the Creative Commons Attribution 4.0 License.
the Creative Commons Attribution 4.0 License.
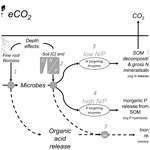
The influence of elevated CO2 and soil depth on rhizosphere activity and nutrient availability in a mature Eucalyptus woodland
Catriona A. Macdonald
David S. Ellsworth
Yolima Carrillo
Elevated carbon dioxide (eCO2) in the atmosphere increases forest biomass productivity but only where soil nutrients, particularly nitrogen (N) and phosphorus (P), are not limiting growth. eCO2, in turn, can impact rhizosphere nutrient availability. Our current understanding of nutrient cycling under eCO2 is mainly derived from surface soil, leaving mechanisms of the impact of eCO2 on rhizosphere nutrient availability at deeper depths unexplored. To investigate the influence of eCO2 on nutrient availability in soil at depth, we studied various C, N, and P pools (extractable, microbial biomass, total soil C and N, and mineral-associated P) and nutrient cycling processes (enzyme activity and gross N mineralisation) associated with C, N, and P cycling in both bulk and rhizosphere soil at different depths at the Free Air CO2 enrichment facility in a native Australian mature Eucalyptus woodland (EucFACE) on a nutrient-poor soil. We found decreasing nutrient availability and gross N mineralisation with depth; however, this depth-associated decrease was reduced under eCO2, which we suggest is due to enhanced root influence. Increases in available PO, adsorbed P, and the C : N and C : P ratio of enzyme activity with depth were observed. We conclude that the influences of roots and of eCO2 can affect available nutrient pools and processes well beyond the surface soil of a mature forest ecosystem. Our findings indicate a faster recycling of nutrients in the rhizosphere, rather than additional nutrients becoming available through soil organic matter (SOM) decomposition. If the plant growth response to eCO2 is reduced by the constraints of nutrient limitations, then the current results would call to question the potential for mature tree ecosystems to fix more C as biomass in response to eCO2. Future studies should address how accessible the available nutrients at depth are to deeply rooted plants and if fast recycling of nutrients is a meaningful contribution to biomass production and the accumulation of soil C in response to eCO2.
- Article
(1070 KB) - Full-text XML
- BibTeX
- EndNote
With elevated carbon dioxide (eCO2) in the atmosphere, higher photosynthesis rates can drive increases in forest biomass productivity (Ainsworth and Long, 2005; Norby and Zak, 2011). However, enhanced forest productivity in the long-term is not possible in areas where soil nutrients, particularly nitrogen (N) and phosphorus (P; Fisher et al., 2012), limit growth (Ellsworth et al., 2017; Terrer et al., 2019, 2018). In contrast, plant–microbe interaction under eCO2 might stimulate soil organic matter (SOM) decomposition and alleviate nutrient limitation (Luo et al., 2004; Drake et al., 2011; Wang and Wang, 2021). Higher root exudation rates and the stimulation of root growth and fine root production and turnover are all mechanisms that can potentially elicit SOM decomposition and subsequent nutrient release in the rhizosphere (Bernard et al., 2022). Root-mediated changes to SOM decomposition and nutrient cycling resulting from a changing climate may be especially important in forest systems where tree roots extend far below the soil surface and where eCO2 may also alter the root distribution with depth (Iversen et al., 2008; Iversen, 2010). However, the current understanding of nutrient cycling under eCO2 is mainly derived from surface soils, leaving mechanisms of the impact of eCO2 on nutrient availability at deeper depths unexplored (Jackson et al., 1996).
In the organic-rich surface layers of soil, where most fine roots are located, microbial activity is high (De Graaff et al., 2014). As SOM content, root density, and microbial biomass decline with depth, so does microbial activity and the rate of processes in soil (Hobley and Wilson, 2016). Despite this, deeper SOM has been found to be more responsive to fresh C inputs (Fontaine et al., 2007), with the implication that the decomposition effect of fresh C from the rhizosphere is likely to increase with depth. With an extending root system, such as that which may occur under eCO2 (Iversen, 2010), plants can introduce C where labile C may not have previously been abundant (Iversen et al., 2008; Kuzyakov and Blagodatskaya, 2015), thus promoting microbial activity and accelerated C decomposition at depth and potentially releasing nutrients. Moreover, increased C to the rhizosphere can shift the stoichiometric balance of C relative to soil nutrients (De Graaff et al., 2006; Kuzyakov, 2010; Carrillo et al., 2014). With the increased abundance of C, the microbial demand for N and P increases (Sistla and Schimel, 2012), in turn leading to an increase in microbial SOM decomposition (Bengtson et al., 2012; Carrillo et al., 2017). Furthermore, microbes have been found to improve their nutrient use efficiency to compensate for the stoichiometric imbalance of decomposer and substrate (Mooshammer et al., 2014). This is manifested through accumulation of N and P in microbial biomass, faster gross mineralisation rates, and smaller pools of available inorganic nutrients in the soil solution available for plant uptake. The phenomenon has been found for both N (Rütting et al., 2010) and P (Spohn, 2016; Spohn and Widdig, 2017). How these shifts in stoichiometry manifest in deeper soils is unclear but may have wide-ranging implications for forest productivity in response to eCO2.
Belowground allocation of plant-derived C has differential impacts on N and P, owing to inherent differences in their cycling. Plant-available N in inorganic form (ammonium and nitrate) is derived primarily through SOM decomposition involving the microbial processes of depolymerisation and the mineralisation of organic compounds and through nitrification (Schimel et al., 2015). In contrast, plant-available inorganic P (phosphate) can be sourced from both organic sources via microbial SOM decomposition and inorganic sources via dissolution from primary minerals and desorption from secondary minerals (Adeleke et al., 2017; Fig. 1). Plant and microbial P limitation is often driven by the mechanism of transitioning P between inaccessible organically bound P to an available inorganic form via a dissolved phase, which renders it susceptible to sorption to secondary mineral surfaces like clays and metal hydroxides (Gérard, 2016). In older, highly weathered soils of higher clay content, inorganic P availability can be more constraining for plant and microbial activity than N. In these soils, where the primary mineral P source has been depleted, most of the P left in the system is in organic form, either in biomass of plants and microbial cells or in SOM (Lambers et al., 2008; Walker and Syers, 1976). Increased root exudation and microbial activity in the rhizosphere can increase the decomposition of organic P in SOM through phosphatase enzyme production (Bünemann, 2015) and facilitate the release of mineral-adsorbed P by releasing organic acids, competing for sorption sites, and lowering soil pH. Therefore, the equilibrium of inorganic P between adsorbed and available forms is determined by root exudation, microbial enzyme production, and soil mineralogy (Fig. 1), all factors that are considered depth-dependent properties.
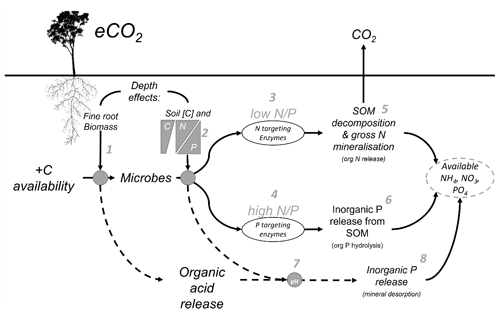
Figure 1Conceptual diagram of the mechanisms affecting nutrient availability as influenced by soil depth. Elevated CO2 increases the C availability belowground, but the effect of that extra C is moderated by depth-dependent mechanisms. (1) Root exudation in the rhizosphere soil is proportional to fine-root biomass which decreases with depth. (2) The microbial strategy to release nutrients is a function of soil C content and the N-to-P ratio, which also can change with depth. (3) The microbial strategy is a response to the N-to-P ratio either producing N-targeting enzymes in low N-to-P conditions or (4) P-targeting enzymes in high N-to-P conditions. (5) Nitrogen-targeting enzymes act to decompose SOM and increase gross N mineralisation, transforming organic N into NH and ultimately NO, which are available for plant uptake. (6) P-targeting enzymes cut phosphates from organic molecules by hydrolysis. (7) One further mechanism behind the nutrient release affected by eCO2 is that soil pH is changed, thus impacting the soil sorption capacity by the organic acid exudates from the roots and the microbial mineralisation thereof. (8) The decreased acidity tips the balance of phosphates in solid and in solution to increase the soil solution content and P availability by mineral desorption.
Given that N and P cycling in soil differs, and that the factors controlling those processes can vary with depth, soil nutrient stoichiometry also tends to vary with depth (Li et al., 2016). The soil C : N ratio tends to decrease with depth under the increased microbial processing of C. Declining SOM content with depth will also lower the N content. In contrast, soil C : P can decrease, but more often remains unchanged, as mineral-adsorbed P remains in soil despite the SOM content declining; the potential implication of this is a reduction in soil N : P at depth (Li et al., 2016; Zhao et al., 2017). Therefore, many heavily weathered surface soils may be constrained in available PO, but, at depth, some soils may be N limited. This is important in the context of eCO2 because the response of SOM decomposition to increased labile C availability could be dependent on which nutrient is most limiting to microbes (Dijkstra et al., 2013), which in turn would be expected to depend on the depth. Accordingly, extrapolations of nutrient limitation from surface soil processes to deeper soil layers become unreliable without accounting for mechanisms controlling nutrient processing as the stoichiometry changes with depth. The lack of experimental evidence concerning soil nutrient cycling processes in deeper soil renders the assumption that native biomes will increase their productivity under eCO2 contentious (Iversen et al., 2011; Rumpel and Kögel-Knabner, 2011).
