the Creative Commons Attribution 4.0 License.
the Creative Commons Attribution 4.0 License.
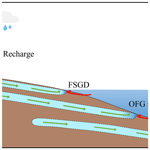
Ideas and perspectives: Land–ocean connectivity through groundwater
Damian L. Arévalo-Martínez
Hermann W. Bange
Ercan Erkul
Marion Jegen
Nils Moosdorf
Jens Schneider von Deimling
Christian Berndt
Michael Ernst Böttcher
Jasper Hoffmann
Volker Liebetrau
Ulf Mallast
Gudrun Massmann
Aaron Micallef
Holly A. Michael
Hendrik Paasche
Wolfgang Rabbel
Isaac Santos
Jan Scholten
Katrin Schwalenberg
Beata Szymczycha
Ariel T. Thomas
Joonas J. Virtasalo
Hannelore Waska
Bradley A. Weymer
For millennia, humans have gravitated towards coastlines for their resource potential and as geopolitical centres for global trade. A basic requirement ensuring water security for coastal communities relies on a delicate balance between the supply and demand of potable water. The interaction between freshwater and saltwater in coastal settings is, therefore, complicated by both natural and human-driven environmental changes at the land–sea interface. In particular, ongoing sea-level rise, warming and deoxygenation might exacerbate such perturbations. In this context, an improved understanding of the nature and variability of groundwater fluxes across the land–sea continuum is timely yet remains out of reach. The flow of terrestrial groundwater across the coastal transition zone and the extent of freshened groundwater below the present-day seafloor are receiving increased attention in marine and coastal sciences because they likely represent a significant yet highly uncertain component of (bio)geochemical budgets and because of the emerging interest in the potential use of offshore freshened groundwater as a resource. At the same time, “reverse” groundwater flux from offshore to onshore is of prevalent socio-economic interest, as terrestrial groundwater resources are continuously pressured by over-pumping and seawater intrusion in many coastal regions worldwide. An accurate assessment of the land–ocean connectivity through groundwater and its potential responses to future anthropogenic activities and climate change will require a multidisciplinary approach combining the expertise of geophysicists, hydrogeologists, (bio)geochemists and modellers. Such joint activities will lay the scientific basis for better understanding the role of groundwater in societally relevant issues such as climate change, pollution and the environmental status of the coastal oceans within the framework of the United Nations Sustainable Development Goals. Here, we present our perspectives on future research directions to better understand land–ocean connectivity through groundwater, including the spatial distributions of the essential hydrogeological parameters, highlighting technical and scientific developments and briefly discussing the societal relevance of that connectivity in rapidly changing coastal oceans.
- Article
(2501 KB) - Full-text XML
- BibTeX
- EndNote
This article is dedicated to Dr. Volker Liebetrau (1965–2022). Volker, our co-author and friend, passed away on 7 February 2022. Volker is remembered for his enthusiasm, commitment, and smile. His passing is a massive loss to the community, but there will be much that will still be carried on in his memory thanks to his hard work and passion.
The exchange of groundwater between land and ocean is a wide-spread phenomenon, which has significant impacts on the biogeochemical cycles of the coastal ocean (e.g. Church, 1996; Moore, 2010; Santos et al., 2021). Coastal margins play a disproportionally important role for marine ecosystems compared to the open ocean due to their greater biological productivity, sediment–water interactions and air–sea transfer of climate-relevant trace gases (Liu et al., 2010). Increasing anthropogenic activities result in high nutrient fluxes into the coastal ocean, leading to eutrophication, deoxygenation and the release of greenhouse gases, which in turn could exacerbate the current global warming trend and significantly affect the livelihood of nations that rely on coastal ecosystem services (e.g. Van Meter et al., 2018; Oehler et al., 2021; Rocha et al., 2021). In addition, accelerating global sea-level rise (GSLR) can negatively influence terrestrial coastal aquifers due to the inland displacement of the fresh-saline-water interfaces, referred to as saltwater intrusion (SWI; Ferguson and Gleeson, 2012; Taylor et al., 2013). Sea-level rise at regional and local scales can modify the chemical balance within subterranean estuaries and increase their spatial extent by enhancing SWI, which results in enhanced load of e.g. nutrients and pollutants to the coastal ocean (Moore and Joye, 2021). In turn, increased human usage of groundwater resources is estimated to account for approximately 14 % of the observed GSLR through a net transfer of freshwater from deep reservoirs into the ocean (Konikow, 2011; Church et al., 2013; Taylor et al., 2013). Increasing usage of non-renewable groundwater might further exacerbate global water depletion (Bierkens and Wada, 2019), which is further impacted by climate variability through changes in recharge and precipitation (Thomas and Famiglietti, 2019; Beebe et al., 2022).
The cross-shelf extension of terrestrial coastal groundwater systems can be distinguished into two key (often interrelated) elements (see Table 1 and Fig. 1). The first comprises meteoric groundwater flux from terrestrial aquifers through the seabed (including the intertidal zone) into the coastal ocean, which is generally referred to as fresh submarine groundwater discharge (FSGD; e.g. Kohout, 1964; Taniguchi et al., 2002, 2019). The second consists of large (>10 km horizontal extent) freshened (and often brackish) groundwater reservoirs embedded in sediment and rocks below the present-day seafloor, collectively called offshore freshened groundwater (OFG; Post et al., 2013).
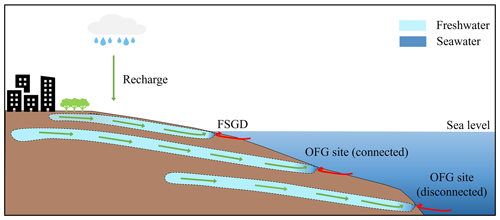
Figure 1Schematic representation of known pathways for the transport and storage of fresh and freshened groundwater between terrestrial and marine realms. Areas surrounded by dashed lines indicate groundwater reservoirs, whereas arrows represent freshwater (green) and seawater (red) fluxes. Based on Bratton et al. (2010) and Weymer et al. (2020).
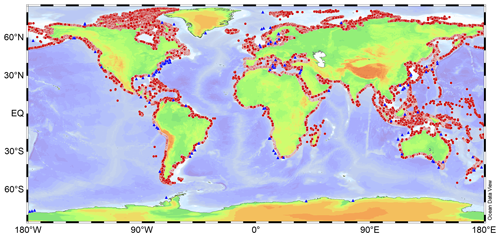
Figure 2Global distribution of reported FSGD (red circles) and OFG (blue triangles) sites. Location data of FSGD and OFG from Luijendijk et al. (2020) and Micallef (2020), respectively.
FSGD connects terrestrial groundwater systems to the coastal ocean on most coastlines in the world (Fig. 2; Luijendijk et al., 2020). FSGD is essentially the surplus of the terrestrial water budget. Most known FSGD occurs within the first few 100 m from the coast, although its occurrence has also been reported at tens to hundreds of kilometres offshore (Manheim, 1967; Kooi and Groen, 2001; Bratton, 2010). Given the large degree of spatio-temporal variability in FSGD, estimates of regional and global fluxes are still highly uncertain (Taniguchi et al., 2019). Globally, FSGD accounts for 1 %–10 % of the global freshwater input to the ocean (Abbott et al., 2019; Luijendijk et al., 2020). Locally, however, FSGD can be key for sustaining some marine ecosystems (Luijendijk et al., 2020).
