the Creative Commons Attribution 4.0 License.
the Creative Commons Attribution 4.0 License.
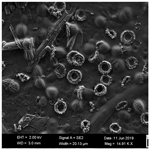
Biomineralization of amorphous Fe-, Mn- and Si-rich mineral phases by cyanobacteria under oxic and alkaline conditions
Karim Benzerara
Agnès Elmaleh
Maria Ciobanu
Alexis De Wever
Paola Bertolino
Miguel Iniesto
Didier Jézéquel
Purificación López-García
Nicolas Menguy
Elodie Muller
Fériel Skouri-Panet
Sufal Swaraj
Rosaluz Tavera
Christophe Thomazo
David Moreira
Iron and manganese are poorly soluble elements in oxic and alkaline solutions, whereas they are much more soluble under anoxic conditions. As a result, the formation of authigenic mineral phases rich in Fe and/or Mn has traditionally been viewed as diagnostic of global or local anoxic conditions. Here we reveal that some specific cyanobacteria of very small size (< 2 µm, i.e., picocyanobacteria) can biomineralize abundant, authigenic Fe(III)-, Mn(IV)- and Si-rich amorphous phases under oxic conditions in an alkaline lake in Mexico. The resulting biominerals cluster as small globules arranged as rings around the division septum of cyanobacterial cells. These rings are enveloped within an organic, likely polysaccharidic envelope and are partially preserved, at least morphologically, upon sedimentation. Based on their 16S rDNA sequence, these cyanobacteria were affiliated with the Synechococcales order. The high Fe and Mn enrichment of the biominerals questions the systematic inference of anoxic conditions based on their detection. Moreover, this process scavenges iron from the water column, an overlooked biological contribution to the Fe cycle. Finally, it reveals a new case of controlled biomineralization of Si-rich phases by bacteria.
- Article
(6509 KB) - Full-text XML
-
Supplement
(26858 KB) - BibTeX
- EndNote
Iron is the fourth-most abundant element in the Earth's crust (Taylor, 1964). It is essential to a broad diversity of organisms, particularly primary producers (Liu et al., 2021). However, Fe is poorly soluble in oxic, neutral to alkaline waters and sometimes becomes a limiting nutrient for the biosphere (Tagliabue et al., 2017). Overall, its bioavailability affects the efficiency of atmospheric carbon uptake in aqueous environments (Aumont and Bopp, 2006). Therefore, efforts have been invested to model the Fe cycle in these environments (Boyd and Ellwood, 2010). Fe enters the cycle through different sources such as eolian dust, detrital particles, release from anoxic sediments or hydrothermal vents (Jickells et al., 2005; Elrod et al., 2004; Tagliabue et al., 2010). Biological activity sets a “ferrous wheel” by mediating the dissolution of particulate Fe and degrading biogenic Fe–organic complexes on the one hand and through Fe cell uptake and complexation by organic ligands on the other hand. Adsorption on various settling mineral particles can scavenge iron out of this wheel. Overall, the balance between removal and release controls the bioavailability of Fe. In any case, since dissolved Fe concentrations remain low under these conditions, no significant authigenesis of Fe-rich mineral phases is expected in an oxic water column. By contrast, soluble reduced Fe(II) was much more abundant in ancient aqueous environments before atmosphere oxygenation, 2.4 billion years ago (Canfield, 2005). At that time, authigenesis of Fe-rich mineral phases was usual, by precipitation of Fe(II) phases and/or local oxidation of Fe(II) in Fe(III), resulting in the massive deposition of sedimentary Fe-rich formations such as banded iron formations (BIFs) (Canfield, 2005). The nature of the authigenic minerals feeding these formations has been widely discussed. Among the possible candidates, Fe(II)-rich silicates such as greenalite have been suggested (Rasmussen et al., 2019). Like iron, reduced Mn(II) is highly soluble, while oxidized Mn (III, IV) precipitates as insoluble oxides under oxic conditions (Johnson, 2019). Consequently, the finding of Fe- and/or Mn-rich authigenic minerals has often been considered to be an indication of past anoxic conditions (Rasmussen et al., 2019; Tosca et al., 2016).
Over the last years, we have studied several crater lakes in Mexico with a main interest for the formation of carbonate microbialites harbored by these lakes (Zeyen et al., 2021). In some of the shore-based microbialites, we found that local seepages of anoxic groundwater could feed the instant precipitation of Fe-containing phases, as either Fe–Mg-layered double hydroxides in Lake Alchichica, where dissolved orthosilicic acid concentrations are low, or Fe-bearing kerolite/stevensite in other lakes, where dissolved H4SiO4 concentrations are higher (Zeyen et al., 2019). To further characterize the Fe and Mn cycles in these lakes, we also systematically examined the particulate fraction in their water column using a combination of approaches at the bulk and the micro- to nano-scale. This produced one fortuitous find based on the examination of the oxic part of the water column of Lake La Preciosa: we evidenced a biological process forming an Fe- and Mn-rich mineral phase under oxic and alkaline conditions, a process of particular interest considering the aforementioned considerations. In the following, we characterize the structure and chemical composition of these biominerals and their association with cell structures, demonstrate their biogenicity, and identify the microorganisms biomineralizing them. This allows the environmental and biological importance of this possibly overlooked biomineralization process to be discussed.
2.1 Field campaign and sample collection
Cyanobacteria-forming Fe- and Mn-rich phases were detected in all field campaigns at Lake La Preciosa at all depths (0 to 35 m) in May 2014, May 2016, March 2018 and May 2019. Temperature, dissolved oxygen concentration, turbidity and pH were measured in situ over a depth profile using a YSI 6600 multiparameter probe and an optode sensor for dissolved oxygen and temperature (SDOT; NKE Instrumentation) in May 2016. The detection limit for dissolved O2 was ca. 0.1 % (0.3 ± 0.2 µM). The oxygen sensor was calibrated against water-vapor-saturated ambient air (100 % O2 local saturation), and the “zero” O2 was checked using a sodium sulfite solution at 10 wt %. The pH sensor was calibrated using three buffers (4, 7 and 10). Lake water was sampled with a Niskin bottle in the center of the lake at different depths from the surface down to 35 m, the maximum depth of Lake La Preciosa. Part of the water was filtered through 0.2 µm polyethersulfone (PES) filters on the same day and kept in sterile tubes for chemical analyses. Some of the filtered solutions were acidified with nitric acid (2 %) for major cation analyses. The other part was used for measurement of anion and orthosilicic acid (H4SiO4) concentrations without pre-acidification. Last, the concentration of the microbial cells was increased 5000 times by filtering 5 L water samples using 0.2 µm pore size cell trap units (MEM-TEQ Ventures Ltd, Wigan, UK).
2.2 Fluorescence-activated cell sorting (FACS) and cell micromanipulation
Cyanobacterial cells were sorted with a BD FACSAria III cell sorter (Becton Dickinson and Company, San Jose, California, USA) equipped with one gas-state 633 nm laser (> 18 mW, elliptical shape) and three solid-state lasers at 488 nm (> 20 mW, elliptical shape), 405 nm (> 50 mW, elliptical shape) and 375 nm (> 7 mW, top-hat shape). The 488 nm laser was used for the analysis of forward scatter (FSC; 488/10, 1.0 ND filter), side scatter (SSC; 488/10), phycoerythrin (PE; 585/42, 556LP) and chlorophyll/phycocyanin (PerCP-Cy5-5; 695/40, 655LP). Light was detected by Hamamatsu R3896 photomultiplier tubes in C10562 sockets (Hamamatsu, 211 Hamamatsu City, Japan). The applied voltages were 220 V (FSC), 324 V (SSC), 703 V (PE) and 577 V (PerCP_Cy5-5). The trigger was set to the FSC with a threshold of 2000. The fluidic system was run at 45 psi (3.102 bar) with an 85 µm nozzle. Samples were sorted at a speed of 6000–6500 events s−1, with a flow rate of 1, corresponding to approximately 10 µL min−1. The sheath fluid consisted of sterile 1X PBS buffer (K2HPO4 0.11 %, KH2PO4 0.03 %, NaCl 0.8 %). In addition to the excitation lasers, an additional BD Accudrop laser of 660 nm was used for the drop delay setup. Lake La Preciosa cell suspensions were filtered on a 35 µm mesh just before sorting to avoid clogs. We used 1 µm UV Fluoresbrite microspheres (24062, Polysciences, Warrington, USA) for calibration of the log range and a pure culture of Synechococcus rubescens as a positive control for phycoerythrin and chlorophyll/phycocyanin fluorescence (Wood et al., 1985). Twenty-five thousand events of one distinct phycoerythrin/chlorophyll-positive population (Pop1; Fig. S6a and b in the Supplement) were sorted directly on 13 mm, 0.4 µm pore size polycarbonate filters (MerckMillipore, Germany) using the most accurate sorting mode “single-cell purity”. The filters were prepared and observed by SEM as described below. For DNA extraction, 20 cells were sorted under the same conditions, directly into a 0.2 mL microtube (Eppendorf), and stored at −20 ∘C. Cytometric data were processed using the BD FACSDIVA V9.0.1 software (Becton, Dickinson and Company, San Jose, California, USA).