The Eucalyptus Free Air CO2 Enrichment (EucFACE) facility in eastern Australia has experimentally exposed a Eucalyptus woodland, on a low N and P soil, to eCO2 concentration (+150 ppm, parts per million) continuously since 2013 (Drake et al., 2016). To date, the site has not seen any evidence of an increase in aboveground biomass in the Eucalyptus trees under eCO2 (Ellsworth et al., 2017), despite an increase in the photosynthetic rate of both the dominant tree species and the understorey grasses in this ecosystem (Ellsworth et al., 2017; Pathare et al., 2017). The lack of plant biomass response to the CO2 treatment is hypothesised to be caused by a severe P limitation of the soil, additions of which were shown to increase plant biomass in a tree stand close by that was not exposed to eCO2 (Crous et al., 2015). In this system, the mineralisation and decomposition of SOM have only been investigated in the upper soil layers (Hasegawa et al., 2016; Castañeda-Gómez et al., 2020, 2021). The potential for the plants in this system to utilise nutrients in the deeper soil layers of the top metre of soil is relevant because this highly weathered, nutrient-poor soil system may already have reached a maximum efficiency for nutrient cycling in the upper soil layer where SOM and microbial activity is greater. Additionally, Eucalyptus trees are known to have very deep roots to access water from groundwater aquifers (Laclau et al., 2013), though fine roots capable of nutrient acquisition are thought to be most abundant in the surface soil layers (Piñeiro et al., 2020). Despite the considerable number of P-limited forests globally, there are still large uncertainties surrounding rhizosphere activity and nutrient cycling in older, P-limited soils compared to younger soils in the Northern Hemisphere that are often N limited (Fisher et al., 2012; Terrer et al., 2019).
To investigate the influence of eCO2 on nutrient availability in soil at depth, we studied various C, N, and P pools (extractable, microbial biomass, total soil C and N, and mineral-associated P) and nutrient cycling processes (enzyme activity and gross N mineralisation) associated with C, N, and P cycling in both bulk and rhizosphere soil at different depths at the EucFACE facility. We asked the following questions: (1) what is the difference between rhizosphere and bulk soil in terms of soil properties and is this changed with soil depth? (2) What is the effect of eCO2 on nutrient availability and C : N : P stoichiometry in the rhizosphere, and does it change with soil depth? Given that increased root exudation will prime microbial nutrient mining, we hypothesise that (1) nutrient availability (inorganic N and P) will be higher in the rhizosphere compared to bulk soil. We also hypothesise that (2) eCO2 will increase the availability of P to a greater extent than N in surface soil, but not at deeper layers, and that (3) eCO2 will have less impact on N than P availability and increase the processes contributing to P release (P-targeting enzymes) more so than N release (N-targeting enzymes and gross N mineralisation). This effect will be less important with depth because the overall N : P ratio declines with depth, alleviating the P limitation and thus shifting the demand from P to N.
2.1 Experimental design
The study was performed at the Eucalyptus Free-Air CO2 Enrichment (EucFACE) experiment located in a Cumberland Plain woodland with mature Eucalyptus trees in Sydney, Australia (33∘37′ S and 150∘44′ E; 23 m a.s.l., above sea level). The site has six experimental rings (n=3), each with a diameter of 25 m. The CO2 treatment has been implemented to three of the rings (eCO2) since September 2012 and reached +150 ppm above ambient CO2 (aCO2) in February 2013 (Ellsworth et al., 2017). The remaining three rings are controls (aCO2). The soil at the site is a developing red and/or yellow aeric podsol in weakly organised alluvial deposits (Ross et al., 2020), including iron–manganese nodules (Clarendon formation) with a metal-oxide-rich (field observation) transition to a hardpan clay layer, called Londonderry clay (Atkinson, 1988), found at a variable depth throughout the site (between 35–85 cm). The dominant tree species is Eucalyptus tereticornis, and the dominant understorey grass is Microlaena stipoides. The site has an average precipitation of 800 mm yr−1, with a total precipitation of 16.8 mm in the month leading up to the sampling campaign. The yearly mean temperature was 17 ∘C. For a detailed site description, see Ellsworth et al. (2017).
2.2 Field sampling, soil preparation, and root biomass determination
Soil cores (5 cm diameter) were collected from all rings in September 2017. A total of 12 cores were taken in each ring, spread as three in each of the four pre-established 2 × 2 m subplots designated for soil sampling (four subplots per ring; a total of 72 soil cores). Each core was sampled down to the clay layer, which varied with depth across the site (35–85 cm). Each core was divided into the three depths for investigation, i.e. 0–10 and 10–30 cm and a transition (a 10 cm interval where sandy loam transitioned into clay). Samples were kept cool until further processing in the laboratory which took place within 1 week of collection. Although the depth of the transition layer differed throughout the site, the chemical properties are assumed to be similar within this zone across the plots, as the water periodically builds up above the clay before it drains, creating conditions for podzolisation. Soils were processed to separate bulk from rhizosphere soil. The rhizosphere soil was defined as any soil that was still attached to the fine roots when these were separated from the soil, and soil was collected by gently shaking the roots. All other soil in the core was considered to be bulk soil. For both rhizosphere soil and bulk soil, subplots 1 and 2 and 3 and 4, were combined to two samples per ring and depth (n=6 samples per ring). This was necessary in order to have sufficient rhizosphere soil sample for subsequent analysis. Samples were sieved to <2 mm. Subsamples for potential enzyme activity were frozen (−20 ∘C) immediately after sieving. Soil samples to be analysed for nutrient availability and microbial biomass were stored at 5 ∘C until processed. The roots already handpicked for rhizosphere soil were washed and dried within a week of sampling and later separated into fractions larger and smaller than 3 mm in diameter. Additionally, any remaining roots were hand-picked from a subsample (∼ 50 g) of sieved soil and scaled to the total sample weight.
2.3 Extractable carbon, nitrogen, and phosphorus and microbial biomass
Microbial biomass C, N, and P were determined on fresh soil following the fumigation extraction method of Vance et al. (1987). Briefly, fumigated samples were treated with ethanol-free CHCl3 under vacuum conditions (fumigated for 4 d for C and N and 1 d for P) and then extracted for C, N, and P using K2SO4 and Bray P1. All extracts were filtered through Whatman 42 grade filter papers and frozen until analysis. Fumigated and unfumigated extracts of K2SO4 (0.5 M) were analysed for C and N on TOC-L (total organic carbon analyser; Shimadzu Corporation, Japan). Fumigated and unfumigated extracts of Bray P1 were analysed for PO; additionally, unfumigated K2SO4 extracts were analysed for inorganic N (ammonium and nitrate), according to Rayment and Lyons (2011), by colorimetry (AQ2 discrete analyser; SEAL Analytical, Inc., Mequon, WI, USA). Soil was dried (70 ∘C) for the determination of gravimetric soil moisture, and air-dried soil was used for pH (1:5 s:w; S20 SevenEasy™ pH; Mettler-Toledo International Inc., Columbus, OH, USA). Subsamples of the air-dried soil were cleared of visible root fragments and analysed for total soil C and N (LECO TruMac CN analyser; LECO Corporation, USA) and for mineral-associated inorganic P.
2.4 Mineral-adsorbed inorganic phosphorus
To quantify mineral-associated inorganic P, a 1 g air-dried subsample was extracted with NaOH-Na2EDTA (0.25 M NaOH and 0.05 M Na2EDTA) and horizontally shaken for 16 h at 80 rpm (revolutions per minute), after which it was filtered (Rayment and Lyons, 2011). Extracts were diluted 1:10 with sterile water and analysed using the Malachite Green reagent (Ohno and Zibilske, 1991) in a clear 96 well plate. The plates were analysed by colorimetry on a CLARIOstar plate reader (BMG LABTECH, Germany) at 610 nm after 1 h incubation at 25 ∘C.
2.5 Pool dilution for gross N mineralisation rates
To assess the gross N mineralisation rate, an isotope pool dilution assay using 15N-enriched ammonium was made with a series of laboratory incubations, following the method of Rütting et al. (2011). Ammonium concentration and ammonium 15N excess from two time points was done on KCl extracts (Stange et al., 2007; Putz et al., 2018) with SpinMass (Sample Preparation of Inorganic Nitrogen MASSpectrometer) at ISOGOT (Department of Earth Sciences, University of Gothenburg, Sweden). The 15N label was added in duplicate to fresh and sieved soil samples (5 g), with a label consisting of 10 µg (15NH4)2SO4 (15N fraction of 99 %; Cambridge Isotope Laboratories, Inc.) in 0.25 mL Milli-Q water. After label addition, samples were incubated for 15 min and 24 h under steady temperature (20 ∘C) and in darkness. The incubations were extracted with 1 M KCl (15 mL), shaken for 1 h at 120 rpm, and filtered through 42 grade, ashless Whatman filters and frozen until analysis. All gross mineralisation rates were calculated using the equation in Kirkham and Bartholomew (1955).
2.6 Potential enzyme activity method
The potential activity of seven enzymes associated with C, N, and P mineralisation were determined for bulk and rhizosphere soil, respectively. For this, we used fluorometrically labelled substrates, following the method of Bell et al. (2013). In total, 2 g of frozen soil was mixed to a slurry (1:33 w:v) with Milli-Q water in a laboratory blender for 1 min. The slurry was pipetted into 96 well plates with three technical replicates and given fluorescent substrates (4-methylumbelliferone, MUB, and 7-amino-4-methylcoumarin, MUC), in accordance with the Bell et al. (2013) protocol. The samples were then incubated at 25 ∘C for 3 h and analysed for fluorescence with a CLARIOstar plate reader (BMG LABTECH, Germany). Four enzymes (α-D-glucopyranoside (AG), β-D-glucopyranoside (BG), β-D-cellobioside (CB), and β-D-xylopyranoside (XYL)) targeted C-rich compounds (sugar, cellulose, and hemicellulose), two enzymes (L-Leucine-7-aminopeptidase (LAP) and N-acetyl-β-D-glucosamine (NAG)) targeted N-rich compounds (proteins and chitin), and acid phosphatase (PHOS) targeted organic compounds with P. These enzymes are considered to be representative of the total enzyme pool active in the soil; however, storage in −20 ∘C may have altered the potential enzymatic activity, and comparisons with activities in fresh soil from other land uses should be made with caution (Lane et al., 2022).
