth and no specimens below 1.2 cm depth. However, the assemblages showed a decrease in the relative density of H. germanica compared to T0 (70 % in the upper layer). At T2 and T3, most specimens were still concentrated in the uppermost 0.2 cm, with about 2000 ind. 10 cm−3. Below the 0–0.2 cm level down to the initial water–sediment interface (0.8 cm depth at T2 and 1.1 cm depth at T3), the vertical distribution displayed persistently low densities of less than 100 ind. 10 cm−3 and 100–300 ind. 10 cm−3 for T2 and T3 respectively. From T1 to T4, H. germanica densities decreased in the uppermost layer in favour of A. confertitesta, while these remained dominant in the deeper layers (Fig. 8c). At T4, A. confertitesta largely dominated the 0 to 0.4 cm depth layers, with about 1900 ind. 10 cm−3 versus 200 ind. 10 cm−3 for H. germanica. Below 0.4 cm depth, lower densities (∼140 to 240 ind. 10 cm−3) of A. confertitesta were observed, whereas H. germanica appeared to be more abundant below 0.4 cm depth (∼660 to 860 ind. 10 cm−3).
3.5 Foraminiferal migration: relationship with test sizes and specific speed
To evaluate the migration speed of each species, data from the OHV core T1 replicate 1 (T1 R1) were used. This core was collected at T1 18 h after the disturbance that buried the initial water–sediment interface under a thick sediment layer (2.9 cm after compaction, Fig. 4). The foraminifera spread in the added sediment layer displayed a unimodal vertical distribution, where the density peak was located in the sediment slice 2.2–2.4 cm depth below the new surface and thus at 0.5–0.7 cm above the initial interface. Compared to the distribution profile displayed before the disturbance (T0), we observed an upward migration of both Ammonia confertitesta and Haynesina germanica, with a maximum vertical distance covering 2.6 cm at the time of sampling (Fig. 8b). Some individuals of the two species did not migrate at all as they were still present below the initial interface (6 % of total A. confertitesta individuals versus 14 % for H. germanica). The weighted mean speed was different between the two species (0.41 mm h−1 for A. confertitesta and 0.47 mm h−1 for H. germanica).
Based on the results of the T1 R1 core from the OHV microcosm, we investigated the correlation between the individuals' test sizes of each species and their location in the sediment column to find an eventual relationship between size and migration speed. To do so, a morphometric analysis was performed on the test of each specimen found at each sediment layer. The vertical distribution of the individual test area (mm2), mean values, and standard deviations are shown by species in Fig. 9. The results showed a very high heterogeneity of test areas for Ammonia confertitesta, with values spreading from 0.04 to 0.2 mm2 around median values per slice of approximately 0.1 mm2. The statistical test did not reveal significant differences (ANOVA, p value = 0.119) in A. confertitesta test size between the different sediment slices. For H. germanica, however, statistically significant differences were found between sediment slices (ANOVA, p value = . However, the Tukey post hoc test highlighted significant differences between the 0.8 to 1.2 cm depth interval and the two similar depth intervals of 2–2.6 and 2.8–3.0 cm. Above 0.8 cm depth, the size of H. germanica specimens did not show significant differences compared to the other levels.
4.1 Geochemical and physical stability of the experimental system
Parameters like temperature, salinity, and TIN in overlying water and O2 penetration in the sediment were monitored throughout the experiment in order to control the geochemical stability of the microcosms. While water in the microcosms was often renewed, temperature and salinity remained constant, whereas TIN concentrations and OPD demonstrated that the geochemical stability of the microcosm was difficult to reach.
In the first part of the experiment (before day 14; Fig. 2), the high TIN concentrations could be attributed to the seeding of the microcosm, including, along with living foraminifera, high quantities of phytodetritus, meiofauna, and fecal pellets (<500 µm). This organic matter supply was concentrated within a 0.3 cm layer at the sediment surface (organic-matter-concentrated layer; Fig. 4). The mineralization of this organic matter is an additional source of TIN in the overlying water of the microcosms. After T0, recurrent increases of TIN underlined by sharp peaks (days 16, 28, 35, and 42) occurred from the water renewals until the following sampling (Fig. 2). This testified to continuous fluxes of TIN released from the sediment to overlying waters, causing an increase of TIN concentration in the water column interrupted by water renewals in the aquaria. The peak amplitudes were gradually diminished due to the progress of organic matter mineralization and impoverishment of the system.
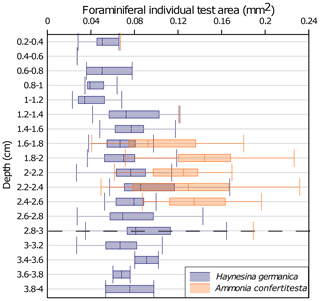
Figure 9Vertical distribution of benthic foraminiferal test size in the one-time high-volume microcosm, core T1 R1. Values are shown as box plots (median, 25th and 75th quartiles) The depth is expressed by sediment slices. The dotted line (at the 2.8–3 level) symbolizes the initial water–sediment interface before the sedimentary disturbance.
Even if the geochemical state of TIN in the microcosm was not perfectly stable, the regular renewals of seawater prevented excessive accumulation of organic matter degradation products in the overlying waters and sediment and were sufficiently effective to maintain TIN concentrations at a lower concentration range than that observed in the in situ sediment of Bourgneuf Bay (Metzger et al., 2019).
The aerobic degradation of the organic matter added with the introduction of foraminifera on day 7 was probably responsible for the shallowing of the oxygen penetration depth observed between T0 and T1 in the control and frequent low-volume (FLV) microcosms. From the introduction of foraminifera, the OPD stabilization in both microcosms was reached after 22–29 d (i.e. between T0 and T1). Indeed, previous experimental studies of meso-microcosms involving reworked sediment showed stabilization of oxygen fluxes and OPD after an equilibration period of 2–3 weeks (Ernst et al., 2002; Hansen and Blackburn, 1991, 1992; Porter et al., 2006). In the FLV microcosm, a steady state was set up from T1 until the end of the experiment despite recurrent additions of small volumes of sediment that did not affect OPD, whose values were similar to those of the control microcosm. In the OHV treatment, the addition of a large volume of sediment at once was most likely the driving factor for the deepening of the OPD at T1 (Fig. 3). Indeed, the sediment added in the microcosm settled by decantation to form a deeper oxygenated and water-enriched layer (Fig. 4). It then took up to a maximum of 3 weeks for the OPD to reach a level similar to that observed in the control and FLV microcosms during a steady state (T4, Fig. 3).
These results suggest that the large abrupt sediment supply could have a significant impact (p value <0.05) on OPD and as such could be a driver of redox front shifts and microhabitat disturbance. In contrast, recurrent low sediment supply, resulting in the deposition of thin layers, did not show significant differences to the control and thus may be considered to have only slight or negligible impacts on benthic habitats.
4.2 Effect of sediment disturbance on benthic foraminiferal abundances
A significant decreasing trend in total foraminiferal abundance is observed in the OHV treatment (Fig. 5). The foraminiferal living faunas are therefore more affected by the arrival of a higher amount of sediment at one time than by recurrent thinner inputs (FLV). This is in accordance with previous observations reported for marine areas subject to high sedimentary deposits, e.g. turbidites deposits. In fact, Tsujimoto et al. (2020) reported lower abundances of benthic foraminifera after the deposit of about 10 cm of sediment after the 2011 Tōhoku-oki earthquake due to burial-associated foraminiferal death. This matches with other previously reported observations after turbidite events (e.g. Bolliet et al., 2014; Hess and Jorissen, 2009). In the case of the study of Tsujimoto et al. (2020), a first recolonization of the superficial sediment by some of the species of the original assemblage (pre-turbidite) is observed within 5 months from the event, suggesting either the survival of some species in the thick sediment deposit (and migration towards the surface) or the recolonization of superficial sediment from refuge zones close to the sampling site. Since the recolonization from refuge zones was impossible in our set-up, our results suggest that part of the assemblage could survive this kind of deposit, at least during a short time interval (4 weeks). The presence of pre-event faunas on the recolonized sediment could be due to the remigration of buried faunas at the surface. The survival and reproduction of this fauna on longer timescales, however, were not assessed in our experiment. It is quite possible that assemblages facing a similar event within a natural environment would receive species coming from refuge zones, as suggested by long-term observations reported by (Bolliet et al., 2014; Hess and Jorissen, 2009; Tsujimoto et al., 2020).
The two other treatments of our set-up (control and FLV) did not show clear and significant trends, supporting the hypothesis that total foraminiferal abundances are not affected by frequent low-volume sediment inputs. However, the trends were significantly different when we looked at the two main species of our microcosms separately. In fact, Ammonia confertitesta did not suffer from a significant decline in abundance, neither in the control nor in the two treatments (Figs. 6 and 7a). The only significant linear regression was observed for the frequent low-volume microcosm where a slight increase of A. confertitesta abundance was observed through time. However, we believe that this result is untrustworthy as it was probably due to a lack of replicates for the T0 and T3. Our observations were restricted to the >125 µm fraction of faunas, only including adult specimens, so that we could exclude the possibility that reproductions during the experiment would be the reason for this increase.