We also isolated cyanobacterial cells by micromanipulation from samples collected in March 2018 using an Eppendorf PatchMan NP2 micromanipulator equipped with 6 µm diameter microcapillaries (Eppendorf) mounted on a Leica Dlll3000 B inverted microscope. Individual cells were rinsed twice with sterile 10 mM Tris pH 8.0 buffer. Several sets of 5 to 10 cells were deposited in a volume of 0.4 µL of the same buffer in 0.2 mL tubes (Eppendorf) and stored frozen at −20 ∘C until further processing.
2.3 16S rRNA gene sequencing and phylogenetic analysis
DNA was extracted from the FACS-sorted and the micromanipulated cells with the PicoPure DNA extraction kit (Applied Biosystems). In the case of the micromanipulated cells, whole-genome amplification (WGA) was carried out on the PicoPure-extracted DNA using multiple displacement amplification (MDA) with the REPLI-g WGA kit (Qiagen); 16S rRNA genes were amplified by PCR from the PicoPure-purified and WGA-amplified DNAs using the cyanobacterial-specific primers CYA-106F (5′-CGG ACG GGT GAG TAA CGC GTG A) and CYA-1380R (5′-ACG ACT TCG GGC GTG ACC). PCR amplifications were carried out in a 25 µL reaction volume with a GoTaq polymerase reaction mix (Promega, Lyon, France). PCR reactions consisted of an initial denaturing period (95 ∘C for 3 min) followed by 35 cycles of denaturing (93 ∘C for 45 s), annealing (55 ∘C for 45 s), extension (72 ∘C for 2 min) and a final extension period (72 ∘C for 5 min). Because of the possible presence of more than one species in the samples, we constructed 16S rRNA gene clone libraries with the PCR amplicons using the Topo TA cloning system (Invitrogen) following the instructions provided by the manufacturer. After plating, positive transformants were screened by PCR amplification using the M13R and T7 flanking vector primers. Amplicons were sequenced by Sanger sequencing (Genewiz, Essex, UK).
All forward and reverse Sanger sequences were quality-controlled and merged using CodonCode Aligner (http://www.codoncode.com/aligner/, last access: 22 May 2022, version v11.0.1). Then, we utilized Mafft (Katoh and Standley, 2013) to produce multiple sequence alignments including our sequences and the closest blast (Altschul et al., 1997) hits identified in GenBank. Gaps and ambiguously aligned regions were removed with trimAl (Capella-Gutiérrez et al., 2009). A maximum-likelihood phylogenetic tree was constructed with IQ-TREE (Nguyen et al., 2015) using the general-time-reversible (GTR) model and branch support estimated by ultrafast bootstrapping.
2.4 Sediment collection
A sediment core measuring 10 cm in length was collected from the bottom of Lake La Preciosa at 35 m depth during the field campaign in May 2016 using a gravitational Uwitec corer with a diameter of 90 mm. The sediment core was transferred into a glove bag and placed under anoxic conditions (N2 atmosphere) immediately after collection. It was then processed and sliced in the field into centimeter-scale fractions along the core's vertical axis. Oxygen levels in the glove bag were monitored with a multi 3430 WTW oxygen meter (FDO 925 optode) and were always below the detection limit of 0.1 mg L−1. Back in France, sediments were vacuum-dried in a Jacomex™ glovebox (< 1 ppm O2) before being analyzed by SEM.
2.5 Solution chemistry
Orthosilicic acid (H4SiO4) concentrations were determined by continuous-flow colorimetric analyses (QuAAtro Axflow) at the Institut de Physique du Globe de Paris (IPGP; Paris, France) using non-acidified, 0.2 µm filtered La Preciosa solutions. Anion concentrations were measured using ion chromatography (ICS1100 Dionex Thermo Fisher) using non-acidified, 0.2 µm filtered La Preciosa solutions. Concentrations of major cations were determined by inductively coupled plasma–optical emission spectrometry (ICP–OES; iCAP6200 Thermo Fisher), using acidified, 0.2 µm filtered La Preciosa solutions. The uncertainty in the concentration measurements of orthosilicic acid, anions and cations was lower than 5 %. Activities of anions, cations and orthosilicic acid as well as saturation indices of the surface water solutions of the lakes were calculated using the Visual MINTEQ software.
2.6 Confocal laser scanning microscopy (CLSM) and correlative with scanning electron microscopy (SEM)
For standard CLSM analyses, cells concentrated using cell trap units were deposited on a glass slide, covered by a coverslip and sealed with nail polish. Samples were observed by CLSM using a Zeiss LSM 710. Excitation was performed at 405 and 488 nm, and emission spectra were measured for each pixel of the images over the 405–720 nm wavelength range using a 34-channel Quasar T-PMT detector. Data were processed using the Zeiss Zen software. For correlative CLSM-SEM, cells were first washed with Milli-Q water and then deposited on a coverslip and left to dry. The coverslip was mounted onto a KorrMik Life Sciences sample holder, and the correlative Shuttle and Find software implemented in ZEN 2012 was used. CLSM analyses were conducted first. The same areas were relocated in the SEM with the Shuttle and Find software.
2.7 Scanning electron microscopy (SEM)
Two types of sample preparation were conducted for scanning electron microscopy (SEM) analyses: (i) in one case, cells were sorted by FACS directly on a 13 mm, 0.4 µm pore size polycarbonate membrane (MerckMillipore, Germany) and then washed with 1 mL sterile Milli-Q water and dried at room temperature; (ii) in another case, cell suspensions were vitrified by plunging and freezing in liquid ethane on a gold planchet before being freeze-dried in a Leica EM ACE600 apparatus. Observations were performed using a Zeiss Ultra 55 field emission gun SEM. The acquisition of high-spatial-resolution images was performed at an accelerating voltage of 1 kV and a working distance of ∼ 3 µm with a 20 mm aperture using an annular in-column, in-lens detector for detecting secondary electrons. For the search of Fe- and Mn-rich rings in samples, backscattered electron (BSE) images were acquired using an angle-selective backscattered (AsB) detector at an accelerating voltage of 10 kV and a working distance of ∼ 7.5 mm with a 60 µm aperture at high current. This also allowed cells to be counted over large mosaics (e.g., 730×550 µm), converting numbers into cell density (cells mL−1) based on the ratio of the total filter surface to the surface of the mosaic divided by the volume of filtered water in milliliters. To estimate the error bar, the mosaic was divided into four quadrants to achieve four counting replicates and derive a standard deviation. The elemental composition of mineral phases was determined under the same conditions as BSE imaging by energy-dispersive X-ray spectrometry (EDXS) using an EDS QUANTAX detector (Bruker). EDXS data were analyzed using the ESPRIT software package (Bruker).
2.8 Transmission electron microscopy (TEM), scanning transmission electron microscopy (STEM) and energy-filtered TEM (EFTEM)
Cells concentrated from the water column using cell trap units were washed before being deposited on a formvar-coated TEM grid and air-dried. Fe- and Mn-rich rings were more fastidious to find in sediments because of the high contrast of all mineral particles composing them. Therefore, we enriched samples in rings by a weak acid leaching (step addition of acetic acid keeping pH at ∼ 6). These samples were also deposited on a formvar-coated TEM grid for further analyses.