Similar to FSGD, OFG has been observed in shelf sediments throughout the world's oceans (Fig. 2; Post et al., 2013; Micallef et al., 2021). Likewise, OFG is a potential freshwater resource, or a resource of water that can be treated with desalination with comparably small energy consumption (Bakken et al., 2012), and has therefore gained increased attention over the past decade (Post et al., 2013; Micallef et al., 2021). Although OFG is generally a relic of past sea-level low stand (fossil groundwater), some reservoirs are likely hydraulically connected to the terrestrial aquifers groundwater system, as shown for the US Atlantic coast (Gustafson et al., 2019; Thomas et al., 2019); Canterbury Bight, New Zealand (Micallef et al., 2020; Weymer et al., 2020); and the Achziv submarine canyon in northern Israel (Paldor et al., 2020). Here, we emphasize the importance of improving our understanding of connected OFG, since its extraction as an unconventional resource for mitigating temporal water scarcity in coastal communities might cause seawater intrusion (Yu and Michael, 2019a) and distant land subsidence (Chen et al., 2007; Yu and Michael, 2019b).
With ongoing research in near-coastal groundwater fluxes (FSGD) and offshore reservoirs (OFG) being carried out by largely different scientific communities, we address unexploited scientific and technical synergies between them. The reliance on markedly different methodologies leads to differences in scientific language and, in turn, conceptually disconnects the research of both phenomena (FSGD is usually assessed using geochemical tracers and hydrological observations from the intertidal zone or numerical groundwater modelling (Taniguchi et al., 2019; Luijendijk et al., 2020), whereas OFG studies often require ship-based geophysical methods (Micallef et al., 2021)). Here, we present new perspectives on future research directions to improve the understanding of land–ocean connectivity through groundwater, with a particular focus on joint activities of FSGD and OFG research communities. This includes (i) improving our quantitative understanding of the distribution and variability of groundwater fluxes at regional and global scales; (ii) assessing long-term changes in groundwater sources and their expected impact on marine environments, as well as their potential usage; and (iii) evaluating conceptual and technological developments which will potentially advance joint FSGD–OFG research.
2.1 Fresh submarine groundwater discharge
The interaction between saline and fresh groundwater in coastal regions is governed by complex processes, e.g. density contrasts between fresh and saline water, tidal effects and geological heterogeneity (Michael et al., 2016; Jiao and Post, 2019). Saline groundwater can intrude landward, salinizing terrestrial aquifers (resulting in SWI). Yet, at the same time, terrestrial groundwater can cross the land–sea continuum and appear offshore as FSGD and/or OFG (Fig. 2; see e.g. Whiticar, 2002; Post et al., 2013; Jurasinski et al., 2018; Micallef et al., 2020). Groundwater flow is associated with external forcing (e.g. groundwater heads, framework geology, onshore groundwater usage, sea level) that dictates the hydrostatic gradient causing fluxes to be directed inland, offshore or both. Strong distortions of hydraulic gradients can influence or even reverse groundwater flow, which, in turn, might have widespread consequences for pelagic and benthic marine ecosystems (e.g. Donis et al., 2017; Lecher and Mackey, 2018; Szymczycha et al., 2020; Santos et al., 2021), as well as for associated services such as fisheries, because both nutrients and contaminants are transported into the coastal ocean via groundwater. A recent study estimated the global input of groundwater into the ocean via FSGD to be less than 1 % of the surface-water runoff. However, on local scales, FSGD can reach 25 % of the river flux (Luijendijk et al., 2020), and saline SGD releases recycled nutrients at rates comparable to global rivers (Santos et al., 2021). The high spatial variability of this influx is partly controlled by climate at a regional scale and partly by lithological heterogeneities at a local scale (Sawyer et al., 2016). Because the extrapolation of point-scale measurements onto a regional, continental or global scale is difficult, FSGD quantification heavily relies on hydrogeological modelling (Moosdorf et al., 2021), which can result in great uncertainties on large spatial scales.
2.2 Offshore freshened groundwater
OFG resides beneath the seafloor along continental shelves and, in contrast to FSGD, is commonly assumed to have lower groundwater flow velocities (e.g. Micallef et al., 2020). Recent estimates report OFG to comprise a volume of approximately 1×106 km3 (Micallef et al., 2021), which is about 10 % of the Earth's liquid fresh water (Shlklomanov, 1993). Different OFG emplacement mechanisms have been proposed, of which meteoric recharge, sub- and proglacial injection, diagenesis and the decomposition of gas hydrates are the most significant (Micallef et al., 2021). OFG systems may be coupled with FSGD (e.g. Paldor et al., 2020; Attias et al., 2021), and modelling shows that FSGD and OFG can occur in equilibrium with present-day sea level for a range of different stratigraphic configurations (Michael et al., 2016). However, OFG can also be decoupled from interaction with the water column (see e.g. Micallef et al., 2020). Post et al. (2013) compiled a global estimation of OFG sites based mainly on borehole observations. Geophysical technologies have updated these global estimates through the detection of OFG residing within siliciclastic continental margins in the United States and New Zealand (Gustafson et al., 2019; Micallef et al., 2020), along a carbonate coastline in Malta (Haroon et al., 2021) and offshore from the volcanic islands of Hawaii (Attias et al., 2020). These studies have improved our understanding of spatial OFG distributions but do not bridge the knowledge gap between coastal nearshore and offshore hydrological systems. To date, continuous tracing of terrestrial aquifers along the full onshore–offshore gradient remains technically challenging (Weymer et al., 2020), and observation strategies need to be developed for specific settings. Geophysical methods employed as imaging tools to characterize the subsurface offer promising avenues towards bridging the information gap across the land–sea interface, although they are only currently available on local scales (e.g. Siemon et al., 2020; Ishizu and Ogawa, 2021). Hydrologically connected OFG systems should in principle be associated with discharging groundwater (see e.g. Weymer et al., 2020), either close to the coastline, along faults or other lithological discontinuities, or at distant locations near the shelf break. However, OFG could also seep into the marine environment on time scales of > 100–1000 kyr, making it difficult to obtain observations that provide insights into its effects on biological communities if no dedicated offshore drilling is carried out.
In the terrestrial realm, the role of coastal groundwater as a habitat (Pohlman, 2011; Leitão et al., 2015; Adyasari et al., 2019) and in shaping pelagic and benthic coastal communities (Lecher and Mackey, 2018; Oberle et al., 2022) has become increasingly recognized. In contrast, the role of OFG as a fresh- or brackish-water habitat within a purely marine environment remains unknown. Hence, its investigation might constitute a new frontier in ocean sciences in view of its potential exploitation as an unconventional source of water.
Human use of OFG could affect both fresh groundwater discharging into the ocean and groundwater hydraulic heads on land. FSGD has local ecological impacts on e.g. seagrass (Carruthers et al., 2005), corals (Oehler et al., 2019; Correa et al., 2021; Oberle et al., 2022), phytoplankton (Rodellas et al., 2015; Sugimoto et al., 2017; Waska and Kim, 2010), molluscs (Hwang et al., 2010), meio- and macrofauna (Zipperle and Reise, 2005; Kotwicki et al., 2014; Grzelak et al., 2018; Londoño-Londoño et al., 2022a), and fish populations (Fujita et al., 2019; Pisternick et al., 2020). These influences are often triggered by nutrient and carbon inputs into the submarine environment (Santos et al., 2021; Böttcher et al., 2023). Moreover, upward fluid migration within soft seafloor sediments might fluidize them, favouring the formation of pockmarks that potentially release greenhouse gases such as carbon dioxide and methane (Whiticar, 2002; Judd and Hovland, 2009; Donis et al., 2017; Virtasalo et al., 2019; Hoffmann et al., 2020; Purkamo et al., 2022). While some of these effects have been perceived as a threat for ecosystems – for instance, by inducing toxic algal blooms, adding alkalinity (Cabral et al., 2021) or harbouring dense microbial communities (Ionescu et al., 2012) – they can also sustain coastal ecology and increase fishery yields. Pumping OFG that is associated with FSGD could reduce the associated landward reservoirs and eventually impact the coastal marine environment. Moreover, anthropogenic intervention on coastal sediments might impact benthic–pelagic coupling associated with FSGD (von Ahn et al., 2021).