In contrast, Haynesina germanica showed significant linear decreasing trends in the two disturbed microcosms (OHV and FLV) with time (Fig. 7b), suggesting that this species is more sensitive to all kinds (i.e. frequency and intensity) of burial than A. confertitesta. However, despite insignificant p values (0.08) and a lower R2 (0.37) than the two disturbed microcosms, a similar decreasing trend was also visible for the control microcosm. It is therefore difficult to completely attribute the decline of H. germanica to the different sediment inputs. The role of the experimental conditions in the species' response should be considered. Indeed, of the two main species used in our set-up, A. confertitesta (often reported as Ammonia tepida in the existing literature) was widely used as a target species in experimental studies, and it is known to tolerate laboratory conditions well and has also been used for longer time periods (i.e. days to months; e.g. Bradshaw, 1957; de Nooijer et al., 2009; Denoyelle et al., 2012; Geslin et al., 2014, 2004; Le Cadre and Debenay, 2006; Nardelli et al., 2014; Koho et al., 2018; Deldicq et al., 2020; Stouff et al., 1999), which is in agreement with our observations (Fig. 7a). Haynesina germanica has been rarely used in previous experimental set-ups and only in short-time experiments (i.e. hours to days; e.g. (Deldicq et al., 2021; Jauffrais et al., 2016b; Langlet, 2020; Seuront and Bouchet, 2015). The reason for the decreasing trend of H. germanica abundance in our control microcosm can be attributed to several experimental factors. It has a more restricted diet based on specific epipelic microalgae (Choquel, 2021, unpublished; Lee et al., 1989; Pillet et al., 2011) compared to the Ammonia group, which can alternatively feed on organic detritus, bacteria, and meiofauna (Dupuy et al., 2010; Mojtahid et al., 2011; Pascal et al., 2009; Wukovits et al., 2018). Moreover, recent experimental results showed that H. germanica's diet can switch from high-quality (low C:N values) to lower-quality organic material but that this switch often drives lowered fitness of the species (Wukovits et al., 2021). As the experiment was designed to observe the foraminiferal response to sedimentary deposits, we decided not to add extra organic matter during the experiment and to limit the tested variables. The consequent decrease of organic matter quality over time could have been unfavourable to H. germanica in competition with A. confertitesta. Moreover, it has been shown that H. germanica is a kleptoplastidic species that can assimilate undigested chloroplasts from specific microalgal preys (Choquel, 2021, unpublished; Jauffrais et al., 2016b; LeKieffre et al., 2018) and perform photosynthesis as an alternative metabolism (LeKieffre et al., 2018b). However, our experiments were mostly conducted in the dark (except at the sampling times) so this metabolism was unavailable to limit starvation.
4.3 Effect of sediment disturbance on benthic foraminiferal vertical distribution
4.3.1 Foraminiferal response to sedimentary deposits
According to the specific preferences, benthic foraminifera can have epifaunal to shallow infaunal (within the first 2 cm of sediment), intermediate (1–4 cm), or deep (>4 cm) infaunal microhabitats (Corliss, 1991). The two main species living in our microcosm are mainly epifaunal or shallow infaunal (Alve, 2001; Bouchet et al., 2009; Cesbron et al., 2016; Murray and Alve, 2000; Papaspyrou et al., 2013; Thibault de Chanvalon et al., 2015). This preferential shallow life position is obvious when no bioturbation-induced modification of the sedimentary microhabitats occurs (e.g. Alve, 2001; Cesbron et al., 2016; Jorissen et al., 1992; McCorkle et al., 1997; Mojtahid et al., 2010; Murray, 2006). In accordance with the literature, most of living individuals of these two species were always located in the uppermost centimetre of the control microcosm (Fig. 8a). Similarly, in both OHV and FLV microcosms, at T0 before the physical disturbance, most of the foraminifera were observed in the 0–0.2 cm layer. According to Jorissen et al. (1995), this shallow habitat preference in a not-food-limited environment such as the one in our microcosms is mainly driven by oxygen availability. In our microcosms, the oxygen penetration depths varied within a range of 1.2 and 2.2 mm below the sediment surface in all the aquaria at all sampling times. This means that, despite the significant OPD variations observed between T0 and T1, the oxic layers at all core tops were always thinner than the slicing resolution of 0.2 cm used for foraminiferal analysis. Therefore, it was impossible to determine a possible effect of OPD stabilization on the vertical distribution of the living foraminifera within the topmost 0.2 cm. Nevertheless, we can assess that the near absence of fauna below 0.2 cm depth could have been limited by oxygen availability. After the disturbances, in both the one-time high-volume and frequent low-volume microcosms, an upward migration of the fauna was observed within a short time, i.e. 18 h after each sediment addition. In the FLV treatment, the migration through the added sediment (0.2–0.5 cm) was rapid and seemed to follow the recovery of the oxic front in the uppermost layer (<0.2 cm, Figs. 8c and 3). The same dynamic was repeatedly observed at all successive sampling times (1 d after a new disturbance event) and therefore suggests that, in the FLV microcosm, the resilience of the microhabitat was achieved within 18 h after the sedimentary disturbance. This observation is in accordance with previous studies reporting rapid migration of epifaunal species after physical disturbance but largely reduces the recovery time, as previously reported (i.e. 22 d, Ernst et al., 2002). As we did not measure the dissolved-oxygen evolution between the moment of each sediment supply and subsequent sampling times, we cannot assess if this migration was performed under hypoxic conditions.
A rapid upward migration was also observed in the OHV treatment following the addition of a thick (2.9 cm after 1 d of compaction) layer of sediment (Fig. 8b). In this microcosm, however, at T1, no living individuals were able to reach the sediment–water interface. The observed unimodal distribution centred within the added sediment layer suggests that the migration started rapidly after the disturbance. At T2, the vertical distribution was comparable to the two other microcosms, with a peak at the surface, and it remained the same in the following sampling periods, suggesting that the recovery was achieved within 2 weeks after the disturbance.
In the OHV microcosm, the foraminiferal fauna was positioned between 0.8 and 3 cm depth at T1, being in its migration phase before reaching the surface at T2 (Fig. 8b). During this period of migration, the OPD was measured at 2.1±0.1 mm depth (Fig. 3), meaning that all the foraminifera had been moving through anoxic sediment layers. The possibility of migration of benthic foraminifera through anoxic sediment and towards oxygenated layers was already reported by Geslin et al. (2004) for deep-sea species. The shallow infaunal species we had in our microcosm, however, are generally reported as being sensitive to oxygen depletion in terms of motility. Despite several studies pointing out the ability of coastal foraminiferal species, including Ammonia spp., to survive days-to-months-long anoxia (e.g. Geslin et al., 2014; Nardelli et al., 2014), there is no consensus about their ability to actively move under anoxic conditions. In some studies, the vertical migration of Ammonia tepida (assimilated to Ammonia confertitesta here) was reported as being driven by the redox fronts. For example, Thibault de Chanvalon et al. (2015) attributed the observed bimodal distribution of this species in estuarine intertidal mudflats to the combination of downward burial by bioturbation and the ability of the specimens burrowed up to 3 cm deep in the sediment to move back to the surface. These authors suggested that A. tepida is able to detect the oxygenated layer through geochemical gradients of other chemical species (e.g. NO, Mn2+, or Fe2+). Other studies, however, highlighted the reduction or cessation of motility of A. tepida in the absence of oxygen and attributed this to a state of reduced metabolism or dormancy induced by the anoxia (e.g. Maire et al., 2016). In accord with this hypothesis, Koho et al. (2018) reported changes in Ammonia confertitesta ultrastructure as a stress response to oxygen depletion and suggested that these change could be related to dormancy (NB: in Koho et al., 2018). Ammonia confertitesta was mentioned as Ammonia sp. T6, one of the phylotypes of Ammonia distinguished by molecular identification (Holzmann and Pawlowski, 2000), and was renamed Ammonia confertitesta by Hayward et al. (2021). Additionally, in support of this theory, Le Kieffre et al. (2017) showed that Ammonia tepida highly reduces its metabolism and Corg uptake when exposed to anoxic conditions (LeKieffre et al., 2017). Our results rather support the hypothesis of Thibault de Chanvalon et al. (2015), stating that A. tepida would be able to follow redox fronts. The monitoring of the water column concentration of nutrients (Fig. 2) and oxygen penetration depth throughout the experiment (Fig. 3) gave us evidence of stabilization of sedimentary redox fronts 14 d after the first depositional event. Moreover, the presence of a Corg-enriched layer, corresponding to the original sediment surface, at 2.7 and 0.5 to 1.5 cm (respectively at T1 to T4) depth in the OHV and FLV microcosms did not seem to have influenced the upward migration, suggesting that oxygen, more than organic matter availability, was the major driving factor. Similarly, Haynesina germanica also showed high migration skills after the sedimentary disturbances. This species has recently been suggested to be able to move under low-oxygenated conditions and also to take advantage of the presence of existing trails to move into cohesive sediment (Deldicq et al., 2020). This agrees with our observations of rapid migration within 1 d after the FLV treatment and at a maximum of 1 week after the OHV treatment (Fig. 8c).