Observations of unfixed samples were carried out using a Jeol 2100F TEM microscope operating at 200 kV, equipped with a Schottky emitter; a STEM device, which allows Z-contrast imaging in the high-angular annular dark-field (HAADF) mode; a Jeol Si(Li) X-ray detector; and a GIF 2001 Gatan energy filter. Semi-quantitative analyses of EDXS spectra were performed using the JEOL Analysis Station software. This was based on the use of k factors. After subtracting out the background noise in the EDXS spectrum, the software performed a Gaussian fit of selected elemental peaks and calculated the area under the peaks. From this, the atomic percentage of each selected element was assessed. EFTEM elemental mapping of C, Fe and Mn was performed using the three-window technique (Hofer et al., 1997). This technique requires three energy-filtered images: two positioned before the ionization edge (pre-edge images), which serve to calculate the background, and one positioned just after the edge (post-edge image). A calculated background image was subtracted from the post-edge image to give an elemental map, in which changes in background shape were considered. Maps were calculated for the C K edge and Fe and Mn L2,3 edges using a 20 eV wide (for C) or 40 eV wide (for Fe and Mn) energy window for pre-edge and post-edge. Zero-loss images were obtained by selecting elastically scattered electrons only, using a 10 eV wide energy window.
2.9 Scanning transmission X-ray microscopy (STXM)
Cells suspended in the water column and sediment samples were prepared in the same way as for TEM and deposited on TEM grids. Scanning-transmission-X-ray-microscopy analyses were performed on the HERMES beamline at the SOLEIL synchrotron (St. Aubin, France). This microscopy uses monochromated X-rays in the soft X-ray energy domain (200–2000 eV) which are focused onto a ∼25 nm spot by a Fresnel zone plate. This approach provides images with a ∼25 nm spatial resolution and spatially resolved speciation information based on X-ray absorption near-edge structure (XANES) spectroscopy. An image is obtained by positioning the sample at the focal point of the lens and raster-scanning it in the x and y directions while recording the intensity of the transmitted X-rays. Some cells with rings were pre-located by short TEM imaging conducted prior to STXM measurements in order to facilitate the analyses. However, in order to assess potential electron beam damage, we also analyzed by STXM cells in areas of the TEM grids kept pristine with no prior TEM analyses. A stack of STXM images of the areas of interest were acquired at a sequence of photon energies at the C K edge first. The spectral resolution was 0.12 eV in the 282–291.5 eV energy range, where most of the narrowest XANES peaks were present. Then, stacks were acquired at the Mn L2,3 edges (spectral resolution of 0.1 eV in the 642–650 and 653.75–662 eV ranges) before the Fe L2,3 edges (spectral resolution of 0.15 eV in the 709–721 eV range). Areas free of particles were used to measure the incident flux (I0). Images were converted from transmitted intensity units to optical density units (OD) following the formula OD = . XANES spectra were extracted and mapped using the aXis2000 software (McMaster University, http://unicorn.mcmaster.ca/axis/aXis2000-IDLVM.html).
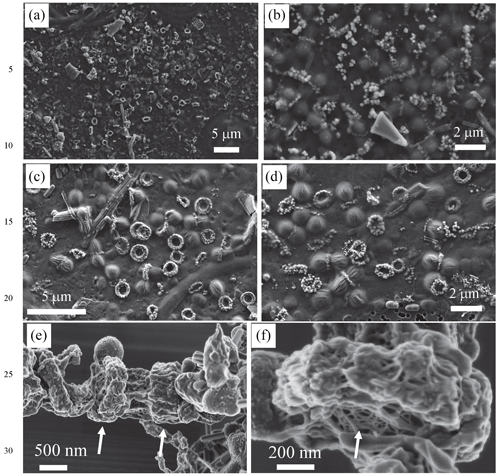
Figure 1Scanning electron microscopy imaging of Fe-, Mn- and Si-rich rings formed by dividing cells. (a, b) SEM images obtained with the secondary-electron detector of an air-dried sample collected in 2018 (acceleration voltage: 5 keV; working distance: 7 mm). (c, d) SEM images obtained with the secondary-electron detector of air-dried samples collected in 2019 (acceleration voltage: 2 keV; working distance: 3 mm). (e, f) SEM images obtained with the in-lens detector of freeze-dried samples collected in 2018. Arrows in (e) show some rings. Arrow in (f) shows the mesh texture of the polysaccharidic envelope of the rings.
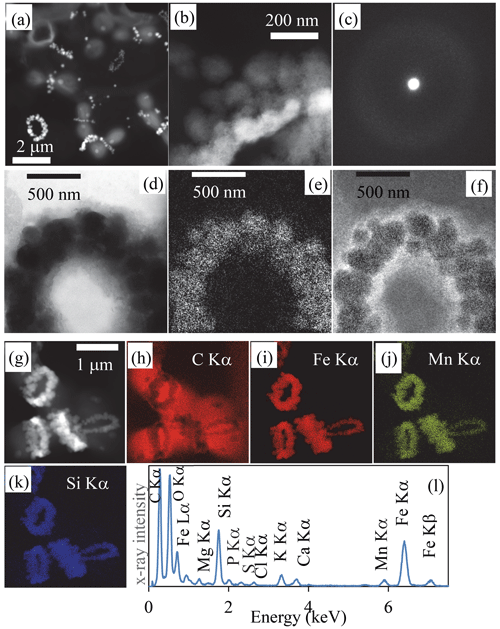
Figure 2Transmission-electron-microscopy analyses of bacterial Fe-, Mn- and Si)-rich rings. (a) Scanning-transmission-electron-microscopy image in high-angular annular dark field (STEM-HAADF) mode of dividing cells collected in 2019. Rings which are composed of high-atomic-number elements appear bright. (b) STEM-HAADF image at higher magnification of a ring collected in 2018, showing that it is composed of globules which themselves show a fibrous texture. (c) Selected area of electron diffraction pattern of a ring, characteristic of an amorphous material. (d) Zero-loss energy-filtered transmission-electron-microscopy (EFTEM) image of a ring collected in 2018. (e) EFTEM image at the Fe L2,3 edges. (f) EFTEM image at the C K edge. (g) STEM-HAADF image of cells with rings chemically mapped using energy-dispersive X-ray spectrometry (EDXS). (h) Chemical map of carbon based on the C Kα emission line. (i) Chemical map of iron based on the Fe Kα emission line. (j) Chemical map of manganese based on the Mn Kα emission line. (k) Chemical map of silicon based on the Si Kα emission line. (l) EDXS spectrum of the rings observed in the chemical maps.
3.1 A new biomineralization process of amorphous Fe-, Mn- and Si-rich phases
We sampled water at several depths in the Mexican crater Lake La Preciosa, up to ∼ 35 m below water level, and examined the plankton (> 0.2 µm). Among the broad morphological diversity of microorganisms observed by light microscopy, one frequent morphotype appeared as doublets of coccoid cells measuring ∼ 1 µm in diameter each and forming loose aggregates of up to ∼ 50 cells (Fig. 1 in the Supplement). Their abundance was estimated to be about 2.3 (± 0.3) × 105 cells mL−1. An opaque band systematically separated each doublet of dividing cells. This trait morphologically resembles that of previously described members of the Cyanocatena genus (Hindák, 1982). With electron microscopy, these bands appeared as bright rings with a diameter of 1 µm (Figs. 1 and 2, Fig. 2 in the Supplement). They localized at the division septum of cells and were sometimes observed in planar view when detached from dividing cells (Figs. 1b–d and 2a). Overall, these rings appear as traces of septation events. In some cases, we observed single rings oriented perpendicularly in between two pairs of dividing cells (Fig. 1 in the Supplement). This pattern suggests that cells divided along two consecutive perpendicular planes. By contrast, in rare cases, we observed a series of aligned cells with rings perpendicular to the alignment, suggesting a single division direction only.