Considering the manifold biogeochemical impacts of FSGD, it is difficult to assess the overall effect of different pumping approaches and particular FSGD locations in local marine ecosystems should a connected OFG be exploited. Pumping water from a groundwater system means reducing the formation pressure. The reduced pressure can communicate with the terrestrial aquifer and reduce the hydraulic head there (Yu and Michael, 2019b). The extent of this effect will depend on the reservoir properties and on the hydraulic connectivity between the terrestrial and offshore domains, which might in turn lead to SWI (Ferguson and Gleeson, 2012; Yu and Michael, 2019a), groundwater depletion (Bierkens and Wada, 2019) and subsidence (Yu and Michael, 2019b). Despite the large uncertainties regarding the global and long-term effects of these changes on groundwater resources and associated marine ecosystems, lessons may be learnt from the environmental effects of extensive oil exploration (Varma and Michael, 2012; Chaussard et al., 2013).
Changes in FSGD volume and its chemical and/or biological composition could serve as an important indicator for changes in the coastal groundwater system, which could, in turn, also be caused by connectivity with OFG. FSGD can be a source of geochemical tracers (e.g. Ra and Rn; Kim and Hwang, 2002), inorganic nutrients (nitrate, phosphate and silicate; e.g. Waska et al., 2011; Szymczycha et al., 2012), trace metals (e.g. Knee and Paytan, 2011), climate-relevant trace gases (carbon dioxide, nitrous oxide, methane and carbon monoxide; e.g. Bugna et al., 1996; Chapelle and Bradley, 2007; Jurado et al., 2017; Kolker et al., 2021; Reading et al., 2021) and organic material (e.g. dissolved organic matter; see Kim and Kim, 2017, and McDonough et al., 2022) for coastal areas. The input of nutrients results in an FSGD-driven eutrophication of coastal areas and, thus, potentially affects coastal ecosystems (Luijendijk et al., 2020; Oehler et al., 2021; Santos et al., 2021). For example, large outbreaks of the macroalgae Ulva spp. (so-called “green tides”), which occur regularly in eutrophic coasts off China and Korea, are attributed to the nutrients supplied by FSGD (Kwon et al., 2017; Liu et al., 2017; Cho et al., 2019; Zhao et al., 2021). Hence, sustained monitoring of the biogeochemical and microbially driven transformations of key biogeochemical tracers within the subterranean estuary, as well as their release to the overlying water column, might help track changes in FSGD. Furthermore, such monitoring might also facilitate investigating potential impacts on the productivity and ecological status of coastal environments. Beyond coastal nearshore environments, FSGD seems to play an important role for biogeochemical fluxes to the ocean and affects benthic and subseafloor ecosystems in more offshore coastal areas (Micallef et al., 2021 and references therein). Therefore, a thorough investigation of its dynamics in different oceanic basins and geological settings should be performed by future studies.
Furthermore, FSGD and connected OFG could be increasingly affected by ongoing environmental changes on the terrestrial side (e.g. by changing rain patterns or intensity (Thomas and Famiglietti, 2019), eutrophication (derived from increasing applications of fertilizers), urbanization of coastal areas and associated contamination with microplastics (Viaroli et al., 2022), chemical (e.g. pesticides, pharmaceuticals and personal care products; see Knee and Paytan, 2011; Szymczycha et al., 2020), and biological pollutants (bacteria and viruses, including pathogenic species; see e.g. Kyle et al., 2008; Sorensen et al., 2021)). In particular, the effects of FSGD-driven inputs of chemical and/or biological pollutants on coastal areas remain largely unknown.
Various techniques are available to explore and identify FSGD and OFG in the offshore environment (e.g. Micallef et al., 2021) and groundwater resources on the land side (Kirsch, 2006). These techniques often reveal anomalies in the subsurface, the seafloor (e.g. pockmarks) or the seawater column (e.g. salinity, geochemical tracers) associated with fresh groundwater. Often, multiple techniques are applied to build confidence in the interpretation of groundwater dynamics. The technologies used can be broadly categorized into four groups: (i) geophysical imaging techniques, which detect or record physical parameters such as electrical resistivity, seismic velocity, density, temperature, or structural or morphological surface anomalies; (ii) hydrogeological approaches, including modelling and hydrological measurements (e.g. hydraulic heads, salinity and recharge rates); (iii) (bio)geochemical techniques, which analyse (bio)geochemical fingerprints of the fluids; and (iv) (micro)biological sampling, which unravels biological diversity and processes associated with FSGD (see e.g. Taniguchi et al., 2019; Micallef et al., 2021; Ruiz-González et al., 2021, and references therein). These multiple approaches are often mastered by researchers within different disciplinary backgrounds, including geophysics, hydrology, oceanography and biogeochemistry.
Assessing land–ocean hydraulic connectivity through groundwater requires investigating the connectivity of underlying lithologic units and their hydrological characterization. Moreover, it also requires identifying the current distribution of freshened groundwater bodies across the coastline. The occurrence of freshened groundwater along the onshore–offshore continuum may in turn be read from geochemical fingerprinting of fluid samples obtained from the different realms. Hence, the success of such a highly interdisciplinary endeavour in mapping and understanding the connectivity will depend on how well the different methodologies can be integrated. Here, we suggest overarching approaches in which synergies (both conceptual and technological) between FSGD and OFG scientific communities could contribute to an improved understanding of the dynamics of groundwater as a connecting path between land and the ocean at the coastal zone. Table 2 presents some of the most commonly used methods in groundwater studies, for which we foresee promising synergies between FSGD and OFG research.
Table 2Commonly used methods for investigating groundwater fluxes and reservoirs.
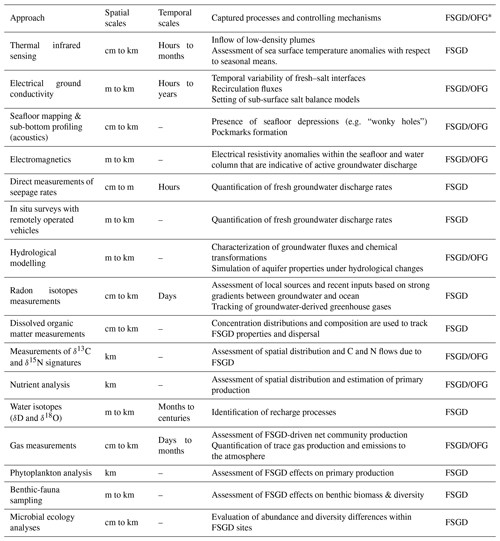
∗ Current application realm.
4.1 Shoreline-crossing lithologies
Seismic reflection imaging is the method of choice for detailed subsurface mapping. Particular lithologies may be identified by the character of the seismic reflection data within a lithological unit, e.g. layered seismic facies for fine-grained marine sediments vs. chaotic patterns for coarser-grained sediments (Thomas et al., 2019; Micallef et al., 2020). Co-located boreholes on seismic sections can greatly improve the identification of different facies and serve as calibration points along those sections. Of particular importance for shoreline-crossing groundwater dynamics is the possibility for seismic data to constrain the continuity of different lithological units, the presence of impermeable clay layers and faults, or other disrupting geological structures. However, seismic information in the transition zone near the coastline is not widely available due to logistical challenges for data acquisition. Land and marine seismic data have inherently different signal-to-noise ratios and imaging depths, making across-shoreline interpretation challenging. On land there are often more boreholes than there are offshore, which can provide data to constrain the lithology distribution. Through the integration of onshore and offshore seismic and borehole data using geostatistical methods such as sequential indicator simulation or multiple-point geostatistics (Deutsch and Pyrcz, 2014), lithology distribution across the shoreline can be modelled to reduce the uncertainty regarding connected pathways between the terrestrial and offshore domains.