4.3.2 Vertical-migration speeds
Only a few studies quantified the locomotion speed of benthic foraminifera in the sediment (Bornmalm et al., 1997; Deldicq et al., 2021; Gross, 2000; Hemleben and Kitazato, 1995; Kitazato, 1988; Maire et al., 2016; Severin and Erskian, 1981). Some of them and additional studies quantified foraminiferal motion speeds in petri dishes with different substrates only focusing on horizontal movement (e.g. Bornmalm et al., 1997; Jauffrais et al., 2016a; Khare and Nigam, 2000; Kitazato, 1988; Maire et al., 2016; Seuront and Bouchet, 2015). In our study, we estimated the average speed of vertical migration of Ammonia confertitesta and Haynesina germanica through the added sediment in the two disturbed microcosms. We calculated the speeds based on the vertical distribution at T1 in the OHV microcosm because this was the only sampling time showing an ongoing migration, while the definitive life position was already reached in the other microcosms at this time. Our estimation assumes that the speed was constant over time (18 h from the sediment disturbance and T1) and that the locomotion started right at the moment of the sediment addition, which could have led to an underestimation of the speeds. A possible bias could also be added by the ∼1 cm sediment compaction observed during the 18 h (Fig. 4), which, on the other hand, could give an overestimation of the speed as a result. We calculated the specific mean speeds of A. confertitesta (0.41 mm h−1) and H. germanica (0.47 mm h−1) (Table 1). As none of the individuals reached the water–sediment interface 18 h after the disturbance, the calculated speeds were approximately maximum values. Recent studies from Deldicq et al. (2020) used flat aquaria to study the vertical and horizontal locomotion abilities of A. confertitesta and H. germanica in the sediment in two dimensions. Cameras tracked the migration pathways of specimens of both species over a short period of time, 48 to 72 h, in the absence of physical disturbances. Based on the distance travelled every 10 min, Deldicq et al. (2020) calculated average speeds for both species and obtained values of 0.72±0.25 mm h−1 for A. confertitesta and 1.1±0.4 mm h−1 for H. germanica (Table 1).
In our microcosms, the mean migration speeds of both species are on the same order of magnitude, with the speed of H. germanica being 2 times lower. If we retain the speeds reported by Deldicq et al. (2020), an average time of 40 and 26 h would have been needed for A. confertitesta and H. germanica respectively to go back to the water–sediment interface, which is consistent with our observations that no specimens had reached the sediment surface 18 h after the disturbance. The differences in speed values could be explained by methodological bias and/or ecological reasons. Indeed, we weighted the migration speeds on the basis of the number of specimens counted at each layer within a core, and our sampling resolution (18 h) was much lower than that (10 min) of Deldicq et al. (2020). If the migration activity is not homogeneous through time as assumed, the low resolution of our observation could have led to an underestimation of the actual speed. Additionally, as suggested by Maire et al. (2016), the presence of both anoxic conditions and potential stress induced by sediment disturbance in our OHV microcosm can be a major factor for lowering locomotion speeds. However, Kitazato (1988) and Khare and Nigam (2000) pointed out the overestimation of speed calculated from individuals presenting crawling-like movements on a glass surface as they encounter less resistance than from sediment matrix. Both this study and Maire et al. (2016) support the capacity of our species to cover a few centimetres of distance in a few hours. Differently from Maire et al. (2016), however, our results show that anoxic conditions do not induce a complete stop of the motility for A. confertitesta.
Table 1Summary of published foraminiferal vertical-migration speeds obtained in experimental sets. Bold font denotes mean values found in the literature.
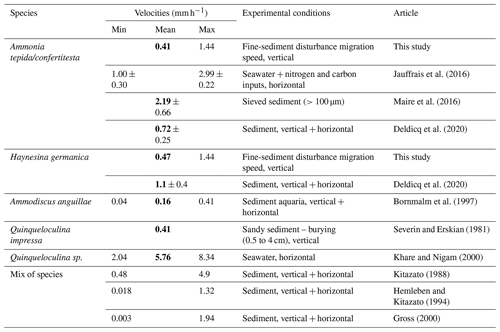
We compared our results to the experimental study conducted by Severin and Erskian (1981) that induced physical disturbances (from 0.5 to 4 cm of sediment suddenly added to the sediment surface containing living foraminifera) on a benthic foraminiferal species other than ours (i.e. Quinqueloculina impressa). The authors observed that the time of first emergence of this species after burial was a function of the deposit thickness as follows: T=434.3 D2, with T being the time of first emergence and D being the burial depth in centimetres. If we apply this relationship to the two species in the OHV microcosm, it would have taken 52.7 h, corresponding to 2.2 d, for the first individuals to reach the surface after crossing the 2.9 cm thick deposit (Table 1), corresponding to a speed of 0.55 mm h−1. Despite the methodological differences (different species, sandy sediment), our findings are in accordance with the results from Severin and Erskian (1981). In their model, the migration speeds are higher when foraminifera have to cross thinner layers. If we apply this model to the FLV treatment, for which speeds were not estimated, we would assume that the speeds would be higher for specimens crossing only a 0.5 cm thick layer of added sediment. For both studied species, based on the formula of Severin and Erskian (1981), we calculated average speeds of 2.7 mm h−1, which are almost 3 times higher than the ones reported by Deldicq et al. (2020). The reliability of this value should be tested in further specific studies. Nevertheless, these findings further suggest that the stress induced by physical disturbances and the amplitude of the disturbance (in terms of the thickness of the sediment deposit) can be a controlling factor influencing foraminiferal migration speed.
4.4 General overview on benthic communities' response to depositional events
The existing literature about the effects of sediment deposition on benthic communities in experimental set-ups mainly concentrates on macro- and megafauna and is limited to relatively short-lasting experiments (from 4 to 32 d). Some interesting observations can be pointed out. Mestdagh et al. (2018), for example, simulated a sudden deposit of about 4 cm of sediment and observed a complete recovery of different species of molluscs and crustacean within 15 d after the disturbance. No decay of abundances of certain species was observed, indicating that some macrofaunal species can deal with 4 cm depth burial without problems. Only individuals with sessile behaviours showed a wide mortality. Similarly, Cottrell et al. (2016) observed a weak migration ability of Mytilus edulis through thin added-sediment layers (<2 cm) but no migration below thicker deposits. The species mortality was also observed to be largely affected by burial duration, increased temperature, and anoxia induced by organic matter mineralization. This was attributed to a short-term tolerance of anoxia, which was not sufficient to overcome oxygen depletion on longer timescales.
Whomersley et al. (2009), in a 9-month-long study focusing on the effect of different frequencies of depositional events on both macro- and meiofauna, showed different responses of the two faunal types in terms of diversity, species abundances, and turnover. Compared to Mestdagh et al. (2018), they observed a more intense impact of burial on macrofauna, with decreasing diversity under both low and high depositional intensity. Macrofauna was also generally more impacted than meiofauna (represented here by nematodes), especially by the low-frequency burial. However, a shift in communities' species composition was only noticed for meiofauna, while no effects were observed on abundances, suggesting a more rapid species turnover under stressful conditions. Similarly to our results, the high intensity of burial seems to further affect their communities compared to weaker deposits (Whomersley et al., 2009). However, contrary to what is reported for nematodes, in our study, we observed a significant decay in foraminiferal abundances in the one-time high-volume microcosm (OHV, Fig. 5). This difference could be related to lower motility and/or turnover rates of foraminifera compared to nematodes or to the short duration of our experiment (51 d) compared to the one of Whomersley et al. (2009) (9 months). The fact that the vertical migration of living foraminifera through newly deposited layers in the OHV treatment was way longer (1 to 7 d) than for the FLV (<18 h) supports the hypothesis of the low motility as the main limitation to survival after burial. Compared to foraminifera, nematodes are much more mobile and possibly able to reach favourable niches faster after a physical disturbance. This lets us conclude that the response, even for the same faunal type (i.e. meiofauna), can be variable. It is therefore crucial to study the response of different components of benthic compartments for the assessment of the effects of physical disturbance in the benthic marine environments.
The knowledge about the specific responses of different faunal types is also important when considering the trophic links between the compartments. For example, Bolam et al. (2011) suggested that trophic-network disruption, caused by physical instability, can affect macrofaunal response to depositional events. The study simulated the impact of dredged sediment upon macrofaunal assemblages and showed different migration abilities for different macrofaunal taxa (e.g. polychaete that are less performant than gastropods) after burial. The authors suggested that the observed survival of successful migrating species can be overestimated in short-time observations because of the dependence on the migration of other co-existing species including prey in a long-term dynamic. In this way, it appears to be fundamental to deepen the knowledge of the response of species from lower trophic levels to similar physical disturbances to holistically interpret the experimental observation at the scale of the whole benthic community.
If we consider all these observations together, we can therefore conclude that, under a condition of physical instability, benthic communities respond in a species-specific way in terms of abundances, diversity, and migration. Less mobile and highly specialist species are negatively affected compared to highly mobile and less trophically restricted species. The main factors influencing the community resilience seem to be turnover time, organic matter and/or oxygen availability, burial depth, frequency of sediment depositions, and migration of co-existing species. The interaction of these parameters can complicate the prevision of the long-term consequences of similar physical conditions in natural settings, but a loss of more sensitive species, both from macrofauna and meiofauna, can be supposed on the basis of the existing experimental results.