2.7 Statistical analyses
The impact of CO2 treatment, depth, and their interaction were assessed separately for bulk and rhizosphere soil at three depth levels (0–10 and 10–30 cm and transition). Two soil depths (0–10 and 10–30 cm) were used in the analysis of rhizosphere where insufficient amounts of rhizosphere soil were recovered during sampling. The subsequent pseudo-replication created with two samples per experimental unit (ring) were dealt with by using a linear mixed effects model, where CO2 and depth and their interactions were fixed factors and the ring a random factor with individual intersects (lme4 package; Bates et al., 2015), corresponding to the EucFACE experimental design (Hasegawa et al., 2016). To assess the role of CO2 and depth effects on rhizosphere soil, we used a linear mixed-effects model with CO2, depth (two depths at 0–10 and 10–30 cm), and soil type (bulk and rhizosphere) as fixed factors with all interactions and the ring as a random factor with individual intersects (Bates et al., 2015). For the gross N mineralisation rate in the deepest layer (10–30 cm depth), ammonium concentrations in most samples were below the detection limit.
Significance was determined with the ANOVA (analysis of variance) function (car package; Fox and Weisberg, 2019) with the Kenward–Roger degrees of freedom estimation. Post hoc analysis was performed with the glht function for multi-comparison (multcomp package; Hothorn et al., 2008). The post hoc Tukey analysis of all CO2, depth, and soil factors were combined into their unique interactions and then processed in the linear mixed-effects model, as previously described. The normal distribution of residuals was assessed, and log transformations were performed where required to meet model assumptions.
3.1 Fine-root biomass
Fine-root biomass density significantly decreased with depth and ranged from 2.75 mg g−1 in the 0–10 cm depth to 0.12 mg g−1 in the transition depth (Fig. 2). There was a significant interaction between depth and CO2, where, in the topsoil (0–10 cm), elevated CO2 (eCO2) samples had a 28 % lower fine-root density than ambient CO2 samples.
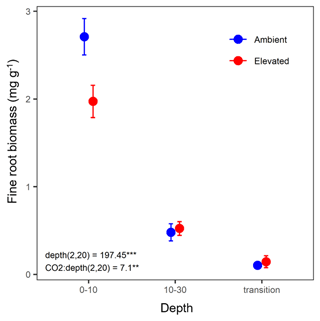
Figure 2Biomass of fine roots of less than 3 mm thickness (mg g−1) in the mature Eucalyptus forest soil exposed to ambient (blue) and elevated (red) CO2 for three depths (0–10 and 10–30 cm and transition). Error bars indicate standard error. Mixed-effects model output stated the (degrees of freedom, Df, and Df residuals) F statistic presented, and asterisks for the P values for significance are as indicated, where indicate P<0.001, and indicate P<0.01.
3.2 Carbon in total soil and dissolved and microbial biomass pools
Dissolved organic carbon (DOC) declined significantly with depth for both bulk and rhizosphere soil, and the decrease by depth was stronger for rhizosphere soil (25 %) than for bulk soil (11 %; Fig. 3a and c). The DOC was significantly higher (by 24 %) in rhizosphere soil than bulk soil (Fig. 2 and Table 1) when averaged across depth (0–10 and 10–30 cm depths). Microbial C declined significantly with depth for both bulk soil and rhizosphere soil (Fig. 3b and d) and was significantly higher in rhizosphere soil (Table 1; Fig. 3) by 36 % (transition was excluded). Total soil C content had a significant effect of depth and an interaction between CO2 treatment and depth (Table 1); the percent soil C content was higher in the 0–10 cm depth under eCO2 but was not different from ambient in the deeper depths (10–30 cm and transition; Table 2).
Table 1The effect of the factors CO2 (eCO2 and eCO2), soil depth (0–10 and 10–30 cm and transition), and soil type (bulk and rhizosphere soil) and their interactions, shown as a model F statistic output. Asterisks and bold typeface indicate the level of significance of P values, with for P<0.001, for P<0.01, and * for P<0.05. The extractable nutrients NH, NO and PO, DOC, and microbial biomass C, N, and P are modelled on a milligram per kilogram (mg kg−1) basis, the gross N mineralisation rate on a milligram per kilogram per day (mg kg−1 d−1) basis, and soil C and N in percent. Note that Df is for degrees of freedom.
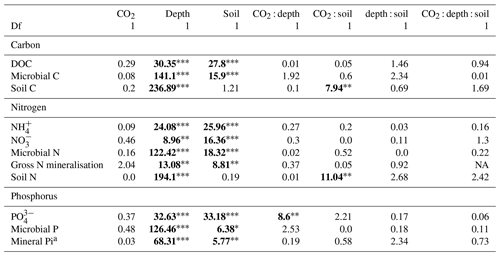
a Three depths (0–10 and 10–30 cm and transition) used in the factor of depth, with 2 Df.
3.3 Rate of gross N mineralisation and N pools
Measured soil N content (including NH, NO, and microbial N) declined significantly with depth for both bulk and rhizosphere soils (Fig. 4). Ammonium, nitrate, microbial N, and gross N mineralisation (Table 1) were significantly higher in rhizosphere soil than in the bulk soil at both 0–10 and 10–30 cm depths (Table 1). Total soil N content showed a significant interaction between CO2 treatment and depth (Table 1), where the percent of soil N content was higher in the 0–10 cm depth under eCO2 but was the same as ambient in the deeper depths (10–30 cm and transition).
The gross N mineralisation rate declined significantly with depth and was significantly higher in rhizosphere soil compared to bulk soil; furthermore, eCO2 did not have a significant effect (Fig. 5; Table 1). The multiple comparisons showed the 0–10 cm bulk soil samples as being of a similar magnitude as the rhizosphere 10–30 cm samples. The 0–10 cm rhizosphere treatment was significantly higher than the ambient 10–30 cm rhizosphere (Fig. 5), though it cannot be statistically separated from any other treatment group due to the high variability.
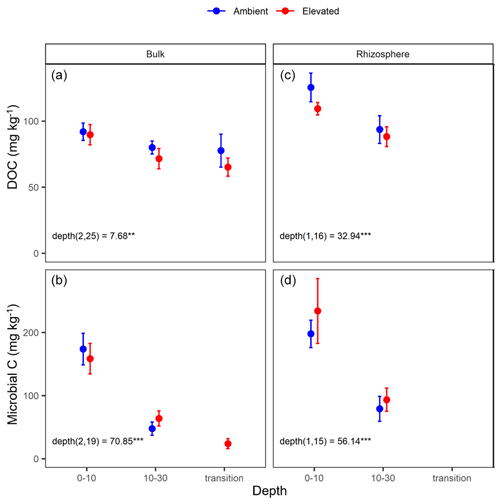
Figure 3Dissolved organic carbon (DOC) and microbial biomass carbon (C) content for bulk and rhizosphere soil of the mature Eucalyptus forest soil exposed to ambient (blue) and elevated (red) CO2 for three depths (0–10 and 10–30 cm and transition). Error bars indicate the standard error. Mixed-effects model output is stated (degrees of freedom and Df residuals), the F statistic is presented, and asterisks for the P values for significance are as indicated, where indicate P<0.001, and indicate P<0.01. Results from the statistical analysis of comparison of soil types (bulk and rhizosphere) are presented in Table 1.
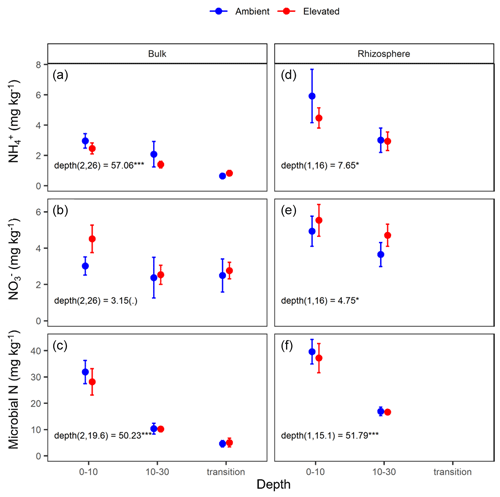
Figure 4Nitrogen (N) pools in the forms of ammonium (NH), nitrate (NO), and microbial biomass N for the bulk and rhizosphere soil of the mature Eucalyptus forest soil exposed to ambient (blue) and elevated (red) CO2 for three depths (0–10 and 10–30 cm and transition). Error bars indicate standard error. The mixed-effects model output is stated (degrees of freedom and Df residuals) and the F statistic presented. Asterisks for the P values for significance are as indicated, where indicate P<0.001, indicate P<0.01, * indicates P<0.05, and (.) indicates a tendency to a significance P<0.1. Results from the statistical analysis of comparison of soil types (bulk and rhizosphere) are presented in Table 1.
3.4 Soil phosphorus
The three assessed P contents (extractable PO, microbial P, and mineral-associated inorganic P) significantly declined with increasing depth and were higher in the rhizosphere compared to bulk soil (Table 1 and Fig. 6). For PO, there was a significant interaction between CO2 and depth, as the concentration of PO did not decline with depth under eCO2. Phosphate concentration in the 10–30 cm depth tended to be higher in eCO2 soils compared to aCO2 soils (Fig. 6d). Microbial P in the bulk soil interacted with CO2 treatment and depth, where microbial P was lower under eCO2 compared to aCO2 in the 0–10 cm depth only (Fig. 6).
3.5 Enzymatic activity results
Enzyme activities decreased significantly with depth but did not differ significantly between soil or CO2 treatment (Tables 5 and 6). One exception to the general trend was CB (β-D-cellobioside) that did not decrease with depth and was significantly higher in rhizosphere soil compared to bulk soil. Notable is the difference in magnitude for N-targeting and P-targeting enzymes, where P enzymes were twice as abundant as N. The two-to-one pattern was maintained as the enzyme activity declined with soil depth.