Land and marine seismic data require different seismic sources, e.g. vibroseis on land and airguns or sparkers at sea, and receivers. Noise levels are generally higher on land, whereas offshore imaging in the transition zone is hampered by seafloor multiple reflections due to the shallow water depth. Generally, clastic sedimentary environments are easier to image than carbonate systems (e.g. Mountain, 2008; Lofi et al., 2013; Bertoni et al., 2020). Amphibious data acquisition, i.e. across the shoreline, is possible and can be accomplished in different ways, for instance by shooting on land and receiving at sea or vice versa. Yet, due to logistical challenges and greater expenses, amphibious sections are not a standard. In karstic carbonate or volcanic systems, the spatial occurrence of localized submarine springs (rather than the diffuse discharge in siliciclastic systems) can help to characterize the onshore–offshore connectivity of aquifers (Bayari et al., 2011).
Ground-penetrating radar (GPR) is suitable for detailed, near-surface lithological imaging on land. The GPR technique is based on an electromagnetic signal that is sensitive to sediment water content. Offshore, hydro-acoustic and seismic methods provide structural information from shallow to deeper depths but are insensitive to the water content. However, unconformable boundaries of subsurface sediment units are typically imaged as strong reflectors in both GPR and reflection seismic profiles due to the associated sharp changes in water content and density, respectively, which permits the cross-shore correlation of onshore GPR profiles with marine seismic profiles using the allostratigraphic approach (see e.g. Virtasalo et al., 2019; Peterson et al., 2020).
4.2 Identification of groundwater bodies
While seismic data can reveal the geological background and are – to some extent – sensitive to the porosity of the rock, they contain no information on pore fluid salinity. The salinity of pore fluids can be explored using electrical methods because the bulk electrical resistivity of a sediment rock is governed by the amount (fluid-saturated pore space) and salinity of fluid present (Archie, 1942; Keller, 1987). The better the porosity of the lithology is known, for example through seismic or lithological data, the better the pore-space fluid saturation on land and the pore water salinity offshore can be assessed from bulk electrical resistivity measurements. A bulk electrical resistivity model of the subsurface can be derived from either direct- or alternating-current electrical measurements (electromagnetic induction), where the latter allows for larger penetration depths and better resolution offshore.
On land, the highest data acquisition speed and therefore the largest areal coverage is achieved through airborne electromagnetic methods (e.g. Bedrosian et al., 2016; Gottschalk et al., 2020; Siemon et al., 2020). Additional ground measurements using direct-current and controlled-source electromagnetic methods provide bulk electrical-resistivity models of the subsurface at higher resolutions and larger depths of penetration (e.g. Pondthai et al., 2020). Resolution in surveys of electrical resistivity on land can be augmented by conducting GPR surveys. GPR methods allow both the detection of contrasts in the electrical-conductivity structure (dielectric constant) contained in coastal sediments at high resolutions (cm to m scales) and the effective mapping of the freshwater–saltwater interface at shallow depths (up to tens of metres; Weymer et al., 2020).
Offshore, a freshened groundwater body can be identified as an electrical-resistivity anomaly caused by the resistivity contrast between fresh and saline pore water. The conductive saline ocean above the seafloor strongly dampens electromagnetic signals, which renders airborne electromagnetic systems incapable of penetrating the seafloor at water depths larger than about 10–20 m (Goebel et al., 2019). Therefore, offshore measurements require specially adapted marine electromagnetic systems. So far, OFG exploration studies have been conducted using surface-towed (e.g. Gustafson et al., 2019; Attias et al., 2021) and/or seafloor-towed (Haroon et al., 2018, 2021; Micallef et al., 2020) systems. Both systems consist of a horizontal electric source dipole followed by several electric receiving dipoles recording the inline electric field. Offsets between transmitter and receiving dipoles typically range between hundreds and several hundreds of metres and can be adjusted according to the target depth. Sea-surface-towed systems have the advantage of a greater acquisition speed, though this is with the cost of a lower resolution and larger source dipole moments (current amplitude times dipole length) being required to compensate for the decay of the source signal in the conductive ocean layer. Seafloor-towed systems have arguably better signal-to-noise ratios and resolution, although their survey speed is much lower, and surveying is hampered by rough seafloor topography and infrastructure. Onshore–offshore acquisition with a land transmitter and offshore receiver is possible (Ishizu and Ogawa, 2021). However, to date there are no peer-reviewed published studies which use this approach. Merging a separately acquired onshore–offshore electrical resistivity section with land and marine systems is possible, although a coherent continuous picture may be hampered by different resolutions, penetration depths, noise levels and the strong 3D-resistivity contrast at the shoreline (“coast effect”; Worzewski et al., 2012). Recently, joint land–water data inversion methods have become available to amend that deficit (Hermans and Paepen, 2020). Furthermore, conversion of electrical-resistivity sections to water saturation on land or pore water salinity (the actual target parameters) requires integration of lithological data, i.e. bulk porosity estimates, and the appropriate choice of an effective medium model.
In situ sampling techniques are effective and simple – albeit labour- and time-intensive – ways to detect freshened groundwater. These methods include pore water extraction using push-point samplers along transects or grids (Waska et al., 2019), in situ detection of springs with infrared cameras (Röper et al., 2014) and collection of seeping groundwater with seepage metres or benthic chambers (e.g. Lee, 1977; Donis et al., 2017). Although mostly applied to nearshore groundwater discharge, all the above-mentioned methods are adaptable to remote systems – for instance, on stationary landers or ROVs (e.g. Ahmerkamp et al., 2017).
4.3 Groundwater flow
Imaging of coastal aquifers using inversion of geophysical data constrained by groundwater transport simulations is a promising method which might greatly reduce uncertainty in FSGD rates and location (Costall et al., 2020). Other promising approaches to detect FSGD over a larger area (tens of kilometres) while also allowing an assessment of its temporal variability are thermal-radiance measurements with manned (e.g. Roxburgh, 1985; Johnson et al., 2008) or unmanned (e.g. Fischer et al., 1964; Dulai et al., 2016; Lee et al., 2016; Mallast and Siebert, 2019) aerial and sea-going vehicles, as well as usage of satellite-based thermal infrared imagery (Londoño-Londoño et al., 2022b).
While geophysical methods provide the geological background and current state of onshore–offshore groundwater distribution (Weymer et al., 2015), they do not capture the dynamics and functioning of the system, which are essential to determining and understanding the nature of land–sea hydrologic connectivity. Physical hydrological measurements are essential for understanding groundwater flow rates and patterns. In coastal systems with variations in fluid density, this involves characterizing groundwater head distributions and associated hydraulic gradients, as well as the salinity distributions. On land, this is typically done with measurements from groundwater wells in addition to geophysics. Offshore, these measurements are more challenging but provide critical information on the forces driving fluid flow through the onshore–offshore system. Because offshore hydrologic data are generally sparse, groundwater modelling is an essential tool to test hypotheses about system function given the geological, hydrological and biogeochemical data available. Groundwater models that incorporate physics-based variable-density flow and salt transport and that capture the essential characteristics of the system (e.g. interconnection of geologic strata; Michael et al., 2016; Thomas et al., 2022) can be used to understand the long-timescale evolution of OFG systems towards their current state (e.g. Cohen et al., 2010; Micallef et al., 2020; Zamrsky et al., 2021) and to predict changes under expected changes in sea level and anthropogenic forcing (Yu and Michael, 2019a, b). These models can characterize both the flow in the subsurface and also the rate and distribution of FSGD and/or diffusive transport processes.