Physical disturbances are often neglected as an important driving factor ecologically influencing biodiversity and standing stocks. The ongoing climate change is supposed to, at least regionally or locally, affect the natural variability of sediment input from the continent to coastal environments. The lack of information about the potential consequences for benthic faunal abundances and diversity could be a strong limit to imagining ecosystem resilience scenarios.
The results of our experimental study suggest that benthic foraminiferal assemblages respond differently to sedimentary depositional events of different intensity and thickness. On the one hand, the total foraminiferal abundances were significantly negatively affected only by the one-time high-volume treatment, suggesting that occasional and thick sediment deposits potentially have a higher impact on standing stocks compared to a frequent, lower stress (represented by the frequent low-volume treatment). On the other hand, both types of tested sedimentary disturbances appeared to negatively influence the abundances of one of the two major species of the set-up, Haynesina germanica. This result suggests that the tolerance of this species to the physical disturbance, no matter its intensity and frequency, is lower than the one of Ammonia confertitesta. In a natural environment, this could mean that a lowered biodiversity can be driven by physical disturbance.
At the scale of microhabitat distribution in the sediment, while the recovery of shallow microhabitats by the tested species was very quick after the frequent low-volume deposit (<24 h), the one-time high-volume treatment induced longer recovery times (i.e. ≤7 d). This difference is also reflected in the geochemical steady state of the porewater. Indeed, the recovery of oxygen penetration depth, similarly to the one at the first foraminifera sampling, was relatively quick for the FLV microcosm (<24 h after each disturbance), while a transitory deepening of the OPD was observed later (T1) in the OHV microcosm (24 h after the disturbance), and a resilient steady state was not reached until 38 (T2) to 52 d (T4) after the disturbance.
The recovery of superficial microhabitats by buried specimens, however, does not seem to be strictly driven by the oxic front. In the OHV microcosm, foraminifera migrated through a thick anoxic sediment layer to reach the water–sediment interface. Considering that the added sediment layer was not enriched in organic matter and that the most food-enriched area of the microcosm was probably the ancient interface (see black layer in Fig. 4), we can conclude that the upward migration was not driven by food research but rather, most likely, by oxygen depletion.
This scientific article is a part of Corentin Guilhermic's PhD project. The data shown and discussed here are a part of a bigger and wider dataset which will be available to the public later during the PhD. All data used and produced in this work are available in the figures and tables presented in the paper.
CG, MPN, AM, and HH designed the experiment; CG, MPN, AM, and DLM performed the sampling procedures and measurements; CG analysed the data; CG and MPN wrote the original paper draft; MPN, AM and HH reviewed and edited the paper.
The contact author has declared that none of the authors has any competing interests.
Publisher’s note: Copernicus Publications remains neutral with regard to jurisdictional claims in published maps and institutional affiliations.
We thank Miroiterie Nogentaise Inc. for providing custom-made aquaria following all our requirements. Thanks is given to Sophie Sanchez for her technical help in the laboratory and to the bachelor students who helped us in collecting the foraminifera from the mudflat and in sieving core slices. The first author is grateful to Edouard Metzger for his valuable advice. We also thank Robin Fentimen for reading the last version of the paper and improving the English language. We thank the three anonymous reviewers and the editor who helped to improve the original version of this paper.
The CNRS INSU LEFE CYBER programme (Fluid Envelopes and the Environment) supported our research by funding the BEGIN project (PI: Maria Pia Nardelli). This research is part of a PhD project (first author) funded by the French Ministry of Scientific Research and Innovation and by Angers University.
This paper was edited by Chiara Borrelli and reviewed by three anonymous referees.
Alve, E.: Colonization of new habitats by benthic foraminifera: a review, Earth-Sci. Rev., 46, 167–185, https://doi.org/10.1016/S0012-8252(99)00016-1, 1999.
Alve, E.: Temporal variability in vertical distributions of live (stained) intertidal foraminifera, Southern England, J. Foramin. Res., 31, 12–24, https://doi.org/10.2113/0310012, 2001.
Alve, E., Korsun, S., Schönfeld, J., Dijkstra, N., Golikova, E., Hess, S., Husum, K., and Panieri, G.: Foram-AMBI: A sensitivity index based on benthic foraminiferal faunas from North-East Atlantic and Arctic fjords, continental shelves and slopes, Mar. Micropaleontol., 122, 1–12, https://doi.org/10.1016/j.marmicro.2015.11.001, 2016.
Anschutz, P., Jorissen, F. J., Chaillou, G., Abu-Zied, R., and Fontanier, C.: Recent turbidite deposition in the eastern Atlantic: Early diagenesis and biotic recovery, J. Mar. Res., 60, 835–854, https://doi.org/10.1357/002224002321505156, 2002.
Barras, C., Jorissen, F. J., Labrune, C., Andral, B., and Boissery, P.: Live benthic foraminiferal faunas from the French Mediterranean Coast: Towards a new biotic index of environmental quality, Ecol. Ind., 36, 719–743, https://doi.org/10.1016/j.ecolind.2013.09.028, 2014.
Belart, P., Clemente, I., Raposo, D., Habib, R., Volino, E., Villar, A., Alves, M., Fontana, L., Lorini, M., Panigai, G., Frontalini, F., Figueiredo, M., Vasconcelos, S., and Laut, L.: Living and dead Foraminifera as bioindicators in Saquarema Lagoon System, Brazil, Lat. Am. J. Aquat. Res., 46, 1055–1072, https://doi.org/10.3856/vol46-issue5-fulltext-18, 2018.
Bernhard, J. M. and Bowser, S. S.: Novel epifluorescence microscopy method to determine life position of foraminifera in sediments, J. Micropalaeontol., 15, 68–68, https://doi.org/10.1144/jm.15.1.68, 1996.
Bernhard, J. M., Ostermann, D. R., Williams, D. S., and Blanks, J. K.: Comparison of two methods to identify live benthic foraminifera: A test between Rose Bengal and CellTracker Green with implications for stable isotope paleoreconstructions: foraminifera viability method comparison, Paleoceanography, 21, PA4110, https://doi.org/10.1029/2006PA001290, 2006.
Bolam, S. G.: Burial survival of benthic macrofauna following deposition of simulated dredged material, Environ. Monit. Assess., 181, 13–27, https://doi.org/10.1007/s10661-010-1809-5, 2011.
Bolliet, T., Jorissen, F. J., Schmidt, S., and Howa, H.: Benthic foraminifera from Capbreton Canyon revisited; faunal evolution after repetitive sediment disturbance, Deep-Sea Res. Pt. II, 104, 319–334, https://doi.org/10.1016/j.dsr2.2013.09.009, 2014.
Bornmalm, L., Corliss, B. H., and Tedesco, K.: Laboratory observations of rates and patterns of movement of continental margin benthic foraminifera, Mar. Micropaleontol., 29, 175–184, https://doi.org/10.1016/S0377-8398(96)00013-8, 1997.
Bouchet, V. M. P., Sauriau, P.-G., Debenay, J.-P., Mermillod-Blondin, F., Schmidt, S., Amiard, J.-C., and Dupas, B.: Influence of the mode of macrofauna-mediated bioturbation on the vertical distribution of living benthic foraminifera: First insight from axial tomodensitometry, J. Exp. Mar. Biol. Ecol., 371, 20–33, https://doi.org/10.1016/j.jembe.2008.12.012, 2009.
Bouchet, V. M. P., Alve, E., Rygg, B., and Telford, R. J.: Benthic foraminifera provide a promising tool for ecological quality assessment of marine waters, Ecol. Indicat., 23, 66–75, https://doi.org/10.1016/j.ecolind.2012.03.011, 2012.
Bouchet, V. M. P., Goberville, E., and Frontalini, F.: Benthic foraminifera to assess Ecological Quality Statuses in Italian transitional waters, Ecol. Indicat., 84, 130–139, https://doi.org/10.1016/j.ecolind.2017.07.055, 2018a.
Bouchet, V. M. P., Telford, R. J., Rygg, B., Oug, E., and Alve, E.: Can benthic foraminifera serve as proxies for changes in benthic macrofaunal community structure?, Implications for the definition of reference conditions, Mar. Environ. Res., 137, 24–36, https://doi.org/10.1016/j.marenvres.2018.02.023, 2018b.
Bradshaw, J. S.: Laboratory studies on the rate of growth of the foraminifer, “Streblus beccarii (linné) var. tepida (Cushman)”, J. Paleontol., 31, 1138–1147, 1957.
Budillon, F., Vicinanza, D., Ferrante, V., and Iorio, M.: Sediment transport and deposition during extreme sea storm events at the Salerno Bay (Tyrrhenian Sea): comparison of field data with numerical model results, Nat. Hazards Earth Syst. Sci., 6, 839–852, https://doi.org/10.5194/nhess-6-839-2006, 2006.
Bussi, G., Dadson, S. J., Prudhomme, C., and Whitehead, P. G.: Modelling the future impacts of climate and land-use change on suspended sediment transport in the River Thames (UK), J. Hydrol., 542, 357–372, https://doi.org/10.1016/j.jhydrol.2016.09.010, 2016.