At higher magnification, the rings appeared as aggregates of globules measuring ∼ 50–100 nm in diameter (Fig. 2b, Fig. 2 in the Supplement) and presenting a nanoporous, sometimes fibrous texture (Fig. 2t and v in the Supplement). The rings mostly contained Fe (∼ 33.6 mol % on average) and Si (∼ 35.9 mol % on average), with some variable amounts of Mn (average of 6.5 mol %) with lower amounts of Mg, Ca and K, as shown by energy-dispersive X-ray spectrometry (EDXS) (Table SI-1, Fig. 2g–l, Fig. 2 in the Supplement). They systematically had a low Al content (1.02 ± 1.38 mol %, 25 measurements; Table SI-1). The ratio was estimated to be 1.5 ± 0.55 on average and varies between 0.8 and 2 (25 measurements). Electron diffraction revealed that the grains comprising the rings were amorphous (Fig. 2c). No Fe- and/or Mn-rich intracellular deposits were detected within the cells. The redox state of Mn and Fe in the rings was determined by X-ray absorption near-edge structure (XANES) spectroscopy using scanning transmission X-ray microscopy (STXM). Analyses at the Fe and Mn L2,3 edges of pristine rings unirradiated by TEM beforehand showed that Fe and Mn composing the rings were oxidized, i.e., in the Fe(III) and Mn(IV) redox states (Fig. 3 in the Supplement). By contrast, Mn-comprising rings irradiated by TEM at low magnification beforehand was systematically reduced. This demonstrates that TEM induces artifactual reduction in Mn in these phases.
3.2 Bacteria biomineralizing Fe- and Mn-rich rings are cyanobacteria phylogenetically close to Cyanobium
The coccoid cells forming the Fe-, Mn- and Si-rich rings showed strong autofluorescence by confocal laser scanning microscopy (CLSM), with two maximum emission peaks at ∼ 585 and 670 nm (Fig. 4 in the Supplement). Correlation of CLSM with SEM confirmed that these autofluorescent cells were the ones forming Fe-, Mn- and Si-rich rings (Fig. 5 in the Supplement). Based on Wood et al. (1985) and a direct comparison with the autofluorescence of reference-phycoerythrin-containing Synechococcus rubescens cells, these two peaks were interpreted as resulting from the fluorescence of phycoerythrin and chlorophyll and/or phycocyanin, respectively. This indicated that cells forming Fe- and Mn-rich rings are cyanobacteria.
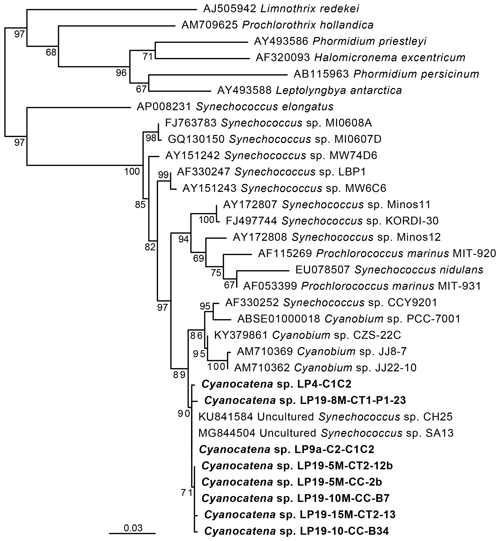
Figure 3Maximum-likelihood phylogenetic tree of cyanobacterial 16S rRNA gene sequences showing the position of the cells carrying Fe and Mn rings. Numbers on branches are bootstrap proportions. The bolded sequences are from this study, derived from the cells sorted by flow cytometry and forming rings, and they were named Cyanocatena, as explained in Sect. 4.2.
These phycoerythrin-containing cyanobacteria were efficiently enriched by fluorescence-activated cell sorting (FACS) based on the autofluorescence properties of their particular pigments (Fig. 6a and b in the Supplement). DNA was extracted from the enriched cell fractions, and, after PCR amplification, their 16S rRNA genes were sequenced. In parallel, micromanipulated cells were also used for 16S rRNA gene sequencing after whole-genome amplification (WGA). Maximum-likelihood phylogenetic tree reconstruction confirmed that these cells were cyanobacteria. More specifically, their sequences branched within a group containing sequences classified as Synechococcus and Cyanobium (Fig. 3). The small differences between La Preciosa sequences (< 1 %) suggested a population of very closely related cyanobacterial strains producing Fe- and Mn-rich rings in this lake.
These 16S rRNA gene sequences were used to screen a large dataset of 16S rRNA gene metabarcoding sequences of plankton from 11 Mexican lakes, including La Preciosa (Zeyen et al., 2021; Iniesto et al., 2022). We detected sequences closely related (> 99 % identity) to those of the Fe-, Mn- and Si-ring-producing cyanobacteria in 9 of these 11 lakes: La Preciosa, Alchichica, Atexcac, Aljojuca, Alberca de los Espinos, Pátzcuaro, Quechulac, Tecuitlapa and Yuriria (Table 2 in the Supplement). These sequences were particularly abundant (representing > 1 % of all bacterial metabarcoding reads) in La Preciosa, Alchichica, Atexcac, Aljojuca and Alberca de los Espinos, sometimes reaching frequencies up to 15 %. Interestingly, these lakes were characterized by relatively high salinity and alkalinity values (Zeyen et al., 2021), whereas those where the sequences were completely absent had the lowest values for these parameters.
3.3 Fe- and Mn-rich rings form under oxygenated conditions and within a polysaccharidic extracellular compartment
Although we have not experimentally determined the speciation of Fe in the La Preciosa water column, we can infer that the concentration of dissolved free Fe is likely below 1 nM (Liu and Millero, 2002) based on the high pH (between 8.8 and 9.0) and oxic conditions (> 25 % of local O2 water saturation), in particular in the upper part of the water column (Fig. 7 in the Supplement). The rest of Fe in the water column of Lake La Preciosa may be (i) colloidal, inorganic, or organic (complexed by dissolved organic molecules) Fe, in the < 0.2 µm dissolved fraction, or (ii) particulate Fe complexed by microbial cells and/or adsorbed/co-precipitated in inorganic particles. Measured dissolved Fe concentrations (i.e., free and colloidal Fe) were always below the detection limit of ICP-AES (inductively coupled plasma–atomic emission spectroscopy; 0.02 nM), except at a depth of 5 m in 2019, when Fe and Mn were anomalously high, reaching 0.1 and 1 µM, respectively. For that date, dissolved Mn concentration was below the detection limit as well at other depths. Here, Fe and Mn were most likely under a colloidal form, suggesting that the size of the colloidal pool varies over time.
Dissolved Si concentrations were measured and found to be relatively high in Lake La Preciosa (5 × 10−4 M). Moreover, as observed in several other alkaline Mexican lakes (Zeyen et al., 2021), Lake La Preciosa solutions were oversaturated with amorphous sepiolite (Wollast et al., 1968), a hydrated Mg-silicate phase, at all depths and all times (Fig. 7 in the Supplement).
Lastly, chemical conditions prevailing locally where the rings form may be different from the ones in the lake. Indeed, we found evidence of an extracellular compartment enclosing the rings. First, SEM observations at low electron voltage of lyophilized samples showed that rings were embedded in a fibrillar matrix, with a mesh texture reminiscent of the extracellular polymer substances (EPSs) excreted by some bacteria (Fig. 1e and f). Second, energy-filtered TEM (EFTEM) imaging showed that the globular grains were contained within a carbon-rich envelope (Fig. 8 in the Supplement). Finally, the detection of a carboxylic-rich polymer using XANES spectroscopy at the C K edge confirmed the polysaccharidic composition of this carbonaceous envelope, based on the presence of a major absorption peak at 288.6 eV, which is interpreted as electron transition in carboxylic C and has often been used as a spectroscopic marker for acidic polysaccharides (Benzerara et al., 2004) (Fig. 8 in the Supplement).