In conjunction with geophysical and hydrological data and analyses, the geochemistry of groundwater fluid samples can provide key information about the origin and age of FSGD and OFG. A combination of stable-isotope and conservative-tracer analysis (e.g. Hoefs, 2009; Dang et al., 2020) can be used to identify sources and to estimate ages of offshore groundwater bodies, i.e. recent or fossil meteoric water (van Geldern et al., 2013), glacial meltwater (Hong et al., 2019), or methane hydrate dissociation (Dählmann and De Lange, 2003). While onshore fluid samples required for this analysis are relatively easily obtained (typically from groundwater observation wells), OFG fluid sample collection requires in situ sampling at depth through a borehole or, if existing, knowledge of FSGD occurrences on the seafloor. FSGD sites on the seafloor can be identified through detection of morphologic depressions (pockmarks, sinkholes) through high-frequency acoustic seafloor bathymetry mapping and identification of anomalous seafloor fauna and flora associated with a change in water salinity and nutrients input (e.g. Lecher and Mackey, 2018; Archana et al., 2021). Other approaches used to search FSGD sites on a regional scale include mapping radiogenic isotopes that are associated with groundwater (Burnett et al., 2006; Paldor et al., 2020; Ikonen et al., 2022); shallow physical imaging of resistivity anomalies; surveys of small-scale magnetic-susceptibility anomalies caused by preservation or diagenetic alteration of iron oxides in sediments (Müller et al., 2011); satellite infrared imagery using e.g. Landsat 8 – infrared (e.g. Wilson and Rocha, 2012; Schubert et al., 2014; Jou-Claus et al., 2021); and surface-reaching fault mapping by seismic methods.
FSGD may also cause measurable anomalies in the deeper water column of offshore sites (Manheim, 1967; Attias et al., 2021). While temperature and salinity anomalies are only measurable in the immediate vicinity of the FSGD location and may be obscured by natural variations in water temperature and by tidal currents, radon and radium anomalies can be traced to larger distances (e.g. Cable et al., 1996; Moore et al., 2011). This methodology works well in areas of diffuse and uniform FSGD, but it might overlook localized point sources, which can account for up to 90 % of FSGD in karstic regions (Null et al., 2014).
In view of the increasing pressure of human activities and natural changes on groundwater resources, the fundamental role of land–ocean connectivity through groundwater in the dynamics of coastal systems requires a critical reassessment. FSGD and the associated fluxes of biogeochemical tracers might affect the physical structure, chemical composition and reactivity, as well as the (micro)biology, of coastal ocean ecosystems. Global and regional environmental changes (i.e. warming, eutrophication, acidification and pollution) modify processes in coastal groundwater and thereby FSGD, with largely unknown consequences for coastal marine ecosystems. Exploitation of OFG connected to terrestrial groundwater is expected to impact terrestrial groundwater flow systems. These feedback mechanisms operate over a wide range of spatial and temporal scales, ranging from molecular to global and from millisecond to millennial. Thus, an overarching goal of future coastal groundwater research should be the development of a suite of ecosystem models of land–ocean connectivity that include the physical, geological, chemical and biological processes at play and that address potential responses to dynamic interactions between nature and humans.
Within this framework, we recommend the following priority research tasks:
-
assess and compare the spatio-temporal variability of physical and biogeochemical processes driving the dynamics of FSGD and OFG in different geological settings;
-
characterize and quantify the geochemical and/or biological composition of FSGD and OFG, as well as its impacts on marine habitats and (micro)biological communities;
-
develop an interdisciplinary framework including hydrological, geophysical, geochemical and (micro)biological measurements to delineate groundwater fluxes (FSGD) and to map reservoirs (OFG) along the transition from nearshore to offshore systems;
-
characterize the stratigraphy at the land–ocean interface to determine the potential for development of connected, active OFG systems;
-
identify, quantify and predict feedbacks between coastal groundwater dynamics and climate change to assess potential changes in the volume and composition of FSGD and OFG;
-
use numerical models and artificial intelligence to predict locations, magnitudes and connectivity of FSGD and OFG; and
-
implement the knowledge gained through models and observations to improve the representation of FSGD and OFG in Earth system models.
Investigation of the land–ocean connectivity through groundwater beyond nearshore FSGD remains especially challenging because of its limited accessibility and large heterogeneity. Its future study will require representative and standardized sampling, the development of new analytical methods (e.g. in situ offshore groundwater measurements), and new observational and experimental frameworks. These endeavours should facilitate fully representative parameterizations of FSGD–OFG connectivity in numerical models across the land–sea interface. Moreover, developing hydrologic and/or oceanographic models of coastal and offshore groundwater and its interactions with other system compartments (sediments, water column, subseafloor environments) will help predict future changes of groundwater dynamics on both regional and global scales.
It is evident that only multidisciplinary research initiatives at local, national and international levels can effectively address the research tasks identified in this perspective paper. Joint projects should link laboratory, field and modelling approaches to better understand the complex interplay of the various physical, chemical and biological processes operating along the land–ocean interface. Likewise, sustained observations will help to amend the current uncertainties in the temporal variability of groundwater flows. An improved understanding of land–ocean connectivity in this context will contribute to our appreciation of the crucial role of coastal groundwater in societally relevant issues such as climate change, pollution and the overall environmental status of the coastal oceans. Future research efforts on this topic will directly address the Sustainable Development Goals 6 (“Clean water and sanitation”), 12 (“Responsible consumption and production”) and 14 (“Life below water”) of the United Nations (see https://www.un.org/sustainabledevelopment/sustainable-development-goals/, last access: 27 January 2023).
The data from the Micallef et al. (2021) study in Fig. 2 are freely available at Zenodo: https://doi.org/10.5281/zenodo.4247833 (Micallef, 2020). The data from Luijendijk et al. (2020) are freely available in the Supplement to that article.
The initial draft of this perspectives paper was prepared by DLAM, AH, HWB, EE, MJ, NM and JSvD. All co-authors contributed to the revision and preparation of the final version of the paper.
The contact author has declared that none of the authors has any competing interests.
Publisher’s note: Copernicus Publications remains neutral with regard to jurisdictional claims in published maps and institutional affiliations.
The conception of this paper was fostered by the discussions during a workshop sponsored by the Future Ocean Network of Kiel University (https://www.futureocean.org/en/, last access: 23 January 2023) in February 2021. The paper contains contributions to the KiSNet Network funded by the German Research Foundation (DFG; grant no. MA7041/6-1), the DFG research unit “DynaDeep” (grant no. FOR 5094/1) and the DFG research training group Baltic TRANSCOAST (grant no. GRK2000/0063). Aaron Micallef acknowledges funding from the European Research Council (ERC) under the European Union's Horizon 2020 research and innovation programme (grant no. 677898 (MARCAN)).
Damian L. Arévalo-Martínez was supported by the Future Ocean Network (grant no. FON2020-03). Additional support was provided by the SMART (Sustainable management of offshore groundwater resources) project funded by the Helmholtz Association.
The article processing charges for this open-access publication were covered by the GEOMAR Helmholtz Centre for Ocean Research Kiel.
This paper was edited by Ben Bond-Lamberty and reviewed by Ana Silva and three anonymous referees.
Abbott, B. W., Bishop, K., Zarnetske, J. P., Minaudo, C., Chapin, F. S., Krause, S., Hannah, D. M., Conner, L., Ellison, D., Godsey, E. S., Plont, S., Marçais, J., Kolbe, T., Huebner, A., Frei, R. J., Hampton, T., Gu, S., Buhman, M., Sara Sayedi, S., Ursache, O., Chapin, M., Henderson, K. D., and Pinay, G.: Human domination of the global water cycle absent from depictions and perceptions, Nat. Geosci., 12, 533–540, 2019.
Adyasari, D., Hassenrück, C., Oehler, T., Sabdaningsih, A., and Moosdorf, N.: Microbial community structure associated with submarine groundwater discharge in northern Java (Indonesia), Sci. Total Environ., 689, 590–601, 2019.
Ahmerkamp, S., Winter, C., Krämer, K., Beer, D. d., Janssen, F., Friedrich, J., Kuypers, M. M. M., and Holtappels, M.: Regulation of benthic oxygen fluxes in permeable sediments of the coastal ocean, Limnol. Oceanogr., 62, 1935–1954, https://doi.org/10.1002/lno.10544, 2017.
Archana, A., Francis, C. A., and Boehm, A. B.: The Beach Aquifer Microbiome: Research Gaps and Data Needs, Front. Environ. Sci., 9, 653568, https://doi.org/10.3389/fenvs.2021.653568, 2021.