Cesbron, F., Geslin, E., Jorissen, F. J., Delgard, M. L., Charrieau, L., Deflandre, B., Jézéquel, D., Anschutz, P., and Metzger, E.: Vertical distribution and respiration rates of benthic foraminifera: Contribution to aerobic remineralization in intertidal mudflats covered by Zostera noltei meadows, Estuar. Coast. Shelf Sci., 179, 23–38, https://doi.org/10.1016/j.ecss.2015.12.005, 2016.
Choquel, C.: Ecologie des foraminifères benthiques, interactions biologiques et géochimiques ; approche pluridisciplinaire à différentes échelles, Angers, 2021.
Choquel, C., Geslin, E., Metzger, E., Filipsson, H. L., Risgaard-Petersen, N., Launeau, P., Giraud, M., Jauffrais, T., Jesus, B., and Mouret, A.: Denitrification by benthic foraminifera and their contribution to N-loss from a fjord environment, Biogeosciences, 18, 327–341, https://doi.org/10.5194/bg-18-327-2021, 2021.
Contreras-Rosales, L. A., Koho, K. A., Duijnstee, I. A. P., de Stigter, H. C., García, R., Koning, E., and Epping, E.: Living deep-sea benthic foraminifera from the Cap de Creus Canyon (western Mediterranean): Faunal–geochemical interactions, Deep-Sea Res. Pt. I, 64, 22–42, https://doi.org/10.1016/j.dsr.2012.01.010, 2012.
Corliss, B. H.: Morphology and microhabitat preferences of benthic foraminifera from the northwest Atlantic Ocean, Mar. Micropaleontol., 17, 195–236, https://doi.org/10.1016/0377-8398(91)90014-W, 1991.
Cottrell, R. S., Black, K. D., Hutchison, Z. L., and Last, K. S.: The Influence of Organic Material and Temperature on the Burial Tolerance of the Blue Mussel, Mytilus edulis: Considerations for the Management of Marine Aggregate Dredging, PLOS ONE, 11, e0147534, https://doi.org/10.1371/journal.pone.0147534, 2016.
D'Angelo, A., Giglio, F., Miserocchi, S., Sanchez-Vidal, A., Aliani, S., Tesi, T., Viola, A., Mazzola, M., and Langone, L.: Multi-year particle fluxes in Kongsfjorden, Svalbard, Biogeosciences, 15, 5343–5363, https://doi.org/10.5194/bg-15-5343-2018, 2018. 18.
Deldicq, N., Seuront, L., Langlet, D., and Bouchet, V.: Assessing behavioural traits of benthic foraminifera: implications for sediment mixing, Mar. Ecol. Prog. Ser., 643, 21–31, https://doi.org/10.3354/meps13334, 2020.
Deldicq, N., Langlet, D., Delaeter, C., Beaugrand, G., Seuront, L., and Bouchet, V. M. P.: Effects of temperature on the behaviour and metabolism of an intertidal foraminifera and consequences for benthic ecosystem functioning, Sci. Rep., 11, 4013, https://doi.org/10.1038/s41598-021-83311-z, 2021.
Denoyelle, M., Jorissen, F. J., Martin, D., Galgani, F., and Miné, J.: Comparison of benthic foraminifera and macrofaunal indicators of the impact of oil-based drill mud disposal, Mar. Pollut. Bull., 60, 2007–2021, https://doi.org/10.1016/j.marpolbul.2010.07.024, 2010.
Denoyelle, M., Geslin, E., Jorissen, F. J., Cazes, L., and Galgani, F.: Innovative use of foraminifera in ecotoxicology: A marine chronic bioassay for testing potential toxicity of drilling muds, Ecol. Indicat., 12, 17–25, https://doi.org/10.1016/j.ecolind.2011.05.011, 2012.
Dessandier, P.-A., Bonnin, J., Kim, J.-H., Bichon, S., Deflandre, B., Grémare, A., and Sinninghe Damsté, J. S.: Impact of organic matter source and quality on living benthic foraminiferal distribution on a river-dominated continental margin: A study of the Portuguese margin: benthic foraminifera, J. Geophys. Res.-Biogeo., 121, 1689–1714, https://doi.org/10.1002/2015JG003231, 2016.
Dudgeon, D.: Multiple threats imperil freshwater biodiversity in the Anthropocene, Curr. Biol., 29, 960–967, https://doi.org/10.1016/j.cub.2019.08.002, 2019.
Dupuy, C., Rossignol, L., Geslin, E., and Pascal, P.-Y.: Predation of mudflat meio-macrofaunal metazoans by a calcareous foraminifer, Ammonia tepida (Cushman, 1926), J. Foramin. Res., 40, 305–312, https://doi.org/10.2113/gsjfr.40.4.305, 2010.
Duros, P., Silva Jacinto, R., Dennielou, B., Schmidt, S., Martinez Lamas, R., Gautier, E., Roubi, A., and Gayet, N.: Benthic foraminiferal response to sedimentary disturbance in the Capbreton canyon (Bay of Biscay, NE Atlantic), Deep-Sea Res. Pt. I, 120, 61–75, https://doi.org/10.1016/j.dsr.2016.11.012, 2017.
Dyer, K. R.: Fine Sediment Particle Transport in Estuaries, in: Physical Processes in Estuaries, edited by: Dronkers, J. and van Leussen, W., Springer Berlin Heidelberg, Berlin, Heidelberg, 295–310, https://doi.org/10.1007/978-3-642-73691-9_ 16, 1988.
Ernst, S., Duijnstee, I., and van der Zwaan, B.: The dynamics of the benthic foraminiferal microhabitat: recovery after experimental disturbance, Mar. Micropaleontol., 46, 343–361, https://doi.org/10.1016/S0377-8398(02)00080-4, 2002.
Extence, C., P. Chadd, R., England, J., J. Dunbar, M., J. Wood, P., and Taylor, E.: The assessment of fine sediment accumulation in rivers using macro-invertebrate community response: macro-invertebrate assessment of fine sediment accumulation, River Res. Appl., 29, 17–55, https://doi.org/10.1002/rra.1569, 2013.
Fontanier, C., Dissard, D., Ruffine, L., Mamo, B., Ponzevera, E., Pelleter, E., Baudin, F., Roubi, A., Chéron, S., Boissier, A., Gayet, N., Bermell-Fleury, S., Pitel, M., Guyader, V., Lesongeur, F., and Savignac, F.: Living (stained) deep-sea foraminifera from the Sea of Marmara: A preliminary study, Deep-Sea Res. Pt. II, 153, 61–78, https://doi.org/10.1016/j.dsr2.2017.12.011, 2018.
Fontanier, C., Mamo, B., Mille, D., Duros, P., and Herlory, O.: Deep-sea benthic foraminifera at a bauxite industrial waste site in the Cassidaigne Canyon (NW Mediterranean): Ten months after the cessation of red mud dumping, Comptes Rendus. Géosci., 352, 87–101, https://doi.org/10.5802/crgeos.5, 2020.
Fossile, E., Nardelli, M. P., Howa, H., Baltzer, A., Poprawski, Y., Baneschi, I., Doveri, M., and Mojtahid, M.: Influence of modern environmental gradients on foraminiferal faunas in the inner Kongsfjorden (Svalbard), Mar. Micropaleontol., 173, 102117, https://doi.org/10.1016/j.marmicro.2022.102117, 2022.
Frontalini, F. and Coccioni, R.: Benthic foraminifera for heavy metal pollution monitoring: A case study from the central Adriatic Sea coast of Italy, Estuar. Coast. Shelf Sci., 76, 404–417, https://doi.org/10.1016/j.ecss.2007.07.024, 2008.
Frontalini, F., Buosi, C., Da Pelo, S., Coccioni, R., Cherchi, A., and Bucci, C.: Benthic foraminifera as bio-indicators of trace element pollution in the heavily contaminated Santa Gilla lagoon (Cagliari, Italy), Mar. Pollut. Bull., 58, 858–877, https://doi.org/10.1016/j.marpolbul.2009.01.015, 2009.
García-Robledo, E., Corzo, A., and Papaspyrou, S.: A fast and direct spectrophotometric method for the sequential determination of nitrate and nitrite at low concentrations in small volumes, Mar. Chem., 162, 30–36, https://doi.org/10.1016/j.marchem.2014.03.002, 2014.
Geslin, E., Heinz, P., Jorissen, F., and Hemleben, C.: Migratory responses of deep-sea benthic foraminifera to variable oxygen conditions: laboratory investigations, Mar. Micropaleont., 53, 227–243, https://doi.org/10.1016/j.marmicro.2004.05.010, 2004.
Geslin, E., Barras, C., Langlet, D., Nardelli, M. P., Kim, J.-H., Bonnin, J., Metzger, E., and Jorissen, F. J.: Survival, reproduction and calcification of three benthic foraminiferal species in response to experimentally induced hypoxia, in: Approaches to Study Living Foraminifera, edited by: Kitazato, H. and Bernhard, J., Springer Japan, Tokyo, 163–193, https://doi.org/10.1007/978-4-431-54388-6_ 10, 2014.