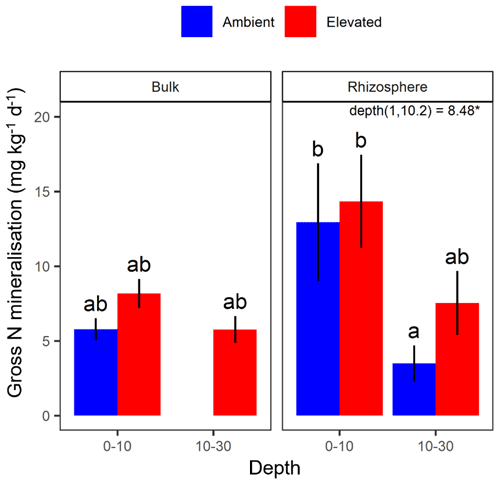
Figure 5Gross N mineralisation for bulk and rhizosphere soil of the mature Eucalyptus forest soil exposed to ambient (blue) and elevated (red) CO2 for two depths (0–10 and 10–30 cm). Error bars indicate the standard error. The mixed-effects model output is stated (degrees of freedom and Df residuals), and the F statistic is presented. Asterisks for the P value for significance are provided, where * indicates P<0.05. Results from statistical analysis of comparison of soil types (bulk and rhizosphere) are presented in Table 1.
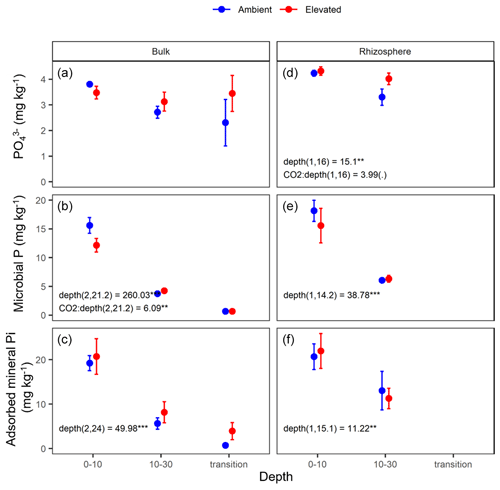
Figure 6Measured soil P pools in the forms of inorganic P (PO), microbial biomass P, and mineral-associated phosphate through adsorption for bulk and rhizosphere soil of the mature Eucalyptus forest soil exposed to ambient (blue) and elevated (red) CO2 for three depths (0–10 and 10–30 cm and transition). Error bars indicate the standard error. Mixed-effects model output is stated (degrees of freedom and Df residuals), and the F statistic is presented. Asterisks for the P values for significance are as indicated, where indicate P<0.001, indicate P<0.01, * indicates P<0.05, and (.) indicates a tendency to a significance P<0.1. Results from statistical analysis of comparison of soil types (bulk and rhizosphere) are presented in Table 1.
3.6 Stoichiometry of soil nutrient pools (C, N, and P) and soil enzymes
The C : N and C : P of extractable nutrients in the bulk soil increased significantly with depth by 24.9 and 20.9 units of C per nutrient, respectively. However, under eCO2, the C : N and C : P stoichiometry did not increase in bulk soil (Tables 3 and 4). The rhizosphere soil N : P ratio significantly declined with depth. When soil was included as an interactive factor in the model (Table 4), C : N was significant by depth : soil. For the extractable C : P, ratio both the interaction between CO2 : depth and CO2 : soil was significant, where the C : P ratio declined with eCO2 and depth but increased with depth when ambient. In the microbial biomass, only C : P significantly increased with depth in bulk soil. The N : P of extractable N and P and microbial biomass stoichiometry significantly increased with depth. When both bulk and rhizosphere soil was considered (only 0–10 and 10–30 cm depth), soil and depth were significantly affected by extractable C : N and N : P, and the interaction of soil and depth was significant for soil C : N (Table 4). The bulk soil total C : N ratio decreased significantly with depth by 9 units. The rhizosphere soil C : N ratio increased slightly by only 1 unit, yet still significantly, with depth. There was also a significant interaction between CO2 and depth in the C : N and C : P ratio of the enzymes (Tables 5 and 6). The C : N and C : P ratios decreased 0.7 and 0.4 units with depth in ambient conditions but increased by 0.4 and 0.3 with depth in eCO2. The ratio between N- and P-targeting enzymes did not change with depth but was maintained in the range of 0.5–0.7 N enzymes per P enzyme. The pH showed a marginally significant effect from the interaction of depth and CO2, where the pH increased slightly in the transition under eCO2 (Table 5).
Table 3Extractable and microbial C, N, and P stoichiometry and the soil C : N ratio for bulk soil (B) on the left of each column and rhizosphere soil (R) on the right for a mature Eucalyptus forest soil exposed to ambient and elevated CO2 for three depths (0–10 and 10–30 cm and transition). Stoichiometry was calculated on a milligrams per kilogram (mg kg−1) mass basis, with the standard error given below in parenthesis.
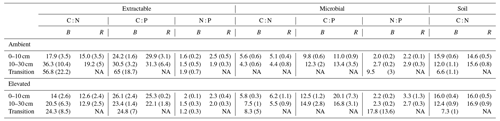
NA: not available.
Table 4Model F statistic and significance of extractable and microbial C, N, and P and soil C : N. Where bulk soil and rhizosphere soil are shown separately, the bulk soil was modelled with three depth levels, whereas rhizosphere soil was modelled with only two. Where bulk soil and rhizosphere soil are shown together (a), only the 0–10 and 10–30 cm depths are included in the model. The significance of P values are as indicated, where indicate P<0.001, indicate P<0.01, and * indicates P<0.05.
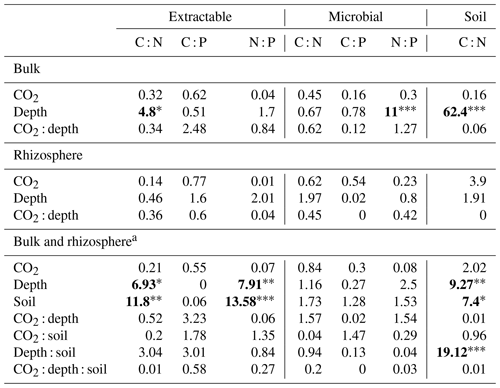
Asterisks and bold typeface indicate the level of significance of P values, with for P<0.001, for P<0.01, and * for P<0.05.
We sampled rhizosphere soil and bulk soil in a depth profile in a Eucalyptus woodland experimentally exposed to eCO2 for 5 years, with the goal to investigate how root activity influences nutrient availability and stoichiometry across depth and under eCO2. Supporting our hypothesis (1), the nutrient availability increased in rhizosphere soil compared to bulk soil. However, we found no clear evidence to support the hypothesis that eCO2 affected the rhizosphere soil to a greater extent than the bulk soil (Table 1). There was some evidence to support hypothesis (2) that eCO2 affected the availability of P more than of N, as available PO was more increased with depth in elevated CO2 compared to ambient CO2 (Fig. 6). Additionally, the low N : P ratio of enzymes supports hypothesis (3), which is that P was more limiting than N (Table 5).
4.1 Depth effects on soil nutrients and microbial biomass
The effect of depth was significant overall, and the microbial biomass C, N, and P, DOC, inorganic N (NH and NO), inorganic P (PO), and mineral-adsorbed inorganic P all decreased in availability with depth (Table 1). However, under eCO2, when bulk and rhizosphere soil were analysed separately, the availability of extractable P in the soil solution in the rhizosphere did not decline with depth (Fig. 6d). Increased P availability below surface soil in the rhizosphere has been found in previous studies at the site (Ochoa-Hueso et al., 2017), which measured nutrient availability down to 30 cm depth, and in other forest sites investigating nutrient availability in deeper soil (Blume et al., 2002; Rumpel and Kögel-Knabner, 2011; De Graaff et al., 2014; Li et al., 2016). Notably, all enzyme activity, including phosphatase activity, declined with depth independently of the CO2 condition (Table 5), indicating that the rhizosphere increase in P availability in the deeper soil was not due to higher SOM decomposition. Contrary to the non-response of the microbial C and N concentration, the microbial P concentration decreased under eCO2 in the 0–10 cm depth in the bulk soil (Fig. 6c). This is similar to the negative effect of CO2 on fine root density (Fig. 2), suggesting that root density and microbial P respond similarly to eCO2, since both decreased.
Stoichiometry changed with depth differently for bulk and rhizosphere soil. The ratio of extractable C to N and to P in bulk soil increased with depth, as DOC decreased less with depth than inorganic N and P. However, contrary to our hypothesis, the ratio between N and P was constant across depth in bulk soil. Hence, without the influence of roots, N and P both declined at a similar rate, while the total magnitude of N is larger than P as both decreased with depth. In the rhizosphere soil, the ratio between DOC and inorganic N and P remained constant with depth, while the N : P ratio significantly decreased; hence, the rhizosphere inorganic P became relatively more available than N at deeper soil. We suggest there was more P available because there were fewer fine roots and lower microbial biomass to immobilise it. Furthermore, inorganic P decreased with depth as more resources were invested to access it; thus, it was supported by the consistently higher P-targeting enzyme activity than N enzyme activity.
Table 5Potential enzyme activity and stoichiometry of enzymes targeting C, N, and P compounds (µmol h−1 g−1) for bulk and rhizosphere soil of a mature Eucalyptus forest soil exposed to ambient and elevated CO2 for three depths (0–10 and 10–30 cm and transition), with the standard error in parenthesis. Four enzymes (α-D-glucopyranoside (AG), β-D-glucopyranoside (BG), β-D-cellobioside (CB), and β-D-xylopyranoside (XYL)) targeted C-rich compounds (sugar, cellulose, and hemicellulose), two enzymes (L-Leucine-7-aminopeptidase (LAP) and N-acetyl-β-D-glucosamine (NAG)) targeted N-rich compounds (proteins and chitin), and acid phosphatase (PHOS) targeted organic compounds with P.