Archie, G. E.: The Electrical Resistivity Log as an Aid in Determining Some Reservoir Characteristics, Transactions of AIME, 146, 54–62, 1942.
Attias, E., Thomas, D., Sherman, D., Ismail, K., and Constable, S.: Marine electrical imaging reveals novel freshwater transport mechanism in Hawai'i, Sci. Adv., 6, eabd4866, https://doi.org/10.1126/sciadv.abd4866, 2020.
Attias, E., Constable, S., Sherman, D., Ismail, K., Shuler, C., and Dulai, H.: Marine electromagnetic imaging and volumetric estimation of freshwater plumes offshore Hawai'i, Geophys. Res. Lett., 48, e2020GL091249, https://doi.org/10.1029/2020GL091249, 2021.
Bakken, T. H., Ruden, F., and Mangset, L. E.: Submarine groundwater: A new concept for the supply of drinking water, Water Resour. Manag., 26, 1015–1026, https://doi.org/10.1007/s11269-011-9806-1, 2012.
Bayari, C. S., Ozyurt, N. N., Oztan, M., Bastanlar, Y., Varinlioglu, G., Koyuncu, H., Ulkenli, H., and Hamarat, S.: Submarine and coastal karstic groundwater discharges along the southwestern Mediterranean coast of Turkey, Hydrogeol. J., 19, 399–414, https://doi.org/10.1007/s10040-010-0677-y, 2011.
Bedrosian, P. A., Schamper, C., and Auken, E.: A comparison of helicopter-borne electromagnetic systems for hydrogeologic studies, Geophys. Prospect., 64, 192–215, https://doi.org/10.1111/1365-2478.12262, 2016.
Beebe, D. A., Huettemann, M. B., Webb, B. M., and Jackson Jr., W. T.: Atmospheric groundwater forcing of a subterranean estuary: A seasonal seawater recirculation process, Geophys. Res. Lett., 49, e2021GL096154, https://doi.org/10.1029/2021GL096154, 2022.
Bertoni, C., Lofi, J., Micallef, A., and Moe, H.: Seismic reflection methods in offshore groundwater research, Geosciences, 10, 299, https://doi.org/10.3390/geosciences10080299, 2020.
Bierkens, M. F. P. and Wada, Y.: Non-renewable groundwater use and groundwater depletion: a review, Environ. Res. Lett., 14, 063002, https://doi.org/10.1088/1748-9326/ab1a5f, 2019.
Böttcher, M. E., Mallast, U., Massmann, G., Moosdorf, N., Müller-Petke, M., and Waska, H.: Coastal-Groundwater interfaces (submarine groundwater discharge), in: Ecohydrological Interfaces, edited by: Krause, S., Hannah, D. M., and Grimm, N., Wiley Science, 400 pp., ISBN 978-1119489672, 2023.
Bratton, J. F.: The Three Scales of Submarine Groundwater Flow and Discharge across Passive Continental Margins, J. Geol., 118, 565–575, 2010.
Bugna, G., Chanton, J. P., Young, J. E., Burnett, W. C., and Cable, P. H.: The importance of groundwater discharge to the methane budgets of nearshore and continental shelf waters of the northeastern Gulf of Mexico, Geochim. Cosmochim. Ac., 60, 4735–4746, https://doi.org/10.1016/S0016-7037(96)00290-6, 1996.
Burnett, W. C., Aggarwal, P. K., Aureli, A., Bokuniewicz, H., Cable, J. E., Charette, M. A., Kontar, E., Krupa, S., Kulkarni, K. M., Loveless, A., Moore, W. S., Oberdorfer, J. A., Oliveira, J., Ozyurt, N., Povinec, P., Privitera, A. M. G., Rajar, R., Ramassur, R. T., Scholten, J., Stieglitz, T., Taniguchi, M., and Turner, J. V.: Quantifying submarine groundwater discharge in the coastal zone via multiple methods, Sci. Total Environ., 367, 498–543, 2006.
Cable, J. E., Burnett, W. C., Chanton, J. P., and Weatherly, G. L.: Estimating groundwater discharge into the northeastern Gulf of Mexico using radon-222, Earth Plan. Sc. Lett., 144, 591–604, https://doi.org/10.1016/S0012-821X(96)00173-2, 1996.
Cabral, A., Dittmar, T., Call, M., Scholten, J., de Rezende, C. E., Asp, N., Gledhill, M., Seidel, M., and Santos, I. R.: Carbon and alkalinity outwelling across the groundwater-creek-shelf continuum off Amazonian mangroves, Limmnol. Oceanogr. Lett., 6, 369–378, 2021.
Carruthers, T. J. B., van Tussenbroek, B. I., and Dennison, W. C.: Influence of submarine springs and wastewater on nutrient dynamics of Caribbean seagrass meadows, Estuar. Coast. Shelf S., 64, 191–199, 2005.
Chapelle, F. H. and Bradley, P. M.: Hydrologic significance of carbon monoxide concentrations in groundwater, Groundwater, 45, 272–280, 2007.
Chaussard, E., Amelung, F., Abidin, H., and Hong, S.-H.: Sinking cities in Indonesia: ALOS PALSAR detects rapid subsidence due to groundwater and gas extraction, Remote Sens. Environ., 128, 150–161, https://doi.org/10.1016/j.rse.2012.10.015, 2013.
Chen, C.-T., Hu, J.-C., Lu, C.-Y., Lee, J.-C., and Chan, Y.-C.: Thirty-year land elevation change from subsidence to uplift following the termination of groundwater pumping and its geological implications in the Metropolitan Taipei Basin, Northern Taiwan, Eng. Geol., 95, 30–47, 2007.
Cho, H.-M., Kim, G., and Shin, K.-H.: Tracing nitrogen sources fueling coastal green tides off a volcanic island using radon and nitrogen isotopic tracers, Sci. Total Environ., 665, 913–919, 2019.
Church, J. A., Clark, P. U., Cazenave, A., Gregory, J. M., Jevrejeva, S., Levermann, A., Merrifield, M. A., Milne, G. A., Nerem, R. S., Nunn, P. D., Payne, A. J., Pfeffer, W. T., Stammer, D., and Unnikrishnan, A. S.: Sea Level Change, in: Climate Change 2013: The Physical Science Basis. Contribution of Working Group I to the Fifth Assessment Report of the Intergovernmental Panel on Climate Change, edited by: Stocker, T. F., Qin, D., Plattner, G.-K., Tignor, M., Allen, S. K., Boschung, J., Nauels, A., Xia, Y., Bex, V., and Midgley, P. M., 1137–1216, Cambridge University Press, Cambridge, United Kingdom and New York, NY, USA, 2013.
Church, T. M.: A groundwater route for the water cycle, Nature, 380, 579–580, 1996.
Cohen, D., Person, M., Wang, P., Gable, C. W., Hutchinson, D., Marksamer, A., Dugan, B., Kooi, H., Groen, K., Lizarralde, D., Evans, R. L., Day-Lewis, F. D., and Lane Jr., J. W.: Origin and extent of fresh paleowaters on the Atlantic continental shelf, USA, Groundwater, 48, 143–158, 2010.
Correa, R. E., Cardenas, M. B., Rodolfo, R. S., Lapus, M. R., Davis, K. L., Giles, A. B., Fullon, J. C., Hajati, M.-C., Moosdorf, N., Sanders, C. J., and Santos, I. R.: Submarine Groundwater Discharge Releases CO2 to a Coral Reef, ACS ES&T Water, 1, 1756–1764, 2021.
Costall, A. R., Harris, B. D., Teo, B., Schaa, R., Wagner, F. M., and Pigois, J. P.: Groundwater Throughflow and Seawater Intrusion in High Quality Coastal Aquifers, Sci. Rep., 10, 9866, https://doi.org/10.1038/s41598-020-66516-6, 2020.