Goineau, A., Fontanier, C., Jorissen, F., Buscail, R., Kerhervé, P., Cathalot, C., Pruski, A. M., Lantoine, F., Bourgeois, S., Metzger, E., Legrand, E., and Rabouille, C.: Temporal variability of live (stained) benthic foraminiferal faunas in a river-dominated shelf – Faunal response to rapid changes of the river influence (Rhône prodelta, NW Mediterranean), Biogeosciences, 9, 1367–1388, https://doi.org/10.5194/bg-9-1367-2012, 2012.
Gooday, A. J., Bernhard, J. M., Levin, L. A., and Suhr, S. B.: Foraminifera in the Arabian Sea oxygen minimum zone and other oxygen-deficient settings: taxonomic composition, diversity, and relation to metazoan faunas, Deep-Sea Res. Pt. II, 47, 25–54, 2000.
Griess, P.: Bemerkungen zu der Abhandlung der HH, Weselsky und Benedikt “Ueber einige Azoverbindungen”?, Ber. Dtsch. Chem. Ges., 12, 426–428, https://doi.org/10.1002/cber.187901201117, 1879.
Gross, O.: Influence of temperature, oxygen and food availability on the migrational activity of bathyal benthic foraminifera: evidence by microcosm experiments, in: Life at Interfaces and Under Extreme Conditions, edited by: Liebezeit, G., Dittmann, S., and Kröncke, I., Springer Netherlands, Dordrecht, 123–137, https://doi.org/10.1007/978-94-011-4148-2_ 12, 2000.
Hansen, L. and Blackburn, T.: Aerobic and anaerobic mineralization of organic material in marine sediment microcosms, Mar. Ecol. Prog. Ser., 75, 283–291, https://doi.org/10.3354/meps075283, 1991.
Hansen, L. S. and Blackburn, T. H.: Mineralization budgets in sediment microcosms: Effect of the infauna and anoxic conditions, FEMS Microb. Lett., 102, 33–43, https://doi.org/10.1111/j.1574-6968.1992.tb05793.x, 1992.
Hayward, B. W., Holzmann, M., Pawlowski, J., Parker, J. H., Kaushik, T., Toyofuku, M. S., and Tsuchiya, M.: Molecular and morphological taxonomy of living Ammonia and related taxa (Foraminifera) and their biogeography, Micropaleontology, 67, 109–274, https://doi.org/10.47894/mpal.67.3.01, 2021.
Heinz, P. and Geslin, E.: Ecological and Biological Response of Benthic Foraminifera Under Oxygen-Depleted Conditions: Evidence from Laboratory Approaches, in: Anoxia, vol. 21, edited by: Altenbach, A. V., Bernhard, J. M., and Seckbach, J., Springer Netherlands, Dordrecht, 287–303, https://doi.org/10.1007/978-94-007-1896-8_ 15, 2012.
Hemleben, C. and Kitazato, H.: Deep-sea foraminifera under long time observation in the laboratory, Deep-Sea Res. Pt. I, 42, 827–832, https://doi.org/10.1016/0967-0637(95)00024-Z, 1995.
Hess, S. and Jorissen, F. J.: Distribution patterns of living benthic foraminifera from Cap Breton canyon, Bay of Biscay: Faunal response to sediment instability, Deep-Sea Res. Pt. I, 56, 1555–1578, https://doi.org/10.1016/j.dsr.2009.04.003, 2009.
Hess, S., Alve, E., Trannum, H. C., and Norling, K.: Benthic foraminiferal responses to water-based drill cuttings and natural sediment burial: Results from a mesocosm experiment, Mar. Micropaleontol., 101, 1–9, https://doi.org/10.1016/j.marmicro.2013.03.004, 2013.
Hir, P. L., Ficht, A., Jacinto, R. S., Lesueur, P., Dupont, J.-P., Lafite, R., Brenon, I., Thouvenin, B., and Cugier, P.: Fine Sediment Transport and Accumulations at the Mouth of the Seine Estuary (France), Estuaries, 24, 950, https://doi.org/10.2307/1353009, 2001.
Hodson, A., Gurnell, A., Tranter, M., Bogen, J., Hagen, J. O., and Clark, M.: Suspended sediment yield and transfer processes in a small High-Arctic glacier basin, Svalbard, Hydrol. Process., 12, 73–86, https://doi.org/10.1002/(SICI)1099-1085(199801)12:1< 73::AID-HYP564>3.0.CO;2-S, 1998.
Holzmann, M. and Pawlowski, J.: Taxonomic relationships in the genus Ammonia (Foraminifera) based on ribosomal DNA sequences, J. Micropaleontol., 19, 11, https://doi.org/10.1144/jm.19.1.85, 2000.
Jalón-Rojas, I., Schmidt, S., and Sottolichio, A.: Turbidity in the fluvial Gironde Estuary (southwest France) based on 10-year continuous monitoring: sensitivity to hydrological conditions, Hydrol. Earth Syst. Sci., 19, 2805–2819, https://doi.org/10.5194/hess-19-2805-2015, 2015.
Jauffrais, T., Jesus, B., Metzger, E., Mouget, J.-L., Jorissen, F., and Geslin, E.: Effect of light on photosynthetic efficiency of sequestered chloroplasts in intertidal benthic foraminifera (Haynesina germanica and Ammonia tepida), Biogeosciences, 13, 2715–2726, https://doi.org/10.5194/bg-13-2715-2016, 2016a.
Jauffrais, T., Jesus, B., Geslin, E., Briand, F., and Jézéquel, V. M.: Locomotion speed of the benthic foraminifer Ammonia tepida exposed to different nitrogen and carbon sources, J. Sea Res., 118, 52–58, https://doi.org/10.1016/j.seares.2016.07.001, 2016b.
Jørgensen, B. B.: The sulfur cycle of a coastal marine sediment (Limfjorden, Denmark)1: Sulfur cycle of marine sediment, Limnol. Oceanogr., 22, 814–832, https://doi.org/10.4319/lo.1977.22.5.0814, 1977.
Jorissen, F. J.: Benthic foraminiferal microhabitats below the sediment-water interface, in: Modern Foraminifera, Springer Netherlands, Dordrecht, 161–179, https://doi.org/10.1007/0-306-48104-9_ 10, 1999.
Jorissen, F. J., Barmawidjaja, D. M., Puskaric, S., and van der Zwaan, G. J.: Vertical distribution of benthic foraminifera in the northern Adriatic Sea: The relation with the organic flux, Mar. Micropaleontol., 19, 131–146, https://doi.org/10.1016/0377-8398(92)90025-F, 1992.
Jorissen, F. J., de Stigter, H. C., and Widmark, J. G. V.: A conceptual model explaining benthic foraminiferal microhabitats, Mar. Micropaleontol., 26, 3–15, https://doi.org/10.1016/0377-8398(95)00047-X, 1995.
Jorissen, F. J., Fouet, M. P. A., Singer, D., and Howa, H.: The Marine Influence Index (MII): a tool to assess estuarine intertidal mudflat environments for the purpose of foraminiferal biomonitoring, Water, 14, 676, https://doi.org/10.3390/w14040676, 2022.
Khare, N. and Nigam, R.: Laboratory experiment to record rate of movement of cultured benthic foraminifera, ONGC Bull., 37, 53–61, 2000.
Kitazato, H.: Locomotion of some benthic foraminifera in and on sediments, J. Foramin. Res., 18, 344–349, https://doi.org/10.2113/gsjfr.18.4.344, 1988.
Koho, K. A., de Nooijer, L. J., and Reichart, G. J.: Combining benthic foraminiferal ecology and shell to deconvolve past bottom water oxygenation and paleoproductivity, Geochim. Cosmochim. Ac., 165, 294–306, https://doi.org/10.1016/j.gca.2015.06.003, 2015.
Koho, K. A., LeKieffre, C., Nomaki, H., Salonen, I., Geslin, E., Mabilleau, G., Søgaard Jensen, L. H., and Reichart, G.-J.: Changes in ultrastructural features of the foraminifera Ammonia spp. in response to anoxic conditions: Field and laboratory observations, Mar. Micropaleont., 138, 72–82, https://doi.org/10.1016/j.marmicro.2017.10.011, 2018.
Kristensen, E. and Blackburn, T. H.: The fate of organic carbon and nitrogen in experimental marine sediment systems: Influence of bioturbation and anoxia, J. Mar. Res., 45, 231–257, https://doi.org/10.1357/002224087788400927, 1987.
Kuhnle, R. A., Bingner, R. L., Foster, G. R., and Grissinger, E. H.: Effect of land use changes on sediment transport in Goodwin Creek, Water Resour. Res., 32, 3189–3196, https://doi.org/10.1029/96WR02104, 1996.
Langezaal, A. M., Jorissen, F. J., Braun, B., Chaillou, G., Fontanier, C., Anschutz, P., and van der Zwaan, G. J.: The influence of seasonal processes on geochemical profiles and foraminiferal assemblages on the outer shelf of the Bay of Biscay, Cont. Shelf Res., 26, 1730–1755, https://doi.org/10.1016/j.csr.2006.05.005, 2006.