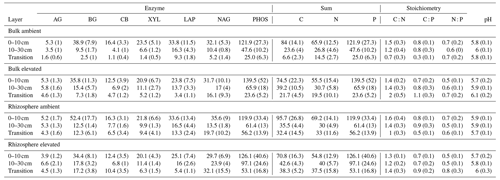
4.2 Rhizosphere effects on nutrient availability and mineralisation across depths
It is a paradigm in rhizosphere research that microbial activity is high near the root because of the input of energy in the form of newly photosynthesised C (Kuzyakov et al., 2000; Kuzyakov and Cheng, 2001). Supporting this, we found that microbial biomass and nutrient availability were higher in the rhizosphere soil compared to bulk soil. Furthermore, the gross N mineralisation rate increased in the rhizosphere compared to bulk soil. Given the positive links found between gross N mineralisation and SOM decomposition (Bengtson et al., 2012; Zhu et al., 2014), these findings suggest that root–microbe interactions are facilitating decomposition and increasing nutrient availability (Andresen et al., 2020).
In contrast, the potential activities of enzymes responsible for depolymerising and hydrolysing N and P from SOM did not increase closer to the root (Table 5), thus supporting previous findings from the site that reported that enzyme activities were not higher in the presence of roots (Ochoa-Hueso et al., 2017; Castañeda-Gómez et al., 2021). The lack of enzymatic activity response to roots in both surface and deeper soil depths could be due to the microbial community lacking access to energy and N to be able to synthesise enzymes (Olander and Vitousek, 2000), although there is no indication N or C are limiting for enzyme production in this system. Alternatively, because of greater nutrient availability, there is a reduced need for enzyme production (Sinsabaugh et al., 2009). Finally, a shift in the microbial community composition favouring fungi over bacteria in the rhizosphere, as has been observed at the site, could lead to lower enzyme production per unit biomass (Castañeda-Gómez et al., 2021).
The stoichiometry of enzymes targeting N and P is an indicator of microbial nutrient demand (Sinsabaugh et al., 2009). In this system, N does not appear to be the most limiting nutrient, given the low ratio of N-to-P-targeting enzymes. The low-enzyme N : P ratio suggests that P is more highly sought after by the microbes in this system (Allison and Vitousek, 2005; Sinsabaugh et al., 2008). We found this independent of soil depth, indicating that P is in higher demand than N in the entire soil profile. Interestingly, no difference in either enzyme amount or stoichiometry was found between bulk soil and rhizosphere soil, which indicate that, given a higher C availability in the rhizosphere, microbes did not increase their enzyme production to mine for organic P. However, P can also be sourced from non-organic sources (Gérard, 2016). This is supported by the high levels of mineral-associated inorganic P in the rhizosphere at depth (Fig. 5). We suggest that non-organic sources of P may be important to microbes in the rhizosphere as an alternative to high-energy-cost enzyme production. Although soil P accumulates in the soil organic fraction with increasing soil age (Crews et al., 1995), this soil is also rich in metal oxides, with large surfaces capable of adsorbing phosphate cations (Achat et al., 2016) that root activity in the rhizosphere can release, with the help of organic acids, without decomposing SOM (Adeleke et al., 2017).
The pattern of decline in nutrient concentrations in deeper soil profiles is well documented (Jobbágy and Jackson, 2001). Though a decline in these concentrations still occurs in the rhizosphere soil with depth, here we can show that root activity counteracts the decline associated with depth, maintaining a higher microbial biomass and nutrient availability in the rhizosphere soil compared to bulk soil (Finzi et al., 2015). Together with the evidence of a higher gross N mineralisation rate in the rhizosphere soil, we suggest that, in this P-limited mature forest, roots can drive the availability of both N and P even in deeper soil. Because we did not find a significant increase in potential enzyme activity in the rhizosphere (Table 6), this effect can instead be driven by microbial biomass turnover, community shift (Castañeda-Gómez et al., 2021), and a strong recycling of nutrients without large decomposition of SOM requiring enzyme activity. Although we can show that deep rhizosphere has an impact on available nutrients, our study cannot assess if plants are utilising the increased availability, though increased root turnover has been reported (Piñeiro et al., 2020), suggesting that is the case. However, assuming that at least part of plant nutrient immobilisation is via the diffusion of concentration gradients (Gilroy and Jones, 2000), a higher nutrient concentration in the deeper rhizosphere soil is likely benefiting plants and microbes.
4.3 Elevated CO2 and depth dependency of rhizosphere effects
Elevated CO2 increases C availability and nutrients in the rhizosphere through increased rhizodeposition and nutrient mobilisation (Phillips et al., 2011; Kuzyakov et al., 2019). Because root density declines with increasing depth, we hypothesised that the effects of eCO2 on C and nutrient availability will be less important with depth. Contrary to that hypothesis, we found that eCO2 interacted with depth by increasing the inorganic P availability at depth under eCO2. Furthermore, mineral-associated inorganic P was constantly higher at depth in the bulk soil under eCO2, though the trend is not significant. Metal hydroxide mineral-rich clay is capable of the strong adsorption of negative ions and organic complexes (Jilling et al., 2018; Rasmussen et al., 2018), which is present at EucFACE. Changes in pH can affect the equilibrium between mineral adsorption and solution concentration, though the small increase in pH that was detected in the rhizosphere soil (less than 0.5 units compared to bulk soil; Table 5) is not necessarily enough to change the sorption capacity. Rather, the higher PO adsorption and concentration in the solution indicates that higher rates of phosphate processes exist in that space. The different forms of soil P thus appear to respond to different drivers, while the microbial biomass did not immobilise the additionally available PO or access the mineral-associated P. This supports the idea that the microbes are not limited by P at depth. The question remains if plants can access the increased P availability at deeper soils.
The relative content and activity of C-degrading compared to N- and P-degrading enzymes was higher in the deeper soil under eCO2 for both rhizosphere soil and bulk soil. These trends with depth suggest that the surface soil is more limited by nutrients (i.e. N and P poor) compared to deeper layers where C is a limiting factor for activity. Thus, eCO2 may cause increased microbial activity and enzyme synthesis at depth rather than in the surface soil. The relative content of enzymes for N-to-P release ranged from 0.5 to 0.8, and this indicated a biological P limitation rather than N limitation. That ratio was consistent through the depth profile, though the total enzyme activity declined with depth. Only cellulase activity (CB; Table 5) was constant in all layers, possibly indicating that plant matter has the potential of being decomposed throughout the soil profile. It was demonstrated by Castañeda-Gomez et al. (2020) that root litter decomposition is increased under eCO2 at the site and contributes to C loss from the system. Root litter decomposition can thus be an important source of nutrient release at depth. Furthermore, eCO2 has been found to increase the rate of root turnover in this system (Piñeiro et al., 2020), which is one of the main sources of C supply to the deeper soil, other than increased root exudation.
In this study, the observed lack of influence of eCO2 on nutrient availability and N mineralisation at the surface is likely due to the topsoil being less limited by C than deeper soils (depth and CO2 interaction). Though enzyme activities decrease with depth, they are more abundant per unit of soil C deeper in the profile. Given the rather low eCO2 fertilisation effect found on photosynthetic rate (Ellsworth et al., 2017; Jiang et al., 2020) and root production in this system (Piñeiro et al., 2020), the presumed limited increase in C release belowground is likely turned over without affecting the SOM decomposition. Mineral-adsorbed P forms are, however, sensitive to root-derived changes in pH (Jones and Darrah, 1994), representing a different mechanism for affecting the P cycle that is separate from SOM decomposition (McGill and Cole, 1981). In the scenario where nutrients mostly become available through recycling, rather than SOM decomposition, it is unlikely that plant nutritional requirements under eCO2 will be satisfied and support continued biomass growth, even where roots are known to grow deeper (Iversen et al., 2011). This “fast in, fast out” C cycle in this mature nutrient-limited ecosystem under eCO2 will not necessarily release long-stored soil C to the atmosphere, but it is not likely to increase C sequestration by gaining additional plant biomass or soil C either; however, a recent meta-analysis assigning the short- and long-term effects of newly fixated C on soil C stocks indicated that any short-term gains of C into SOM could be gone after 1–4 years (van Groenigen et al., 2017).
There are several consistent trends of an increase in nutrient availability with eCO2 in this study, but they were not statistically significant. These variables include available inorganic N, the gross N mineralisation rate, inorganic P, and mineral-associated P. These trends in pools and processes may indicate an increase in both the nutrient availability and up-regulation, if mild, of processes responsible for increased nutrient availability. Though the mature Eucalyptus trees have not responded to eCO2 with aboveground biomass growth (Ellsworth et al., 2017), the understorey species composition has shifted to include more nutrient-demanding grasses with eCO2 (Hasegawa et al., 2018; Ochoa-Hueso et al., 2021). Higher-quality understorey litter may in turn drive increased nutrient availability in the soil (Berg and McClaugherty, 1989). Given the necessarily low replication, common to many FACE experiments (Filion et al., 2000), and the lower-than-expected enhancement of photosynthesis in this FACE system (Ellsworth et al., 2017; Pathare et al., 2017; Jiang et al., 2020), an eCO2 effect was expected to be statistically elusive, but here we do show that it can be discerned.