Dählmann, A. and de Lange, G. J.: Fluid-sediment interactions at Eastern Mediterranean mud volcanoes: A stable isotope study from ODP Leg 160, Earth Plan. Sc. Lett., 212, 377–391, https://doi.org/10.1016/S0012-821X(03)00227-9, 2003.
Dang, X., Gao, M., Wen, Z., Jakada, H., Hou, G., and Liu, S.: Evolutionary process of saline groundwater influenced by palaeo-seawater trapped in coastal deltas: A case study in Luanhe River Delta, China, Estuar. Coast. Shelf S., 244, 106894, https://doi.org/10.1016/j.ecss.2020.106894, 2020.
Deutsch, C. V. and Pyrcz, M. (Eds.): Geostatistical reservoir modeling, 2nd Edn., Oxford University Press, Oxford, 448 pp., ISBN 978-0199731442, 2014.
Donis, D., Janssen, F., Liu, B., Wenzhöfer, F., Dellwig, O., Escher, P., Spitzy, A., and Böttcher, M. E.: Biogeochemical impact of submarine ground water discharge on coastal surface sands of the southern Baltic Sea, Estuar. Coast. Shelf S., 189, 131–142, 2017.
Dulai, H., Kamenik, J., Waters, C. A., Kennedy, J., Babinec, J., Jolly, J., and Williamson, M.: Autonomous long-term gamma-spectrometric monitoring of submarine groundwater discharge trends in Hawaii, J. Radioanal. Nucl. Ch., 307, 1865–1870, https://doi.org/10.1007/s10967-015-4580-9, 2016.
Ferguson, G. and Gleeson, T.: Vulnerability of coastal aquifers to groundwater use and climate change, Nat. Clim. Change, 2, 342–345, https://doi.org/10.1038/nclimate1413, 2012.
Fischer, W. A., Landis, G. H., Moxham, R. M., and Polcyn, F.: Infrared Surveys of Hawaiian Volcanoes – Aerial Surveys with Infrared Imaging Radiometer Depict Volcanic Thermal Patterns + Structural Features, Science, 146, 733–742, 1964.
Fujita, K., Shoji, J., Sugimoto, R., Nakajima, T., Honda, H., Takeuchi, M., Tominaga, O., and Taniguchi, M.: Increase in Fish Production Through Bottom-Up Trophic Linkage in Coastal Waters Induced by Nutrients Supplied via Submarine Groundwater, Front. Environ. Sci., 7, 82, https://doi.org/10.3389/fenvs.2019.00082, 2019.
Goebel, M., Knight R., and Halkjaer, M.: Mapping saltwater intrusion with an airborne electromagnetic method in the offshore coastal environment, Monterey Bay, California, J. Hydrol.: Reg. Stud., 23, 100602, https://doi.org/10.1016/j.ejrh.2019.100602, 2019.
Gottschalk, I., Knight, R., Asch, T., Abraham, J., and Cannia, J.: Using an airborne electromagnetic method to map saltwater intrusion in the northern Salinas Vallet, California, Geophysics, 85, B119–B131, https://doi.org/10.1190/geo2019-0272.1, 2020.
Grzelak, K., Tamborski, J., Kotwicki, L., and Bokuniewicz, H.: Ecostructuring of marine nematode communities by submarine groundwater discharge, Mar. Environ. Res., 136, 106–119, https://doi.org/10.1016/j.marenvres.2018.01.013, 2018.
Gustafson, C., Key, K., and Evans, R. L.: Aquifer systems extending far offshore on the U.S. Atlantic margin, Sci. Rep., 9, 8709, https://doi.org/10.1038/s41598-019-44611-7, 2019.
Haroon, A., Lippert, K., Mogilatov, V., and Tezkan, B.: First application of the marine differential electric dipole for groundwater investigations: A case study from Bat Yam, Israel, Geophysics, 83, B59–B76, 2018.
Haroon, A., Micallef, A., Jegen, M., Schwalenberg, K., Karstens, J., Berndt, C., Garcia, X., Kühn, M., Rizzo, E., Fusi, N. C., Ahaneku, C. V., Petronio, L., Faghih, Z., Weymer, B. A., De Biase, M., and Chidichimo, F.: Electrical resistivity anomalies offshore a carbonate coastline: Evidence for freshened groundwater?, Geophys. Res. Lett., 48, e2020GL091909, https://doi.org/10.1029/2020GL091909, 2021.
Hermans, T. and Paepen, M.: Combined inversion of land and marine electrical resistivity tomography for submarine groundwater discharge and saltwater intrusion characterization, Geophys. Res. Lett., 47, e2019GL085877, https://doi.org/10.1029/2019GL085877, 2020.
Hoefs, J.: Stable isotope geochemistry, 203 pp., Springer, Berlin/Heidelberg, ISBN 978-3-540-70708-0, 2009.
Hoffmann, J. J. L., Schneider von Deimling, J., Schröder, J., Schmidt, M., Scholten, J., Crutchley, G. J., and Gorman, A. R.: Complex eyed pockmarks and submarine groundwater discharge revealed by acoustic data and sediment cores in Eckernförde Bay, SW Baltic Sea, Geochem. Geophy. Geosy., 21, e2019GC008825, https://doi.org/10.1029/2019GC008825, 2020.
Hong, W.‐L., Lepland, A., Himmler, T., Kim, J. H., Chand, S., Sahy, D., Solomon, E. A., Rae, J. W. B., Martma, T., Nam, S.-I., and Knies, J.: Discharge of meteoric water in the eastern Norwegian Sea since the last glacial period, Geophys. Res. Lett., 46, 8194–8204, https://doi.org/10.1029/2019GL084237, 2019.
Hwang, D. W., Kim, G., Lee, W. C., and Oh, H. T.: The role of submarine groundwater discharge (SGD) in nutrient budgets of Gamak Bay, a shellfish farming bay, in Korea, J. Sea Res., 64, 224–230, 2010.
Ionescu, D., Siebert, C., Polerecky, L., Munwes, Y. Y., Lott, C., Häusler, S., Bižić-Ionescu, M. Quast, C., Peplies, J., Glöckner F. O., Ramette, A., Rödiger, T., Dittmar, T., Oren, A., Geyer, S., Stärk, H.-J., Sauter, M., Licha, T., Laronne, J. B., and de Beer, D.: Microbial and Chemical Characterization of Underwater Fresh Water Springs in the Dead Sea, PLoS ONE, 7, e38319, https://https://doi.org/10.1371/journal.pone.0038319, 2012.
Ishizu, K. and Ogawa, Y.: Offshore-onshore resistivity imaging of freshwater using a controlled-source electromagnetic method: A feasibility study, Geophysics, 86, E391–E405, https://doi.org/10.1190/geo2020-0906.1, 2021.
Jiao, J. and Post, V. (Eds.): Coastal Hydrogeology, Cambridge University Press, Cambridge, https://doi.org/10.1017/9781139344142, 2019.
Johnson, A. G., Glenn, C. R., Burnett, W. C., Peterson, R. N., and Lucey, P. G.: Aerial infrared imaging reveals large nutrient-rich groundwater inputs to the ocean, Geophys. Res. Lett., 35, 6, https://doi.org/10.1029/2008GL034574, 2008.
Jou-Claus, S., Folch, A., and Garcia-Orellana, J.: Applicability of Landsat 8 thermal infrared sensor for identifying submarine groundwater discharge springs in the Mediterranean Sea basin, Hydrol. Earth Syst. Sci., 25, 4789–4805, https://doi.org/10.5194/hess-25-4789-2021, 2021.
Judd, A. and Hovland, M. (Eds.): Seabed fluid flow: The impact on geology, biology and the marine environment, 492 pp., Cambridge, Cambridge University Press, ISBN 978-0521114202, 2009.
Jurado, A., Borges, A. V., and Brouyère, S.: Dynamics and emissions of N2O in groundwater: A review, Sci. Total Environ., 584–585, 207–218, 2017.