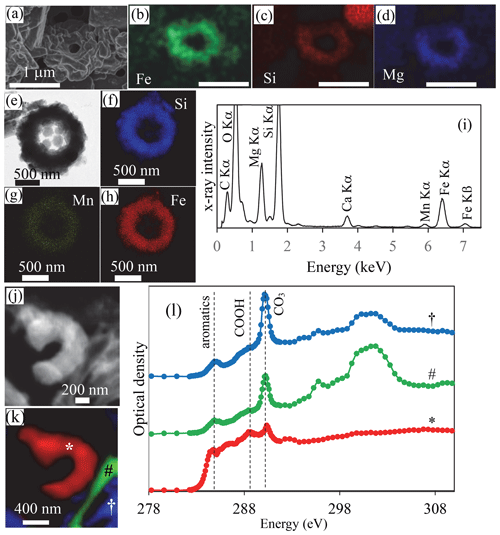
Figure 4SEM, TEM and STXM analyses of cyanobacterial Fe and Mn rings in Lake La Preciosa sediments. (a–d) SEM image in the secondary-electron detection mode and EDXS chemical maps of Fe, Si and Mg of a ring in pristine (non-acid-leached) sediments collected in 2016 at a 3 cm depth in the sediment core. Note that the surface of the rings seems to be covered by a smooth and folded layer in SE mode, suggesting that they are covered by organics. (e) Bright-field STEM image of a ring in acid-leached sediments. (f–h) Corresponding STEM-EDXS chemical maps of Si, Mn and Fe. (i) STEM-EDXS spectrum measured on the ring. (j) Carbon map obtained by subtracting an OD-converted STXM image acquired at 282 eV from an OD-converted image at 288.5 eV. (k, l) Spectral analysis at the C K edge of the image shown in (j). (k) Map of three carbon-containing compounds. Carbon associated with rings is marked with a ∗; carbon typically in a carbonate grain is marked with a #; carbon in a carbonate functional group but with a different crystallographic orientation is marked with a †. (l) Corresponding spectra at the C K edge (see symbols above the spectra). Vertical dashed lines are at 284.8 eV (attributed to aromatic functional groups), 288.5 eV (attributed to carboxylic COOH functional groups) and 290.3 eV (attributed to carbonate CO3 groups). The ring analyzed by STXM was recovered from an acid-leached sediment sample at 1 cm depth in the sediment core (see Fig. 11 in the Supplement for TEM analyses).
3.4 Biomineralized cyanobacterial rings are morphologically preserved upon sedimentation
The sediments were mostly composed of aragonite, anorthite and diatomaceous amorphous silica (Fig. 9 in the Supplement). Moreover, in the bulk Fourier transform infrared spectroscopy spectra of the sediments, bands at 473, 540, 1026, 3625 and 3680 cm−1 could be affiliated with clay-like phases, a proportion of which possibly corresponded to the rings. Although they could not be unambiguously recognized by bulk analyses, cyanobacterial rings with a well-preserved, sometimes partly broken morphology were abundant enough so that they could be observed by electron microscopy in sediment samples with no prior chemical treatment (Fig. 4, Figs. 10–12 in the Supplement). TEM-EDXS showed that, similar to rings in the water column, they had a relatively high Fe content ( 0.36 atoms per atom vs. ∼ 1 atoms per atom in the rings observed in the water column). However, sediment rings had a different chemical composition compared to those found in the water column, with a relatively higher Mg content (∼ 19 mol % in sediments vs. 3.8 mol % on average in the water column) and a lower Mn content (∼ 1.5 mol % vs. 6.5 mol % in the water column). We note that this statement is based on few analyzed sediment rings only and will need further support from additional analyses, with particular attention to some potential progressive transformation within the water column and with depth in the sediments. The surface appearance of some rings suggested that they were contained within an organic envelope (Fig. 4a, Fig. 10a in the Supplement), which was further supported by STXM analyses at the C K edge on acetic-acid-leached samples (Fig. 4l). Here, the spectral signature of carbon was different from that measured on water column rings. However, this may have resulted from artifactual damage induced by the acid leaching of the samples, TEM pre-screening and/or transformation of the organics upon sedimentation. Overall, bulk chemical analyses of the sediments showed that Fe2O3 represented up to 2 % and MnO 0.1 % of the total sediment mass (including ignition loss). This provides some maximum estimate of the Fe- and/or Mn-containing ring abundance.
4.1 Biological and environmental significance of this biomineralization process
Our findings show that some picocyanobacteria biomineralize Fe-, Si- and Mn-rich phases in oxic and alkaline water. The intensity and environmental distribution of this biomineralization process remain unknown. Several groups of picocyanobacteria described using classical approaches seem to form Fe-rich precipitates as well, with diverse textural patterns (Hindák, 2002). For example, Cyanodictyon imperfectum, detected in several lakes worldwide, forms rings composed of iron oxides at its division septum (Cronberg and Weibull, 1981; Economou-Amilli and Spartinou, 1991). Cyanocatena planctonica has also been described to form rings composed of iron oxides at the division septum (Hindák, 1982). All these cells divide along one division plane only, a taxonomic feature different from most Lake La Preciosa cyanobacteria. Hindák (1982) described another cyanobacterium, Cyanogranis ferruginea, which divides along two planes and forms iron oxide rings but not at the cell septa, in contrast with the cells in Lake La Preciosa. No Mn or Si enrichment was reported by any of these studies, but mineralogical analyses were too limited to be conclusive. One difficulty in inferring phylogenetic affiliations is that sequence data for all these cyanobacterial morphotypes are not available. Although the morphological features of picocyanobacteria alone cannot be used to reliably discriminate between different taxa (Callieri et al., 2012), the morphotype observed in La Preciosa resembles Cyanocatena cells (Hindák, 1982), at least regarding their biomineralization pattern. Therefore, we propose to ascribe our new 16S rDNA sequence to this genus as Cyanocatena sp. The taxonomic affiliation of this sequence allows the investigation of the potential prevalence of this unusual biomineralization process in different lakes by the analysis of massive metabarcoding datasets. While the enrichment in Fe and Mn is moderate in Lake La Preciosa sediments, higher sedimentary enrichments can be discovered using such an approach.
Although we ignore the molecular mechanisms of this biomineralization process (see Sect. 4.2), several potential functions can be speculated for this trait. (i) High amounts of Fe and Mn are needed by cyanobacteria compared with other microorganisms (Raven et al., 1999; Nelson and Junge, 2015), but, at the same time, elevated intracellular concentrations of these elements may enhance oxidative stress, therefore requiring some appropriate homeostasis (Liu et al., 2021; Kranzler et al., 2013). Different systems are involved in bacterial intracellular storage of Fe, including different types of ferritin family proteins (Keren et al., 2004). The sequestration of high amounts of Fe and Mn by extracellular amorphous phases may contribute to homeostasis and provide a larger but less toxic Fe and Mn reserve (Brown et al., 2005; Cosmidis and Benzerara, 2022). (ii) Alternatively, Lingappa et al. (2021) showed high intracellular accumulation of Mn in some cyanobacteria and suggested that it could be used as a catalytic antioxidant, useful in environments with high oxidative stress. Here Mn may instead be accumulated outside the cells and serve similar purposes. (iii) The oxidation of Fe and Mn upon extracellular release may also generate protons which could be used to generate a proton gradient and gain energy, a mechanism suggested for Fe-oxidizing bacteria (Chan et al., 2004). (iv) Last, the sequestration of high amounts of Fe and/or Mn in these biominerals may be a way to divert these elements from other organisms and therefore serves competition purposes, as suggested for the production of siderophores by some cyanobacteria in the case of iron stress (Wilhelm, 1995).