Langlet, D.: Motion behavior and metabolic response to microplastic leachates in the benthic foraminifera Haynesina germanica, J. Exp. Mar. Biol. Ecol., 6, 151395, https://doi.org/10.1016/j.jembe.2020.151395, 2020.
Laut, L., da Matta, G., Camara, G., Belart, P., Clemente, I., Ballalai, J., Volino, E., and Couto, E. C. G.: Living and dead foraminifera assemblages as environmental indicators in the Almada River Estuary, Ilhéus, northeastern Brazil, J. South Am. Earth Sci., 105, 102883, https://doi.org/10.1016/j.jsames.2020.102883, 2021.
Larson, F. and Sundbäck, K.: Recovery of microphytobenthos and benthic functions after sediment deposition, Mar. Ecol. Prog. Ser., 446, 31–44, https://doi.org/10.3354/meps09488, 2012.
Le Cadre, V. and Debenay, J.-P.: Morphological and cytological responses of Ammonia (foraminifera) to copper contamination: Implication for the use of foraminifera as bioindicators of pollution, Environ. Pollut., 143, 304–317, https://doi.org/10.1016/j.envpol.2005.11.033, 2006.
Lee, J. J., McEnery, M. E., Kuile, B. T., Erez, J., Röttger, R., Rockwell, R. F., Faber, W. W., Lagziel, A., and Rottger, R.: Identification and Distribution of Endosymbiotic Diatoms in Larger Foraminifera, Micropaleontology, 35, 353, https://doi.org/10.2307/1485677, 1989.
Lehto, N. J., Larsen, M., Zhang, H., Glud, R. N., and Davison, W.: A mesocosm study of oxygen and trace metal dynamics in sediment microniches of reactive organic material, Sci. Rep., 7, 11369, https://doi.org/10.1038/s41598-017-10179-3, 2017.
LeKieffre, C., Spangenberg, J. E., Mabilleau, G., Escrig, S., Meibom, A., and Geslin, E.: Surviving anoxia in marine sediments: The metabolic response of ubiquitous benthic foraminifera (Ammonia tepida), PLoS ONE, 12, e0177604, https://doi.org/10.1371/journal.pone.0177604, 2017.
LeKieffre, C., Bernhard, J. M., Mabilleau, G., Filipsson, H. L., Meibom, A., and Geslin, E.: An overview of cellular ultrastructure in benthic foraminifera: New observations of rotalid species in the context of existing literature, Mar. Micropaleont., 138, 12–32, https://doi.org/10.1016/j.marmicro.2017.10.005, 2018a.
LeKieffre, C., Jauffrais, T., Geslin, E., Jesus, B., Bernhard, J. M., Giovani, M.-E., and Meibom, A.: Inorganic carbon and nitrogen assimilation in cellular compartments of a benthic kleptoplastic foraminifer, Sci. Rep., 8, 10140, https://doi.org/10.1038/s41598-018-28455-1, 2018b.
Maire, O., Barras, C., Gestin, T., Nardelli, M., Romero-Ramirez, A., Duchêne, J., and Geslin, E.: How does macrofaunal bioturbation influence the vertical distribution of living benthic foraminifera?, Mar. Ecol. Prog. Ser., 561, 83–97, https://doi.org/10.3354/meps11929, 2016.
Martins, V. A., Frontalini, F., Tramonte, K. M., Figueira, R. C. L., Miranda, P., Sequeira, C., Fernández-Fernández, S., Dias, J. A., Yamashita, C., Renó, R., Laut, L. L. M., Silva, F. S., Rodrigues, M. A. C., Bernardes, C., Nagai, R., Sousa, S. H. M., Mahiques, M., Rubio, B., Bernabeu, A., Rey, D., and Rocha, F.: Assessment of the health quality of Ria de Aveiro (Portugal): Heavy metals and benthic foraminifera, Mar. Pollut. Bull., 70, 18–33, https://doi.org/10.1016/j.marpolbul.2013.02.003, 2013.
Martins, M. V. A., Silva, F., Laut, L. L. M., Frontalini, F., Clemente, I. M. M. M., Miranda, P., Figueira, R., Sousa, S. H. M., and Dias, J. M. A.: Response of Benthic Foraminifera to Organic Matter Quantity and Quality and Bioavailable Concentrations of Metals in Aveiro Lagoon (Portugal), PLoS ONE, 10, e0118077, https://doi.org/10.1371/journal.pone.0118077, 2015.
Martins, M. V. A., Pinto, A. F. S., Frontalini, F., da Fonseca, M. C. M., Terroso, D. L., Laut, L. L. M., Zaaboub, N., da Conceição Rodrigues, M. A., and Rocha, F.: Can benthic foraminifera be used as bio-indicators of pollution in areas with a wide range of physicochemical variability?, Estuarine, Coast. Shelf Sci., 182, 211–225, https://doi.org/10.1016/j.ecss.2016.10.011, 2016.
Mathers, K. L., Doretto, A., Fenoglio, S., Hill, M. J., and Wood, P. J.: Temporal effects of fine sediment deposition on benthic macroinvertebrate community structure, function and biodiversity likely reflects landscape setting, Sci. Total Environ., 829, 154612, https://doi.org/10.1016/j.scitotenv.2022.154612, 2022.
McCorkle, D. C., Corliss, B. H., and Farnham, C. A.: Vertical distributions and stable isotopic compositions of live (stained) benthic foraminifera from the North Carolina and California continental margins, Deep-Sea Res. Pt. I, 44, 983–1024, https://doi.org/10.1016/S0967-0637(97)00004-6, 1997.
Meslard, F., Bourrin, F., Many, G., and Kerhervé, P.: Suspended particle dynamics and fluxes in an Arctic fjord (Kongsfjorden, Svalbard), Estuar. Coast. Shelf Sci., 204, 212–224, https://doi.org/10.1016/j.ecss.2018.02.020, 2018.
Mestdagh, S., Bagaço, L., Braeckman, U., Ysebaert, T., De Smet, B., Moens, T., and Van Colen, C.: Functional trait responses to sediment deposition reduce macrofauna-mediated ecosystem functioning in an estuarine mudflat, Biogeosciences, 15, 2587–2599, https://doi.org/10.5194/bg-15-2587-2018, 2018.
Metzger, E., Barbe, A., Cesbron, F., Thibault de Chanvalon, A., Jauffrais, T., Jézéquel, D., and Mouret, A.: Two-dimensional ammonium distribution in sediment pore waters using a new colorimetric diffusive equilibration in thin-film technique, Water Res., 2, 100023, https://doi.org/10.1016/j.wroa.2018.100023, 2019.
Mojtahid, M., Griveaud, C., Fontanier, C., Anschutz, P., and Jorissen, F. J.: Live benthic foraminiferal faunas along a bathymetrical transect (140–4800 m) in the Bay of Biscay (NE Atlantic), Rev. Micropaléontol., 53, 139–162, https://doi.org/10.1016/j.revmic.2010.01.002, 2010.
Mojtahid, M., Jorissen, F., Durrieu, J., Galgani, F., Howa, H., Redois, F., and Camps, R.: Benthic foraminifera as bio-indicators of drill cutting disposal in tropical east Atlantic outer shelf environments, Mar. Micropaleontol., 61, 58–75, https://doi.org/10.1016/j.marmicro.2006.05.004, 2006
Mojtahid, M., Zubkov, M. V., Hartmann, M., and Gooday, A. J.: Grazing of intertidal benthic foraminifera on bacteria: Assessment using pulse-chase radiotracing, J. Exp. Mar. Biol. Ecol., 399, 25–34, https://doi.org/10.1016/j.jembe.2011.01.011, 2011.
Morvan, J., Debenay, J.-P., Jorissen, F., Redois, F., Bénéteau, E., Delplancke, M., and Amato, A.-S.: Patchiness and life cycle of intertidal foraminifera: Implication for environmental and paleoenvironmental interpretation, Mar. Micropaleont., 61, 131–154, https://doi.org/10.1016/j.marmicro.2006.05.009, 2006.
Murray, J. W.: Ecology and Applications of Benthic Foraminifera, xi 426 pp. Cambridge, New York, Melbourne: Cambridge University Press, ISBN 9780 521 82839 0, Geological Magazine, 145, 600–601, https://doi.org/10.1017/S0016756808004676, 2006.
Murray, J. W. and Alve, E.: Major aspects of foraminiferal variability (standing crop and biomass) on a monthly scale in an intertidal zone, J. Foramin. Res., 30, 177–191, https://doi.org/10.2113/0300177, 2000.
Nardelli, M. P., Barras, C., Metzger, E., Mouret, A., Filipsson, H. L., Jorissen, F., and Geslin, E.: Experimental evidence for foraminiferal calcification under anoxia, Biogeosciences, 11, 4029–4038, https://doi.org/10.5194/bg-11-4029-2014, 2014.
Nesbitt, E. A., Martin, R. A., Martin, D. E., and Apple, J.: Rapid deterioration of sediment surface habitats in Bellingham Bay, Washington State, as indicated by benthic foraminifera, Mar. Pollut. Bull., 97, 273–284, https://doi.org/10.1016/j.marpolbul.2015.06.006, 2015.
de Nooijer, L. J., Toyofuku, T., and Kitazato, H.: Foraminifera promote calcification by elevating their intracellular pH, P. Natl. Acad. Sci. USA, 106, 15374–15378, https://doi.org/10.1073/pnas.0904306106, 2009.