We found that nutrient availability and gross N mineralisation were always higher in rhizosphere soil compared to bulk soils, but enzymatic activity was not. The effect of depth, generally, caused a decrease in the available nutrients and process rates feeding into the available pools. However, the impact of roots and eCO2 counteracted the decrease found with depth when interactions between soil depth and CO2 or soil depth and soil type (bulk or rhizosphere) occurred. This response of lower concentrations found with increasing depth particularly affected available PO, adsorbed P, and the C : N and C : P enzyme activity. We can conclude that roots and eCO2 can affect available nutrient pools and processes well below the surface soil of a forest ecosystem, though it is not clear if the plants can benefit and take up nutrients from deeper parts of the soil profile. Our findings indicate a faster recycling of nutrients in the rhizosphere rather than additional nutrients becoming available through SOM decomposition. If the tree response to eCO2 is hindered or prevented by nutrient limitations, then the current results would question the potential for mature tree ecosystems to fix more C as biomass in response to eCO2. Future studies are encouraged to focus on how accessible the available nutrients at depth are to deeper rooted plants and if this fast recycling of nutrients is meaningful in the production of plant biomass and the accumulation of soil C response to eCO2.
Code and data presented in this work can be shared upon request.
The initial idea and experimental design were done by JP and YC, with support by CAM. The data were gathered by JP, with support from YC, CAM, and LCA. JP did the data management, statistical analysis, and wrote the paper with contributions from DSE, CAM, LCA and YC.
The contact author has declared that none of the authors has any competing interests.
Publisher's note: Copernicus Publications remains neutral with regard to jurisdictional claims in published maps and institutional affiliations.
This article is part of the special issue “Global change effects on terrestrial biogeochemistry at the plant–soil interface”. It is not associated with a conference.
The authors acknowledge the Dharug Nation as the traditional owners of the land on which EucFACE and Western Sydney University is located. We are thankful for support in the field and lab from Vinod Kumar, Craig McNamara, Norbert Klause, Elise Pendall, Jeff Powell, and Laura Castañeda-Gómez. The EucFACE facility was built as an initiative of the Australian Government as part of the Nation Building Economic Stimulus Package and is supported by the Australian Commonwealth in collaboration with Western Sydney University.
This research has been supported by the Australian Research Council (grant no. DP160102452) and the Svenska Forskningsrådet Formas (grant no. 2017-00423).
This paper was edited by Lucia Fuchslueger and reviewed by Nicholas Bouskill and Laynara F. Lugli.
Achat, D. L., Augusto, L., Gallet-Budynek, A., and Loustau, D.: Future challenges in coupled C–N–P cycle models for terrestrial ecosystems under global change: a review, Biogeochemistry, 131, 173–202, https://doi.org/10.1007/s10533-016-0274-9, 2016.
Adeleke, R., Nwangburuka, C., and Oboirien, B.: Origins, roles and fate of organic acids in soils: A review, South Afr. J. Bot., 108, 393–406, https://doi.org/10.1016/j.sajb.2016.09.002, 2017.
Ainsworth, E. A. and Long, S. P.: What have we learned from 15 years of free-air CO2 enrichment (FACE)? A meta-analytic review of the responses of photosynthesis, canopy properties and plant production to rising CO2, New Phytol., 165, 351–372, https://doi.org/10.1111/j.1469-8137.2004.01224.x, 2005.
Allison, S. D. and Vitousek, P. M.: Responses of extracellular enzymes to simple and complex nutrient inputs, Soil Biol. Biochem., 37, 937–944, https://doi.org/10.1016/j.soilbio.2004.09.014, 2005.
Andresen, L. C., Carrillo, Y., Macdonald, C. A., Castañeda-Gómez, L., Bodé, S., and Rütting, T.: Nitrogen dynamics after two years of elevated CO2 in phosphorus limited Eucalyptus woodland, Biogeochemistry, 150, 297–312, https://doi.org/10.1007/s10533-020-00699-y, 2020.
Atkinson, G.: A multivariate analysis of alluvial terrace soils of the clarendon and cranebrook formations, Nepean River, NSW, Soil Res., 26, 243–259, 1988.
Bates, D., Mächler, M., Bolker, B., and Walker, S.: Fitting Linear Mixed-Effects Models Using lme4, J. Stat. Soft., 67, 1–48, https://doi.org/10.18637/jss.v067.i01, 2015.
Bell, C. W., Fricks, B. E., Rocca, J. D., Steinweg, J. M., McMahon, S. K., and Wallenstein, M. D.: High-throughput Fluorometric Measurement of Potential Soil Extracellular Enzyme Activities, J. Visual. Exp., 81, 50961, https://doi.org/10.3791/50961, 2013.
Bengtson, P., Barker, J., and Grayston, S. J.: Evidence of a strong coupling between root exudation, C and N availability, and stimulated SOM decomposition caused by rhizosphere priming effects, Ecol. Evol., 2, 1843–1852, https://doi.org/10.1002/ece3.311, 2012.
Berg, B. and McClaugherty, C.: Nitrogen and phosphorus release from decomposing litter in relation to the disappearance of lignin, Can. J. Bot., 67, 1148–1156, https://doi.org/10.1139/b89-150, 1989.
Bernard, L., Basile-Doelsch, I., Derrien, D., Fanin, N., Fontaine, S., Guenet, B., Karimi, B., Marsden, C., and Maron, P.-A.: Advancing the mechanistic understanding of the priming effect on soil organic matter mineralization, Funct. Ecol., 36, 1355–1377, https://doi.org/10.1111/1365-2435.14038, 2022.
Bünemann, E. K.: Assessment of gross and net mineralization rates of soil organic phosphorus – A review, Soil Biol. Biochem., 89, 82–98, https://doi.org/10.1016/j.soilbio.2015.06.026, 2015.
Carrillo, Y., Dijkstra, F. A., LeCain, D., Morgan, J. A., Blumenthal, D., Waldron, S., and Pendall, E.: Disentangling root responses to climate change in a semiarid grassland, Oecologia, 175, 699–711, https://doi.org/10.1007/s00442-014-2912-z, 2014.
Carrillo, Y., Bell, C., Koyama, A., Canarini, A., Boot, C. M., Wallenstein, M., Pendall, E., and Vries, F.: Plant traits, stoichiometry and microbes as drivers of decomposition in the rhizosphere in a temperate grassland, J. Ecol., 105, 1750–1765, https://doi.org/10.1111/1365-2745.12772, 2017.
Castañeda-Gómez, L., Walker, J. K. M., Powell, J. R., Ellsworth, D. S., Pendall, E., and Carrillo, Y.: Impacts of elevated carbon dioxide on carbon gains and losses from soil and associated microbes in a Eucalyptus woodland, Soil Biol. Biochem., 143, 107734, https://doi.org/10.1016/j.soilbio.2020.107734, 2020.
Castañeda-Gómez, L., Powell, J. R., Ellsworth, D. S., Pendall, E., and Carrillo, Y.: The influence of roots on mycorrhizal fungi, saprotrophic microbes and carbon dynamics in a low-phosphorus Eucalyptus forest under elevated CO2, Funct. Ecol., 35, 2056–2071, 2021.
Crews, T. E., Kitayama, K., Fownes, J. H., Riley, R. H., Herbert, D. A., Mueller-Dombois, D., and Vitousek, P. M.: Changes in Soil Phosphorus Fractions and Ecosystem Dynamics across a Long Chronosequence in Hawaii, Ecology, 76, 1407–1424, https://doi.org/10.2307/1938144, 1995.
Crous, K. Y., Ósvaldsson, A., and Ellsworth, D. S.: Is phosphorus limiting in a mature Eucalyptus woodland? Phosphorus fertilisation stimulates stem growth, Plant Soil, 391, 293–305, https://doi.org/10.1007/s11104-015-2426-4, 2015.
De Graaff, M.-A., Groenigen, K.-J., Six, J., Hungate, B., and Kessel, C.: Interactions between plant growth and soil nutrient cycling under elevated CO2: a meta-analysis, Glob. Change Biol., 12, 2077–2091, https://doi.org/10.1111/j.1365-2486.2006.01240.x, 2006.
De Graaff, M.-A., Jastrow, J. D., Gillette, S., Johns, A., and Wullschleger, S. D.: Differential priming of soil carbon driven by soil depth and root impacts on carbon availability, Soil Biol. Biochem., 69, 147–156, https://doi.org/10.1016/j.soilbio.2013.10.047, 2014.
Dijkstra, F., Carrillo, Y., Pendall, E., and Morgan, J.: Rhizosphere priming: a nutrient perspective, Front. Microbiol., 4, 216, https://doi.org/10.3389/fmicb.2013.00216, 2013.
Drake, J. E., Gallet-Budynek, A., Hofmockel, K. S., Bernhardt, E. S., Billings, S. A., Jackson, R. B., Johnsen, K. S., Lichter, J., McCarthy, H. R., McCormack, M. L., Moore, D. J. P., Oren, R., Palmroth, S., Phillips, R. P., Pippen, J. S., Pritchard, S. G., Treseder, K. K., Schlesinger, W. H., DeLucia, E. H., and Finzi, A. C.: Increases in the flux of carbon belowground stimulate nitrogen uptake and sustain the long-term enhancement of forest productivity under elevated CO2, Ecol. Lett., 14, 349–357, https://doi.org/10.1111/j.1461-0248.2011.01593.x, 2011.
Drake, J. E., Macdonald, C. A., Tjoelker, M. G., Crous, K. Y., Gimeno, T. E., Singh, B. K., Reich, P. B., Anderson, I. C., and Ellsworth, D. S.: Short-term carbon cycling responses of a mature eucalypt woodland to gradual stepwise enrichment of atmospheric CO2 concentration, Glob. Change Biol., 22, 380–390, https://doi.org/10.1111/gcb.13109, 2016.
Ellsworth, D. S., Anderson, I. C., Crous, K. Y., Cooke, J., Drake, J. E., Gherlenda, A. N., Gimeno, T. E., Macdonald, C. A., Medlyn, B. E., Powell, J. R., Tjoelker, M. G., and Reich, P. B.: Elevated CO2 does not increase eucalypt forest productivity on a low-phosphorus soil, Nat. Clim. Change, 7, 279–282, https://doi.org/10.1038/nclimate3235, 2017.
Filion, M., Dutilleul, P., and Potvin, C.: Optimum experimental design for Free-Air Carbon dioxide Enrichment (FACE) studies, Glob. Change Biol., 6, 843–854, https://doi.org/10.1046/j.1365-2486.2000.00353.x, 2000.