4.2 A new controlled biomineralization process concentrating Fe and Mn under oxic conditions
The chemical composition of the biomineralized rings (Al-free and a ( ratio between 0.8 and 2) is reminiscent of the composition of various phyllosilicate phases such as smectites (ferrosaponite: 0.75) and/or serpentines (greenalite or cronstedtite: to 4). Alternatively, the lowest measured values may correspond to Fe-bearing talcs (), consistent with phases detected in La Preciosa sediments and Mexican microbialites (Zeyen et al., 2019) or a mixture of silica-sorbing amorphous Fe and Mn oxyhydroxides. The exact nature of the local structure of this phase could be determined in the future using techniques such as extended X-ray absorption fine structure (EXAFS) spectroscopy at, for example, the Si and Fe K edges, provided that the rings can be separated from other Fe, Mn and Si phases in the samples. In any case, considering its chemical composition, this biomineralized phase may serve as a precursor phase to silicates. The initial phase may consist of mostly Fe(II) and Mn(II) silicates that subsequently oxidized, since decades of experimental syntheses have consistently shown that the synthesis of Fe(III) smectites was achieved by starting from an Fe(II)-silicate gel (Baron et al., 2016). Alternatively, the amorphous Fe-, Mn- and Si-rich phase may form by the release of Fe(II) and Mn(II) at the septum of the bacterial cells, which would spontaneously oxidize in the orthosilicate-rich water of Lake La Preciosa. This scenario is consistent with the formation of Fe–Si complexes with an ratio between 1 and 2 observed in several experimental studies (Pokrovski et al., 2003; Doelsch et al., 2001). Here, the high Si content of the rings may just be related to the Si-rich environmental conditions and not the result of bacterial Si cycling. Several studies have documented the extracellular neoformation of diverse clay-like phases by bacteria (Konhauser and Urrutia, 1999; del Buey et al., 2021). They do so by binding anions (e.g., ) to positively charged molecular sites on the cell surface or EPSs and by ion bridging with cations bound to negatively charged functional groups and/or by increasing the local pH due to their metabolic activity (Zeyen et al., 2015). Here, while these mechanisms may also occur owing to the EPS envelope surrounding the rings and the possible pH increase due to oxygenic photosynthesis, which favors the precipitation of hydroxides and silicates (e.g., Zeyen et al., 2015), the control on biomineralization by this specific bacterium appears much tighter. First, this biomineralization process is not seen in other cyanobacteria populating Lake La Preciosa and therefore is likely mediated by some biological mechanisms specific to these cyanobacteria. Moreover, (i) the biominerals have a specific ring morphology, with a definite diameter of ∼ 1 µm; (ii) they are associated with the cell division plane; (iii) they are enclosed within an extracellular EPS compartment that might be involved in mineral precipitation and control the unusual textural organization of the Fe, Mn and Si mineral phase as a ring; and (iv) they are rich in Fe and Mn, whereas the concentration of free Fe under these environmental conditions does not allow for abiotic authigenesis of Fe-rich phases. This shows that these cyanobacteria manage to efficiently concentrate Fe and Mn locally from the water column, thus likely involving some energy cost. At this stage, the specific mechanisms involved in this process can only be speculated. Fe complexed by organic molecules is usually the most bioavailable form for cyanobacteria (Beghoura et al., 2019). Alternatively, Fe contained in colloids and particles may be released into the water column through biologically and photochemically mediated dissolution (Baker and Croot, 2010). The uptake of Fe from one or several of these sources might be achieved based on processes similar to those evidenced in many other cyanobacteria, such as reductive uptake (Kranzler et al., 2014) and/or siderophore-mediated Fe(III) uptake (Swanner et al., 2015), but possibly with higher efficiency by these cyanobacteria. Mn is usually taken up in its reduced Mn(II) state through the same transporters as Fe (Qiu et al., 2021). Moreover, some studies have reported significant Mn stores within some cyanobacterial cells (Lingappa et al., 2021). The possibility that Mn in the rings is derived from such an internal store should be investigated. However, here, no intracellular precipitate rich in Mn and/or Fe was detected within the cyanobacteria, suggesting that Fe(II) and Mn(II) are rapidly released after uptake at the division septum where they precipitate to form rings. Whether Fe(II) and/or Mn(II) may be specifically released during cell division remains to be investigated. Moreover, additional lines of inquiry are to determine (i) whether proton uptake by the cells could occur locally at the division septum, locally increasing pH and favoring the precipitation of these phases, and (ii) how the EPS envelope around the rings form or preform before the precipitation of the Fe- and Mn-rich rings. The isolation of this bacterium in cultures and/or the analysis of its genome should help in the future to answer these different questions.
Whatever its mechanism and function, the biomineralization of Fe- and Mn-rich rings appears to be a still overlooked Fe-scavenging process, which, together with the already-known Fe-scavenging formation of lithogenic inorganic particles, removes Fe from the water column. Interestingly, Fe–Mn nodules and crusts on the seafloor are present under oxic conditions too, and their formation may involve microorganisms as well (Hein and Koschinsky, 2014). However, nodules have been suggested to be diagenetic in origin, involving microbially catalyzed oxidation of Mn sourced by reduced fluids, whereas the formation of the rings observed in the present study do not likely require the input of reduced Fe(II) and Mn(II) to the bacteria. Cyanobacteria have been considered to be actors in the formation of some iron deposits by triggering the oxidation of dissolved Fe(II) from anoxic fluids and therefore inducing the precipitation of Fe-rich mineral phases (Crowe et al., 2008; Emerson and Moyer, 2002). The sheaths of some cyanobacteria can also serve as a precipitated iron repository in iron-depositing hot springs (Brown et al., 2005). Here, we evidence an additional mechanism by which cyanobacteria may contribute to the formation of Fe-rich phases, involving Fe scavenging and directed nucleation and growth of Fe precipitates within an organic matrix. The resulting biominerals show diagnostic features, which may help in the search for them in modern and ancient samples, following the example of the recent discovery of purported cyanobacteria microfossils with intracellular Fe-silicate nanocrystals in the 1.88 Ga Gunflint Iron Formation (Lepot et al., 2017). The embedding of Fe-rich rings within EPS in La Preciosa may enhance their preservation, and, concomitantly, the formation of these organominerals may favor the preservation of organic matter upon time, as shown by Keil et al. (1994). Consistently, and despite some chemical transformations suggested by the differences in chemical compositions between sediment and water column rings, at least some of these rings withstand dissolution and remain morphologically preserved in Lake La Preciosa sediments, therefore having some potential for fossilization over longer time periods. Overall, the existence of such a biomineralization process questions the systematic inference of anoxic conditions based on the detection of such Fe- and Mn-rich phases in the sedimentary record.
Data are available upon request.
The supplement related to this article is available online at: https://doi.org/10.5194/bg-20-4183-2023-supplement.
KB, AE, MC, PLG, RT, CT and DM designed the study. KB, AdW, PB, MI, DJ, PLG, RT, CT and DM collected the samples in the field. KB, AE, MC, AdW, PB, MI, DJ, PLG, NM, EM, FSP, SS, RT and DM carried out the measurements. KB, AE, MC, ADW, MI, NM, EM and DM analyzed the data. All authors wrote the manuscript.
The contact author has declared that none of the authors has any competing interests.
Publisher's note: Copernicus Publications remains neutral with regard to jurisdictional claims in published maps and institutional affiliations.
We thank Lucie Franco and Michael Bourges for helping with FACS experiments; Nina Zeyen, Mélanie Poinsot, Jena Johnson and Daniel Nothaft for help in the field; Franck Bourdelle for help with STXM experiments; and Mickael Trichet for help with lyophilization. The authors acknowledge SOLEIL for the provision of beam time. Last, we would also like to thank several instrumentation facilities at IMPMC that enabled streamline measurement and analysis of datasets presented in this work. We extend our gratitude to Maxime Guillaumet and Keevin Béneut for the spectroscopy platform; Imène Esteve, Stéphanie Delbrel and Béatrice Doisneau for SEM support; Ludovic Delbes and Benoît Baptiste for X-ray diffraction facility; and Jean Michel Guignier for the TEM platform.
This research has been supported by the European Research Council FP7 Ideas: European Research Council (grant nos. 307110 and 787904), the Centre National de la Recherche Scientifique (“SAMBA” project, Interrvie program) and the Agence Nationale de la Recherche (grant no. ANR-18-CE02-0013-02).
This paper was edited by Jack Middelburg and reviewed by two anonymous referees.
Altschul, S. F., Madden, T. L., Schäffer, A. A., Zhang, J., Zhang, Z., Miller, W., and Lipman, D. J.: Gapped BLAST and PSI-BLAST: a new generation of protein database search programs, Nucleic Acids Res., 25, 3389–3402, https://doi.org/10.1093/nar/25.17.3389, 1997.