Norkko, A., Rosenberg, R., Thrush, S. F., and Whitlatch, R. B.: Scale- and intensity-dependent disturbance determines the magnitude of opportunistic response, J. Exp. Mar. Biol. Ecol., 330, 195–207, https://doi.org/10.1016/j.jembe.2005.12.027, 2006.
Papaspyrou, S., Diz, P., García-Robledo, E., Corzo, A., and Jimenez-Arias, J.: Benthic foraminiferal community changes and their relationship to environmental dynamics in intertidal muddy sediments (Bay of Cádiz, SW Spain), Mar. Ecol. Prog. Ser., 490, 121–135, https://doi.org/10.3354/meps10447, 2013.
Pascal, P.-Y., Dupuy, C., Richard, P., Mallet, C., telet, E. A. du C., and Niquilb, N.: Seasonal variation in consumption of benthic bacteria by meio- and macrofauna in an intertidal mudflat, Limnol. Oceanogr., 54, 1048–1059, https://doi.org/10.4319/lo.2009.54.4.1048, 2009.
Pillet, L., de Vargas, C., and Pawlowski, J.: Molecular identification of sequestered diatom chloroplasts and kleptoplastidy in foraminifera, Protist, 162, 394–404, https://doi.org/10.1016/j.protis.2010.10.001, 2011.
Porter, E. T., Owens, M. S., and Cornwell, J. C.: Effect of Sediment Manipulation on the Biogeochemistry of Experimental Sediment Systems, J. Coast. Res., 226, 1539–1551, https://doi.org/10.2112/05-0478, 2006.
Pucci, F., Geslin, E., Barras, C., Morigi, C., Sabbatini, A., Negri, A., and Jorissen, F. J.: Survival of benthic foraminifera under hypoxic conditions: Results of an experimental study using the CellTracker Green method, Mar. Pollut. Bull., 59, 336–351, https://doi.org/10.1016/j.marpolbul.2009.08.015, 2009.
Revsbech, N. P.: An oxygen microsensor with a guard cathode, Limnol. Oceanogr., 34, 474–478, https://doi.org/10.4319/lo.1989.34.2.0474, 1989.
Richirt, J., Riedel, B., Mouret, A., Schweizer, M., Langlet, D., Seitaj, D., Meysman, F. J. R., Slomp, C. P., and Jorissen, F. J.: Foraminiferal community response to seasonal anoxia in Lake Grevelingen (the Netherlands), Biogeosciences, 17, 1415–1435, https://doi.org/10.5194/bg-17-1415-2020, 2020.
Ross, B. J. and Hallock, P.: Challenges in using CellTracker Green on foraminifers that host algal endosymbionts, Peer J, 6, e5304, https://doi.org/10.7717/peerj.5304, 2018.
Sánchez-Bayo, F. and Wyckhuys, K. A. G.: Worldwide decline of the entomofauna: A review of its drivers, Biol. Conserv., 232, 8–27, https://doi.org/10.1016/j.biocon.2019.01.020, 2019.
Schneider, C. A., Rasband, W. S., and Eliceiri, K. W.: NIH Image to ImageJ: 25 years of image analysis, Nat. Methods, 9, 671–675, https://doi.org/10.1038/nmeth.2089, 2012.
Schönfeld, J., Alve, E., Geslin, E., Jorissen, F., Korsun, S., and Spezzaferri, S.: The FOBIMO (FOraminiferal BIo-MOnitoring) initiative – Towards a standardised protocol for soft-bottom benthic foraminiferal monitoring studies, Mar. Micropaleontol., 94, 1–13, https://doi.org/10.1016/j.marmicro.2012.06.001, 2012.
Schumacher, S., Jorissen, F. J., Dissard, D., Larkin, K. E., and Gooday, A. J.: Live (Rose Bengal stained) and dead benthic foraminifera from the oxygen minimum zone of the Pakistan continental margin (Arabian Sea), Mar. Micropaleontol., 62, 45–73, https://doi.org/10.1016/j.marmicro.2006.07.004, 2007.
Seuront, L. and Bouchet, V. M. P.: The devil lies in details: new insights into the behavioural ecology of intertidal foraminifera, J. Foramin. Res., 45, 390–401, https://doi.org/10.2113/gsjfr.45.4.390, 2015.
Severin, K. P. and Erskian, M. G.: Laboratory experiments on the vertical movement of Quinqueloculina impressa Reuss through sand, J. Foramin. Res., 11, 133–136, https://doi.org/10.2113/gsjfr.11.2.133, 1981.
Silverberg, N., Gagnon, J.-M., and Lee, K.: A benthic mesocosm facility for maintaining soft-bottom sediments, Neth. J. Sea Res., 34, 289–302, https://doi.org/10.1016/0077-7579(95)90039-X, 1995.
Stouff, V., Geslin, E., Debenay, J.-P., and Lesourd, M.: Origin of morphological abnormalities in Ammonia (foraminifera): studies in laboratory and natural environments, J. Foramin. Res., 29, 152–170, https://doi.org/10.2113/gsjfr.29.2.152, 1999.
Suokhrie, T., Saraswat, R., and Nigam, R.: Foraminifera as Bio-Indicators of pollution: A review of research over the last decade, Adv. Micropaleontol., 267–286, 2017.
Thibault de Chanvalon, A., Metzger, E., Mouret, A., Cesbron, F., Knoery, J., Rozuel, E., Launeau, P., Nardelli, M. P., Jorissen, F. J., and Geslin, E.: Two-dimensional distribution of living benthic foraminifera in anoxic sediment layers of an estuarine mudflat (Loire estuary, France), Biogeosciences, 12, 6219–6234, https://doi.org/10.5194/bg-12-6219-2015, 2015.
Thrush, S. F., Gray, J. S., Hewitt, J. E., and Ugland, K. I.: Predicting the effects of habitat homogenization on marine biodiversity, Ecol. Appl., 16, 1636–1642, https://doi.org/10.1890/1051-0761(2006)016[1636:PTEOHH]2.0.CO;2, 2006.
Tsujimoto, A., Nomura, R., Arai, K., Nomaki, H., Inoue, M., and Fujikura, K.: Changes in deep-sea benthic foraminiferal fauna caused by turbidites deposited after the 2011 Tohoku-oki earthquake, Mar. Geol., 419, 106045, https://doi.org/10.1016/j.margeo.2019.106045, 2020.
Van der Zwaan, G. J., Duijnstee, I. A. P., den Dulk, M., Ernst, S. R., Jannink, N. T., and Kouwenhoven, T. J.: Benthic foraminifers: proxies or problems?, Earth-Sci. Rev., 46, 213–236, https://doi.org/10.1016/S0012-8252(99)00011-2, 1999.
Whomersley, P., Huxham, M., Schratzberger, M., and Bolam, S.: Differential response of meio- and macrofauna to in situ burial, J. Mar. Biol. Assoc. UK, 89, 1091–1098, https://doi.org/10.1017/S0025315409000344, 2009.
Widerlund, A., Nowell, G. M., Davison, W., and Pearson, D. G.: High-resolution measurements of sulphur isotope variations in sediment pore-waters by laser ablation multicollector inductively coupled plasma mass spectrometry, Chem. Geol., 291, 278–285, https://doi.org/10.1016/j.chemgeo.2011.10.018, 2012.
Włodarska-Kowalczuk, M., Pearson, T., and Kendall, M.: Benthic response to chronic natural physical disturbance by glacial sedimentation in an Arctic fjord, Mar. Ecol. Prog. Ser., 303, 31–41, https://doi.org/10.3354/meps303031, 2005.
Włodarska-Kowalczuk, M., Pawłowska, J., and Zajączkowski, M.: Do foraminifera mirror diversity and distribution patterns of macrobenthic fauna in an Arctic glacial fjord?, Mar. Micropaleontol., 103, 30–39, https://doi.org/10.1016/j.marmicro.2013.07.002, 2013.
Wolanski, E. and Gibbs, R.: Resuspension and clearing of dredge spoils after dredging, Cleveland Bay, Australia, Water Environ. Res., 64, 910–914, https://doi.org/10.2175/WER.64.7.9, 1992.
Wood, P. J.: Biological effects of fine sediment in the lotic environment, Environ. Manage., 21, 203–217, https://doi.org/10.1007/s002679900019, 1997.
Wukovits, J., Oberrauch, M., Enge, A. J., and Heinz, P.: The distinct roles of two intertidal foraminiferal species in phytodetrital carbon and nitrogen fluxes – results from laboratory feeding experiments, Biogeosciences, 15, 6185–6198, https://doi.org/10.5194/bg-15-6185-2018, 2018.
Wukovits, J., Enge, A., Bukenberger, P., Wanek, W., Watzka, M., and Heinz, P.: Phytodetrital quality (C:N ratio) and temperature changes affect C and N cycling of the intertidal mixotrophic foraminifer Haynesina germanica, Aquat. Biol., 30, 119–132, https://doi.org/10.3354/ab00746, 2021.