Finzi, A. C., Abramoff, R. Z., Spiller, K. S., Brzostek, E. R., Darby, B. A., Kramer, M. A., and Phillips, R. P.: Rhizosphere processes are quantitatively important components of terrestrial carbon and nutrient cycles, Glob. Change Biol., 21, 2082–2094, https://doi.org/10.1111/gcb.12816, 2015.
Fisher, J. B., Badgley, G., and Blyth, E.: Global nutrient limitation in terrestrial vegetation, Global Biogeochem. Cy., 26, GB3007, https://doi.org/10.1029/2011GB004252, 2012.
Fontaine, S., Barot, S., Barre, P., Bdioui, N., Mary, B., and Rumpel, C.: Stability of organic carbon in deep soil layers controlled by fresh carbon supply, Nature, 450, 277–280, https://doi.org/10.1038/nature06275, 2007.
Fox, J. and Weisberg, S.: An R Companion to Applied Regression, 3rd Edn., Sage, Thousand Oaks CA, SAGE Publishing, ISBN: 9781544336466, 2019.
Gérard, F.: Clay minerals, iron/aluminum oxides, and their contribution to phosphate sorption in soils – A myth revisited, Geoderma, 262, 213–226, https://doi.org/10.1016/j.geoderma.2015.08.036, 2016.
Gilroy, S. and Jones, D. L.: Through form to function: root hair development and nutrient uptake, Trend. Plant Sci., 5, 56–60, https://doi.org/10.1016/S1360-1385(99)01551-4, 2000.
Hasegawa, S., Macdonald, C. A., and Power, S. A.: Elevated carbon dioxide increases soil nitrogen and phosphorus availability in a phosphorus-limited Eucalyptus woodland, Glob. Change Biol., 22, 1628–1643, https://doi.org/10.1111/gcb.13147, 2016.
Hasegawa, S., Piñeiro, J., Ochoa-Hueso, R., Haigh, A. M., Rymer, P. D., Barnett, K. L., and Power, S. A.: Elevated CO2 concentrations reduce C4 cover and decrease diversity of understorey plant community in a Eucalyptus woodland, J. Ecol., 106, 1483–1494, https://doi.org/10.1111/1365-2745.12943, 2018.
Hobley, E. U. and Wilson, B.: The depth distribution of organic carbon in the soils of eastern Australia, Ecosphere, 7, 01214, https://doi.org/10.1002/ecs2.1214, 2016.
Hothorn, T., Bretz, F., and Westfall, P.: Simultaneous Inference in General Parametric Models, Biometrical J., 50, 346–363, 2008.
Iversen, C. M.: Digging deeper: fine-root responses to rising atmospheric CO2 concentration in forested ecosystems, New Phytol., 186, 346–357, https://doi.org/10.1111/j.1469-8137.2009.03122.x, 2010.
Iversen, C. M., Ledford, J., and Norby, R. J.: CO2 enrichment increases carbon and nitrogen input from fine roots in a deciduous forest, New Phytol., 179, 837–847, https://doi.org/10.1111/j.1469-8137.2008.02516.x, 2008.
Iversen, C. M., Hooker, T. D., Classen, A. T., and Norby, R. J.: Net mineralization of N at deeper soil depths as a potential mechanism for sustained forest production under elevated CO2, Glob. Change Biol., 17, 1130–1139, https://doi.org/10.1111/j.1365-2486.2010.02240.x, 2011.
Jackson, R. B., Canadell, J., Ehleringer, J. R., Mooney, H. A., Sala, O. E., and Schulze, E. D.: A global analysis of root distributions for terrestrial biomes, Oecologia, 108, 389–411, https://doi.org/10.1007/BF00333714, 1996.
Jiang, M., Medlyn, B. E., Drake, J. E., Duursma, R. A., Anderson, I. C., Barton, C. V. M., Boer, M. M., Carrillo, Y., Castañeda-Gómez, L., Collins, L., Crous, K. Y., Kauwe, M. G., Santos, B. M., Emmerson, K. M., Facey, S. L., Gherlenda, A. N., Gimeno, T. E., Hasegawa, S., Johnson, S. N., Kännaste, A., Macdonald, C. A., Mahmud, K., Moore, B. D., Nazaries, L., Neilson, E. H. J., Nielsen, U. N., Niinemets, Ü., Noh, N. J., Ochoa-Hueso, R., Pathare, V. S., Pendall, E., Pihlblad, J., Piñeiro, J., Powell, J. R., Power, S. A., Reich, P. B., Renchon, A. A., Riegler, M., Rinnan, R., and Rymer: The fate of carbon in a mature forest under carbon dioxide enrichment, Nature, 580, 227–231, https://doi.org/10.1038/s41586-020-2128-9, 2020.
Jilling, A., Keiluweit, M., Contosta, A. R., Frey, S., Schimel, J., Schnecker, J., Smith, R. G., Tiemann, L., and Grandy, A. S.: Minerals in the rhizosphere: overlooked mediators of soil nitrogen availability to plants and microbes, Biogeochemistry, 139, 103–122, https://doi.org/10.1007/s10533-018-0459-5, 2018.
Jobbágy, E. G. and Jackson, R. B.: The distribution of soil nutrients with depth: Global patterns and the imprint of plants, Biogeochemistry, 53, 51–77, https://doi.org/10.1023/a:1010760720215, 2001.
Jones, D. L. and Darrah, P. R.: Role of root derived organic acids in the mobilization of nutrients from the rhizosphere, Plant Soil, 166, 247–257, https://doi.org/10.1007/bf00008338, 1994.
Kirkham, D. and Bartholomew, W. V.: Equations for Following Nutrient Transformations in Soil, Utilizing Tracer Data: II, Soil Sci. Soc. Am. J., 19, 189–192, https://doi.org/10.2136/sssaj1955.03615995001900020020x, 1955.
Kuzyakov, Y.: Priming effects: Interactions between living and dead organic matter, Soil Biol. Biochem., 42, 1363–1371, https://doi.org/10.1016/j.soilbio.2010.04.003, 2010.
Kuzyakov, Y. and Blagodatskaya, E.: Microbial hotspots and hot moments in soil: Concept & review, Soil Biol. Biochem., 83, 184–199, https://doi.org/10.1016/j.soilbio.2015.01.025, 2015.
Kuzyakov, Y. and Cheng, W.: Photosynthesis controls of rhizosphere respiration and organic matter decomposition, Soil Biol. Biochem., 33, 1915–1925, https://doi.org/10.1016/S0038-0717(01)00117-1, 2001.
Kuzyakov, Y., Friedel, J. K., and Stahr, K.: Review of mechanisms and quantification of priming effects, Soil Biol. Biochem., 32, 1485–1498, https://doi.org/10.1016/S0038-0717(00)00084-5, 2000.
Kuzyakov, Y., Horwath, W. R., Dorodnikov, M., and Blagodatskaya, E.: Review and synthesis of the effects of elevated atmospheric CO2 on soil processes: No changes in pools, but increased fluxes and accelerated cycles, Soil Biol. Biochem., 128, 66–78, https://doi.org/10.1016/j.soilbio.2018.10.005, 2019.
Laclau, J.-P., Silva, E., Rodrigues Lambais, G., Bernoux, M., le Maire, G., Stape, J. L., Bouillet, J.-P., Gonçalves, J. leonardo, Jourdan, C., and Nouvellon, Y.: Dynamics of soil exploration by fine roots down to a depth of 10 m throughout the entire rotation in Eucalyptus grandis plantations, Front. Plant Sci., 4, 243, https://doi.org/10.3389/fpls.2013.00243, 2013.
Lambers, H., Raven, J. A., Shaver, G. R., and Smith, S. E.: Plant nutrient-acquisition strategies change with soil age, Trends Ecol. Evol., 23, 95–103, https://doi.org/10.1016/j.tree.2007.10.008, 2008.
Lane, J. M., Delavaux, C. S., Van Koppen, L., Lu, P., Cade-Menun, B. J., Tremblay, J., and Bainard, L. D.: Soil sample storage conditions impact extracellular enzyme activity and bacterial amplicon diversity metrics in a semi-arid ecosystem, Soil Biol. Biochem., 175, 108858, https://doi.org/10.1016/j.soilbio.2022.108858, 2022.
Li, C., Zhao, L., Sun, P., Zhao, F., Kang, D., Yang, G., Han, X., Feng, Y., and Ren, G.: Deep Soil C, N, and P Stocks and Stoichiometry in Response to Land Use Patterns in the Loess Hilly Region of China, PLoS ONE, 11, e0159075, https://doi.org/10.1371/journal.pone.0159075, 2016.
Luo, Y., Su, B., Currie, W. S., Dukes, J. S., Finzi, A. C., Hartwig, U., Hungate, B., McMurtrie, R. E., Oren, R., Parton, W. J., Pataki, D. E., Shaw, M. R., Zak, D. R., and Field, C. B.: Progressive nitrogen limitation of ecosystem responses to rising atmospheric carbon dioxide, Bioscience, 54, 731–739, https://doi.org/10.1641/0006-3568(2004)054, 2004.
McGill, W. B. and Cole, C. V.: Comparative aspects of cycling of organic C, N, S and P through soil organic matter, Geoderma, 26, 267–286, https://doi.org/10.1016/0016-7061(81)90024-0, 1981.
Mooshammer, M., Wanek, W., Zechmeister-Boltenstern, S., and Richter, A.: Stoichiometric imbalances between terrestrial decomposer communities and their resources: mechanisms and implications of microbial adaptations to their resources, Front. Microbiol., 5, 22, https://doi.org/10.3389/fmicb.2014.00022, 2014.
Norby, R. J. and Zak, D. R.: Ecological Lessons from Free-Air CO2 Enrichment (FACE) Experiments, Ann. Rev. Ecol. Evol. Syst., 42, 181–203, https://doi.org/10.1146/annurev-ecolsys-102209-144647, 2011.