Aumont, O. and Bopp, L.: Globalizing results from ocean in situ iron fertilization studies, Global Biogeochem. Cy., 20, GB2017, https://doi.org/10.1029/2005GB002591, 2006.
Baker, A. R. and Croot, P. L.: Atmospheric and marine controls on aerosol iron solubility in seawater, Mar. Chem., 120, 4–13, https://doi.org/10.1016/j.marchem.2008.09.003, 2010.
Baron, F., Petit, S., Tertre, E., and Decarreau, A.: Influence of aqueous Si and Fe speciation on tetrahedral Fe(III) substitutions in nontronites: A clay synthesis approach, Clay. Clay Miner., 64, 230–244, https://doi.org/10.1346/CCMN.2016.0640309, 2016.
Beghoura, H., Gorgues, T., Aumont, O., Planquette, H. f., Tagliabue, A., and Auger, P.-A.: Impact of inorganic particles of sedimentary origin on global dissolved iron and phytoplankton distribution, J. Geophys. Res.-Oceans, 124, 8626–8646, https://doi.org/10.1029/2019JC015119, 2019.
Benzerara, K., Yoon, T. H., Tyliszczak, T., Constantz, B., Spormann, A. M., and Brown Jr, G. E.: Scanning transmission X-ray microscopy study of microbial calcification, Geobiology, 2, 249–259, https://doi.org/10.1111/j.1472-4677.2004.00039.x, 2004.
Boyd, P. W. and Ellwood, M. J.: The biogeochemical cycle of iron in the ocean, Nat. Geosci., 3, 675–682, https://doi.org/10.1038/ngeo964, 2010.
Brown, I. I., Mummey, D., and Cooksey, K. E.: A novel cyanobacterium exhibiting an elevated tolerance for iron, FEMS Microbiol. Ecol., 52, 307–314, https://doi.org/10.1016/j.femsec.2004.11.020, 2005.
Callieri, C., Cronberg, G., and Stockner, J. G.: Freshwater picocyanobacteria: single cells, microcolonies and colonial forms, in: Ecology of Cyanobacteria II: Their Diversity in Space and Time, edited by: Whitton, B. A., Springer Netherlands, Dordrecht, 229–269, https://doi.org/10.1007/978-94-007-3855-3_8, 2012.
Canfield, D. E.: The early history of atmospheric oxygen: Homage to Robert M. Garrels, Annu. Rev. Earth Pl. Sc., 33, 1–36, https://doi.org/10.1146/annurev.earth.33.092203.122711, 2005.
Capella-Gutiérrez, S., Silla-Martínez, J. M., and Gabaldón, T.: trimAl: a tool for automated alignment trimming in large-scale phylogenetic analyses, Bioinformatics, 25, 1972–1973, https://doi.org/10.1093/bioinformatics/btp348, 2009.
Chan, C. S., De Stasio, G., Welch, S. A., Girasole, M., Frazer, B. H., Nesterova, M. V., Fakra, S., and Banfield, J. F.: Microbial polysaccharides template assembly of nanocrystal fibers, Science, 303, 1656–1658, https://doi.org/10.1126/science.1092098, 2004.
Cosmidis, J. and Benzerara, K.: Why do microbes make minerals?, C. R. Geosci., 354, 1–39, https://doi.org/10.5802/crgeos.107, 2022.
Cronberg, G. and Weibull, C.: Cyanodictyon imperfectum, a new chroococcal blue-green alga from Lake Trummen, Sweden, Algological Studies/Archiv für Hydrobiologie, Supplement Volumes, 27, 101–110, https://doi.org/10.1127/algol_stud/27/1981/101, 1981.
Crowe, S. A., O'Neill, A. H., Katsev, S., Hehanussa, P., Haffner, G. D., Sundby, B., Mucci, A., and Fowle, D. A.: The biogeochemistry of tropical lakes: A case study from Lake Matano, Indonesia, Limnol. Oceanogr., 53, 319–331, https://doi.org/10.4319/lo.2008.53.1.0319, 2008.
del Buey, P., Sanz-Montero, M. E., Braissant, O., Cabestrero, O., and Visscher, P. T.: The role of microbial extracellular polymeric substances on formation of sulfate minerals and fibrous Mg-clays, Chem. Geol., 581, 120403, https://doi.org/10.1016/j.chemgeo.2021.120403, 2021.
Doelsch, E., Stone, W. E. E., Petit, S., Masion, A., Rose, J., Bottero, J.-Y., and Nahon, D.: Speciation and crystal chemistry of Fe(III) chloride hydrolyzed in the presence of SiO4 ligands. 2. Characterization of Si−Fe aggregates by FTIR and 29Si solid-state NMR, Langmuir, 17, 1399–1405, https://doi.org/10.1021/la0013188, 2001.
Economou-Amilli, A. and Spartinou, M.: The diversity of Cyanodictyon imperfectum (Chroococcales, Cyanophyceae) in Lake Amvrakia, Greece, Algological Studies/Archiv für Hydrobiologie, Supplement Volumes, 64, 105–114, 1991.
Elrod, V. A., Berelson, W. M., Coale, K. H., and Johnson, K. S.: The flux of iron from continental shelf sediments: A missing source for global budgets, Geophys. Res. Lett., 31, L12307, https://doi.org/10.1029/2004GL020216, 2004.
Emerson, D. and Moyer, C. L.: Neutrophilic Fe-oxidizing bacteria are abundant at the Loihi Seamount hydrothermal vents and play a major role in Fe oxide deposition, Appl. Environ. Microb., 68, 3085–3093, https://doi.org/10.1128/AEM.68.6.3085-3093.2002, 2002.
Hein, J. R. and Koschinsky, A.: 13.11 – Deep-Ocean Ferromanganese Crusts and Nodules, in: Treatise on Geochemistry (Second Edition), edited by: Holland, H. D. and Turekian, K. K., Elsevier, Oxford, 273–291, https://doi.org/10.1016/B978-0-08-095975-7.01111-6, 2014.
Hindák, F.: On some planktonic coccoid blue-green algae characteristic by Fe-pecipitates, Algological Studies/Archiv für Hydrobiologie, Supplement Volumes, 32, 241–258, 1982.
Hindák, F.: Four new chroococcalean species (Cyanophyta/Cyanobacteria) from Western Slovakia, Biologia – Section Botany, 57, 415–422, 2002.
Hofer, F., Grogger, W., Kothleitner, G., and Warbichler, P.: Quantitative analysis of EFTEM elemental distribution images, Ultramicroscopy, 67, 83–103, https://doi.org/10.1016/S0304-3991(96)00106-4, 1997.
Iniesto, M., Moreira, D., Benzerara, K., Reboul, G., Bertolino, P., Tavera, R., and López-García, P.: Planktonic microbial communities from microbialite-bearing lakes sampled along a salinity-alkalinity gradient, Limnol. Oceanogr., 67, 2718–2733, https://doi.org/10.1002/lno.12233, 2022.
Jickells, T. D., An, Z. S., Andersen, K. K., Baker, A. R., Bergametti, G., Brooks, N., Cao, J. J., Boyd, P. W., Duce, R. A., Hunter, K. A., Kawahata, H., Kubilay, N., laRoche, J., Liss, P. S., Mahowald, N., Prospero, J. M., Ridgwell, A. J., Tegen, I., and Torres, R.: Global iron connections between desert dust, ocean biogeochemistry, and climate, Science, 308, 67–71, https://doi.org/10.1126/science.1105959, 2005.
Johnson, J. E.: From minerals to metabolisms: Evidence for life before oxygen from the geological record, Free Radical Bio. Med., 140, 126–137, https://doi.org/10.1016/j.freeradbiomed.2019.01.047, 2019.
Katoh, K. and Standley, D. M.: MAFFT multiple sequence alignment software version 7: improvements in performance and usability, Mol. Biol. Evol., 30, 772–780, https://doi.org/10.1093/molbev/mst010, 2013.
Keil, R. G., Montluçon, D. B., Prahl, F. G., and Hedges, J. I.: Sorptive preservation of labile organic matter in marine sediments, Nature, 370, 549–552, https://doi.org/10.1038/370549a0, 1994.
Keren, N., Aurora, R., and Pakrasi, H. B.: Critical roles of bacterioferritins in iron storage and proliferation of cyanobacteria, Plant Physiol., 135, 1666–1673, https://doi.org/10.1104/pp.104.042770, 2004.
Konhauser, K. O. and Urrutia, M. M.: Bacterial clay authigenesis: a common biogeochemical process, Chem. Geol., 161, 399–413, https://doi.org/10.1016/S0009-2541(99)00118-7, 1999.
Kranzler, C., Rudolf, M., Keren, N., and Schleiff, E.: Chapter Three - Iron in Cyanobacteria, in: Advances in Botanical Research, vol. 65, edited by: Chauvat, F. and Cassier-Chauvat, C., Academic Press, https://doi.org/10.1016/B978-0-12-394313-2.00003-2, 57–105, 2013.
Kranzler, C., Lis, H., Finkel, O. M., Schmetterer, G., Shaked, Y., and Keren, N.: Coordinated transporter activity shapes high-affinity iron acquisition in cyanobacteria, ISME J., 8, 409–417, https://doi.org/10.1038/ismej.2013.161, 2014.
Lepot, K., Addad, A., Knoll, A. H., Wang, J., Troadec, D., Beche, A., and Javaux, E. J.: Iron minerals within specific microfossil morphospecies of the 1.88 Ga Gunflint Formation, Nat. Commun., 8, 14890, https://doi.org/10.1038/ncomms14890, 2017.
Lingappa, U. F., Yeager, C. M., Sharma, A., Lanza, N. L., Morales, D. P., Xie, G., Atencio, A. D., Chadwick, G. L., Monteverde, D. R., Magyar, J. S., Webb, S. M., Valentine, J. S., Hoffman, B. M., and Fischer, W. W.: An ecophysiological explanation for manganese enrichment in rock varnish, P. Natl. Acad. Sci. USA, 118, e2025188118, https://doi.org/10.1073/pnas.2025188118, 2021.
Liu, L.-M., Li, D.-L., Deng, B., Wang, X.-W., and Jiang, H.-B.: Special roles for efflux systems in iron homeostasis of non-siderophore-producing cyanobacteria, Environ. Microbiol., 24, 551–565, https://doi.org/10.1111/1462-2920.15506, 2021.
Liu, X. and Millero, F. J.: The solubility of iron in seawater, Mar. Chem., 77, 43–54, https://doi.org/10.1016/S0304-4203(01)00074-3, 2002.
Nelson, N. and Junge, W.: Structure and energy transfer in photosystems of oxygenic photosynthesis, Annu. Rev. Biochem., 84, 659–683, https://doi.org/10.1146/annurev-biochem-092914-041942, 2015.
Nguyen, L.-T., Schmidt, H. A., von Haeseler, A., and Minh, B. Q.: IQ-TREE: a fast and effective stochastic algorithm for estimating maximum-likelihood phylogenies, Mol. Biol. Evol., 32, 268–274, https://doi.org/10.1093/molbev/msu300, 2015.
Pokrovski, G. S., Schott, J., Farges, F., and Hazemann, J.-L.: Iron(III)-silica interactions in aqueous solution: insights from X-ray absorption fine structure spectroscopy, Geochim. Cosmochim. Ac., 67, 3559–3573, https://doi.org/10.1016/S0016-7037(03)00160-1, 2003.
Qiu, G.-W., Jiang, H.-B., Lis, H., Li, Z.-K., Deng, B., Shang, J.-L., Sun, C.-Y., Keren, N., and Qiu, B.-S.: A unique porin meditates iron-selective transport through cyanobacterial outer membranes, Environ. Microbiol., 23, 376–390, https://doi.org/10.1111/1462-2920.15324, 2021.
Rasmussen, B., Muhling, J. R., Tosca, N. J., and Tsikos, H.: Evidence for anoxic shallow oceans at 2.45 Ga: Implications for the rise of oxygenic photosynthesis, Geology, 47, 622–626, https://doi.org/10.1130/G46162.1, 2019.
Raven, J. A., Evans, M. C. W., and Korb, R. E.: The role of trace metals in photosynthetic electron transport in O2-evolving organisms, Photosynth. Res., 60, 111–150, https://doi.org/10.1023/A:1006282714942, 1999.
Swanner, E. D., Wu, W., Hao, L., Wüstner, M. L., Obst, M., Moran, D. M., McIlvin, M. R., Saito, M. A., and Kappler, A.: Physiology, Fe(II) oxidation, and Fe mineral formation by a marine planktonic cyanobacterium grown under ferruginous conditions, Front. Earth Sci., 3, 6, https://doi.org/10.3389/feart.2015.00060, 2015.
Tagliabue, A., Bopp, L., Dutay, J.-C., Bowie, A. R., Chever, F., Jean-Baptiste, P., Bucciarelli, E., Lannuzel, D., Remenyi, T., Sarthou, G., Aumont, O., Gehlen, M., and Jeandel, C.: Hydrothermal contribution to the oceanic dissolved iron inventory, Nat. Geosci., 3, 252–256, https://doi.org/10.1038/ngeo818, 2010.
Tagliabue, A., Bowie, A. R., Boyd, P. W., Buck, K. N., Johnson, K. S., and Saito, M. A.: The integral role of iron in ocean biogeochemistry, Nature, 543, 51–59, https://doi.org/10.1038/nature21058, 2017.
Taylor, S. R.: Abundance of chemical elements in the continental crust: a new table, Geochim. Cosmochim. Ac., 28, 1273–1285, https://doi.org/10.1016/0016-7037(64)90129-2, 1964.
Tosca, N. J., Guggenheim, S., and Pufahl, P. K.: An authigenic origin for Precambrian greenalite: Implications for iron formation and the chemistry of ancient seawater, GSA Bull., 128, 511–530, https://doi.org/10.1130/B31339.1, 2016.
Wilhelm, S. W.: Ecology of iron-limited cyanobacteria: a review of physiological responses and implications for aquatic systems, Aquat. Microb. Ecol., 09, 295–303, https://doi.org/10.3354/ame009295, 1995.
Wollast, R., Mackenzie, F. T., and Bricker, O. P.: Experimental precipitation and genesis of sepiolite at earth-surface conditions, Am. Mineral., 53, 1645–1662, 1968.
Wood, A. M., Horan, P. K., Muirhead, K., Phinney, D. A., Yentsch, C. M., and Waterbury, J. B.: Discrimination between types of pigments in marine Synechococcus spp. by scanning spectroscopy, epifluorescence microscopy, and flow cytometry1, Limnol. Oceanogr., 30, 1303–1315, https://doi.org/10.4319/lo.1985.30.6.1303, 1985.
Zeyen, N., Benzerara, K., Li, J., Groleau, A., Balan, E., Robert, J.-L., Esteve, I., Tavera, R., Moreira, D., and Lopez-Garcia, P.: Formation of low-T hydrated silicates in modern microbialites from Mexico and implications for microbial fossilization, Front. Earth Sci., 3, 1–23, https://doi.org/10.3389/feart.2015.00064, 2015.
Zeyen, N., Benzerara, K., Menguy, N., Brest, J., Templeton, A. S., Webb, S. M., Gérard, E., Moreira, D., López-García, P., Tavera, R., and Morin, G.: Fe-bearing phases in modern lacustrine microbialites from Mexico, Geochim. Cosmochim. Ac., 253, 201–230, https://doi.org/10.1016/j.gca.2019.03.021, 2019.
Zeyen, N., Benzerara, K., Beyssac, O., Daval, D., Muller, E., Thomazo, C., Tavera, R., López-García, P., Moreira, D., and Duprat, E.: Integrative analysis of the mineralogical and chemical composition of modern microbialites from ten Mexican lakes: What do we learn about their formation?, Geochim. Cosmochim. Ac., 305, 148–184, https://doi.org/10.1016/j.gca.2021.04.030, 2021.