Ochoa-Hueso, R., Hughes, J., Delgado-Baquerizo, M., Drake, J. E., Tjoelker, M. G., Piñeiro, J., and Power, S. A.: Rhizosphere-driven increase in nitrogen and phosphorus availability under elevated atmospheric CO2 in a mature Eucalyptus woodland, Plant Soil, 1–13, https://doi.org/10.1007/s11104-017-3212-2, 2017.
Ochoa-Hueso, R., Piñeiro, J., Hasegawa, S., Illanas, S., Miranda, H., Reverter, M., and Power, S. A.: Spatial homogenization of understorey plant communities under eCO2 in a mature Eucalyptus woodland, J. Ecol., 109, 1386–1395, https://doi.org/10.1111/1365-2745.13564, 2021.
Ohno, T. and Zibilske, L. M.: Determination of Low Concentrations of Phosphorus in Soil Extracts Using Malachite Green, Soil Sci. Soc. Am. J., 55, 892–895, https://doi.org/10.2136/sssaj1991.03615995005500030046x, 1991.
Olander, L. P. and Vitousek, P. M.: Regulation of soil phosphatase and chitinase activityby N and P availability, Biogeochemistry, 49, 175–191, https://doi.org/10.1023/A:1006316117817, 2000.
Pathare, V. S., Crous, K. Y., Cooke, J., Creek, D., Ghannoum, O., and Ellsworth, D. S.: Water availability affects seasonal CO2-induced photosynthetic enhancement in herbaceous species in a periodically dry woodland, Glob. Change Biol., 23, 5164–5178, https://doi.org/10.1111/gcb.13778, 2017.
Phillips, R. P., Finzi, A. C., and Bernhardt, E. S.: Enhanced root exudation induces microbial feedbacks to N cycling in a pine forest under long-term CO2 fumigation, Ecol. Lett., 14, 187–194, https://doi.org/10.1111/j.1461-0248.2010.01570.x, 2011.
Piñeiro, J., Ochoa-Hueso, R., Drake, J. E., Tjoelker, M. G., and Power, S. A.: Water availability drives fine root dynamics in a Eucalyptus woodland under elevated atmospheric CO2 concentration, Funct. Ecol., 34, 2389–2402, https://doi.org/10.1111/1365-2435.13660, 2020.
Putz, M., Schleusner, P., Rütting, T., and Hallin, S.: Relative abundance of denitrifying and DNRA bacteria and their activity determine nitrogen retention or loss in agricultural soil, Soil Biol. Biochem., 123, 97–104, https://doi.org/10.1016/j.soilbio.2018.05.006, 2018.
Rasmussen, C., Heckman, K., Wieder, W. R., Keiluweit, M., Lawrence, C. R., Berhe, A. A., Blankinship, J. C., Crow, S. E., Druhan, J. L., Hicks Pries, C. E., Marin-Spiotta, E., Plante, A. F., Schädel, C., Schimel, J. P., Sierra, C. A., Thompson, A., and Wagai, R.: Beyond clay: towards an improved set of variables for predicting soil organic matter content, Biogeochemistry, 137, 297–306, https://doi.org/10.1007/s10533-018-0424-3, 2018.
Rayment, G. E. and Lyons, D. J.: Soil Chemical Methods: Australasia, CSIRO Publishing, ISBN: 9780643101364, 2011.
Ross, G. M., Horn, S., Macdonald, C. A., Powell, J. R., Reynolds, J. K., Ryan, M. M., Cook, J. M., and Nielsen, U. N.: Metabarcoding mites: Three years of elevated CO2 has no effect on oribatid assemblages in a Eucalyptus woodland, Pedobiologia, 81–82, 150667, https://doi.org/10.1016/j.pedobi.2020.150667, 2020.
Rumpel, C. and Kögel-Knabner, I.: Deep soil organic matter – a key but poorly understood component of terrestrial C cycle, Plant Soil, 338, 143–158, https://doi.org/10.1007/s11104-010-0391-5, 2011.
Rütting, T., Clough, T. J., MÜLler, C., Lieffering, M., and Newton, P. C. D.: Ten years of elevated atmospheric carbon dioxide alters soil nitrogen transformations in a sheep-grazed pasture, Glob. Change Biol., 16, 2530–2542, https://doi.org/10.1111/j.1365-2486.2009.02089.x, 2010.
Rütting, T., Huygens, D., Staelens, J., Müller, C., and Boeckx, P.: Advances in 15N-tracing experiments: new labelling and data analysis approaches, Biochem. Soc. Trans., 39, 279–283, https://doi.org/10.1042/bst0390279, 2011.
Schimel, D., Stephens, B. B., and Fisher, J. B.: Effect of increasing CO2 on the terrestrial carbon cycle, P. Natl. Acad. Sci. USA, 112, 436–441, https://doi.org/10.1073/pnas.1407302112, 2015.
Sinsabaugh, R. L., Lauber, C. L., Weintraub, M. N., Ahmed, B., Allison, S. D., Crenshaw, C., Contosta, A. R., Cusack, D., Frey, S., Gallo, M. E., Gartner, T. B., Hobbie, S. E., Holland, K., Keeler, B. L., Powers, J. S., Stursova, M., Takacs-Vesbach, C., Waldrop, M. P., Wallenstein, M. D., Zak, D. R., and Zeglin, L. H.: Stoichiometry of soil enzyme activity at global scale, Ecol. Lett., 11, 1252–1264, https://doi.org/10.1111/j.1461-0248.2008.01245.x, 2008.
Sinsabaugh, R. L., Hill, B. H., and Follstad Shah, J. J.: Ecoenzymatic stoichiometry of microbial organic nutrient acquisition in soil and sediment, Nature, 462, 795–798, https://doi.org/10.1038/nature08632, 2009.
Sistla, S. A. and Schimel, J. P.: Stoichiometric flexibility as a regulator of carbon and nutrient cycling in terrestrial ecosystems under change, New Phytol., 196, 68–78, https://doi.org/10.1111/j.1469-8137.2012.04234.x, 2012.
Spohn, M.: Element cycling as driven by stoichiometric homeostasis of soil microorganisms, Basic Appl. Ecol., 17, 471–478, https://doi.org/10.1016/j.baae.2016.05.003, 2016.
Spohn, M. and Widdig, M.: Turnover of carbon and phosphorus in the microbial biomass depending on phosphorus availability, Soil Biol. Biochem., 113, 53–59, https://doi.org/10.1016/j.soilbio.2017.05.017, 2017.
Stange, F. C., Spott, O., Apelt, B., and Russow, R. W. B.: Automated and rapid online determination of 15N abundance and concentration of ammonium, nitrite, or nitrate in aqueous samples by the SPINMAS technique, Isotop. Environ. Health Stud., 43, 227–236, https://doi.org/10.1080/10256010701550658, 2007.
Terrer, C., Vicca, S., Stocker, B. D., Hungate, B. A., Phillips, R. P., Reich, P. B., Finzi, A. C., and Prentice, I. C.: Ecosystem responses to elevated CO2 governed by plant–soil interactions and the cost of nitrogen acquisition, New Phytol., 217, 507–522, https://doi.org/10.1111/nph.14872, 2018.
Terrer, C., Jackson, R. B., Prentice, I. C., Keenan, T. F., Kaiser, C., Vicca, S., Fisher, J. B., Reich, P. B., Stocker, B. D., Hungate, B. A., Peñuelas, J., McCallum, I., Soudzilovskaia, N. A., Cernusak, L. A., Talhelm, A. F., Sundert, K., Piao, S., Newton, P. C. D., Hovenden, M. J., Blumenthal, D. M., Liu, Y. Y., Müller, C., Winter, K., Field, C. B., Viechtbauer, W., Lissa, C. J., Hoosbeek, M. R., Watanabe, M., Koike, T., Leshyk, V. O., Polley, H. W., and Franklin, O.: Nitrogen and phosphorus constrain the CO2 fertilization of global plant biomass, Nat. Clim. Change, 9, 684–689, https://doi.org/10.1038/s41558-019-0545-2, 2019.
Vance, E. D., Brookes, P. C., and Jenkinson, D. S.: An extraction method for measuring soil microbial biomass C, Soil Biol. Biochem., 19, 703–707, https://doi.org/10.1016/0038-0717(87)90052-6, 1987.
van Groenigen, K. J., Osenberg, C. W., Terrer, C., Carrillo, Y., Dijkstra, F. A., Heath, J., Nie, M., Pendall, E., Phillips, R. P., and Hungate, B. A.: Faster turnover of new soil carbon inputs under increased atmospheric CO2, Glob. Chang Biol., 23, 4420–4429, https://doi.org/10.1111/gcb.13752, 2017.
Walker, T. W. and Syers, J. K.: The fate of phosphorus during pedogenesis, Geoderma, 15, 1–19, https://doi.org/10.1016/0016-7061(76)90066-5, 1976.
Wang, Z. and Wang, C.: Magnitude and mechanisms of nitrogen-mediated responses of tree biomass production to elevated CO2: A global synthesis, J. Ecol., 109, 4038–4055, https://doi.org/10.1111/1365-2745.13774, 2021.
Zhao, F., Zhang, L., Sun, J., Ren, C., HAN, X., Yang, G., Pang, G., Bai, H., and Wang, J.: Effect of Soil C, N and P Stoichiometry on Soil Organic C Fractions After Afforestation, Pedosphere, 27, 705–713, https://doi.org/10.1016/S1002-0160(17)60479-X, 2017.
Zhu, B., Gutknecht, J. L. M., Herman, D. J., Keck, D. C., Firestone, M. K., and Cheng, W.: Rhizosphere priming effects on soil carbon and nitrogen mineralization, Soil Biol. Biochem., 76, 183–192, https://doi.org/10.1016/j.soilbio.2014.04.033, 2014.