Jurasinski, G., Janssen, M., Voss, M., Böttcher, M. E., Brede, M., Burchard, H., Forster, S., Gosch, L., Gräwe, U., Gründling-Pfaff, S., Haider F., Ibenthal, M., Karow, N., Karsten, U., Kreuzburg, M., Lange, X., Leinweber, P., Massmann, G., Ptak, T., Rezanezhad F., Rehder, G., Romoth, K., Schade, H., Schubert, H., Schulz-Vogt, H., Sokolova, I. M., Strehse, R., Unger, V., Westphal, J., and Lennartz, B.: Understanding the Coastal ecocline: Assessing sea-land-interactions at non-tidal, low-lying coasts through interdisciplinary research, Front. Mar. Sci., 5, 1–22, https://doi.org/10.3389/fmars.2018.00342, 2018.
Keller, G. V.: Rock and Mineral Properties, in: Electromagnetic Methods in Applied Geophysics, edited by: Nabighian, M., 1, II. Series: Investigations in Geophysics, SEG, ISBN 1-56080-069-0, 1987.
Kim, G. and Hwang, D.-W.: Tidal pumping of groundwater into the coastal ocean revealed from submarine 222Rn and CH4 monitoring, Geophys. Res. Lett., 29, 1678, https://doi.org/10.1029/2002GL015093, 2002.
Kim, J. and Kim, G.: Inputs of humic fluorescent dissolved organic matter via submarine groundwater discharge to coastal waters off a volcanic island (Jeju, Korea), Sci. Rep., 7, 7921, https://doi.org/10.1038/s41598-017-08518-5, 2017.
Kirsch, R. (Ed.): Groundwater Geophysics, A Tool for Hydrogeology, Springer ISBN 10 3-540-29383-3, 2006.
Knee, K. L. and Paytan, A.: Submarine Groundwater Discharge: A source of nutrients, metals, and pollutants to the coastal ocean, in: Treatise on Estuarine and Coastal Science, edited by: Wolanski, E. and McLusky, D. S., Vol. 4, 205–233, Academic Press, Waltham, https://doi.org/10.1016/B978-0-12-374711-2.00410-1, 2011.
Kohout, F. A.: The flow of fresh water and salt water in the Biscayne Bay Aquifer of the Miami area, Florida, in: Sea Water in Coastal Aquifers, edited by: Cooper, H. H., Kohout, F. A., Henry, H. R., and Glover, R. E., Geological survey water-supply paper, 1613-C, USGS, Washington, D.C., 12–32, 1964.
Kolker, D., Bookman, R., Herut, B., David, N., and Silverman, J.: An initial assessment of the contribution of fresh submarine ground water discharge to the alkalinity budget of the Mediterranean Sea, J. Geophys. Res.-Oceans, 126, e2020JC017085, https://doi.org/10.1029/2020JC017085, 2021.
Konikow, L. F.: Contribution of global groundwater depletion since 1900 to sea-level rise, Geophys. Res. Lett., 38, L17401, https://doi.org/10.1029/2011GL048604, 2011.
Kooi, H. and Groen, K.: Offshore continuation of coastal groundwater systems; predictions using sharp-interface approximations and variable-density flow modelling, J. Hydrol., 246, 19–35, https://doi.org/10.1016/S0022-1694(01)00354-7, 2001.
Kotwicki, L., Grzelak, K., Czub, M., Dellwig, O., Gentz, T., Szymczycha, B., and Böttcher, M. E.: Submarine groundwater discharge to the Baltic coastal zone: Impacts on the meiofaunal community, J. Marine Syst., 129, 118–126, 2014.
Kwon, H. K., Kang, H., Oh, Y. H., Park, S. R., and Kim, G.: Green tide development associated with submarine groundwater discharge in a coastal harbor, Jeju, Korea, Sci. Rep., 7, 6325, https://doi.org/10.1038/s41598-017-06711-0, 2017.
Kyle, J., Eydal, H., Ferris, F., and Pedersen, K.: Viruses in granitic groundwater from 69 to 450 m depth of the Äspö hard rock laboratory, Sweden, ISME J., 2, 571–574, https://doi.org/10.1038/ismej.2008.18, 2008.
Ikonen, J., Hendriksson, N., Luoma, S., Lahaye, Y., and Virtasalo, J. J.: Behavior of Li, S and Sr isotopes in the subterranean estuary and seafloor pockmarks of the Hanko submarine groundwater discharge site in Finland, northern Baltic Sea, Appl. Geochem., 147, 105471, https://doi.org/10.1016/j.apgeochem.2022.105471, 2022.
Lecher, A. and Mackey, K.: Synthesizing the effects of submarine groundwater discharge on Marine Biota, Hydrology, 5, 60, https://doi.org/10.3390/hydrology5040060, 2018.
Lee, D. R.: A device for measuring seepage flux in lakes and estuaries, Limnol. Oceanogr., 22, 140–147, 1977.
Lee, E., Yoon, H., Hyun, S. P., Burnett, W. C., Koh, D. C., Ha, K., Kim, D.-J., Kim, Y., and Kang, K.-M.: Unmanned aerial vehicles (UAVs)-based thermal infrared (TIR) mapping, a novel approach to assess groundwater discharge into the coastal zone, Limnol. Oceanogr., 14, 725–735, https://doi.org/10.1002/lom3.10132, 2016.
Leitão, F., Encarnação, J., Range, P., Schmelz, R. M., Teodósio, M. A., and Chícharo, L.: Submarine groundwater discharges create unique benthic communities in a coastal sandy marine environment, Estuar. Coast. Shelf S., 163, 93–98, 2015.
Liu, J., Su, N., Wang, X., and Du, J.: Submarine groundwater discharge and associated nutrient fluxes into the Southern Yellow Sea: A case study for semi-enclosed and oligotrophic seas – implication for green tide bloom, J. Geophys. Res.-Oceans, 122, 139–152, https://doi.org/10.1002/2016JC012282, 2017.
Liu, K.-K., Atkinson, L., Quiñones, R., and Talaue-McManus, L. (Eds.): Carbon and nutrient fluxes in continental margins: A global synthesis, Springer, Berlin & Heidelberg, 741 pp., 2010.
Lofi, J., Pezard, P., Bouchette, F., Raynal, O., Sabatier, P., Denchik, N., Levannier, A., Dezileau, L., and Certain, R.: Integrated onshore-offshore investigation of a Mediterranean layered coastal aquifer, Groundwater, 51, 550–561, 2013.
Londoño-Londoño, J. E., Condesso de Melo, M. T., and Silva, A. C. F.: Groundwater discharge locally shapes the rocky shore macroinvertebrate community in South-Southwest Portugal, Mar. Environ. Res., 179, 105672, https://doi.org/10.1016/j.marenvres.2022.105672, 2022a.
Londoño-Londoño, J. E., Condesso de Melo, M. T., Nascimento, J. N., and Silva, A. C. F.: Thermal-Based Remote Sensing Solution for Identifying Coastal Zones with Potential Groundwater Discharge, J. Mar. Sci. Eng., 10, 414, https://doi.org/10.3390/jmse10030414, 2022b.
Luijendijk, E., Gleeson, T., and Moorsdorf, N.: Fresh groundwater discharge insignificant for the world's oceans but important for coastal ecosystems, Nat. Commun., 11, 1260, https://doi.org/10.1038/s41467-020-15064-8, 2020.
Mallast, U. and Siebert, C.: Combining continuous spatial and temporal scales for SGD investigations using UAV-based thermal infrared measurements, Hydrol. Earth Syst. Sci., 23, 1375–1392, https://doi.org/10.5194/hess-23-1375-2019, 2019.
Manheim, F. T.: Section of geological sciences: evidence for submarine discharge of water on the Atlantic continental slope of the southern United States, and suggestions for further search, T. New York Acad. Sci., 29, 839–853, 1967.
McDonough, L. K., Andersen, M. S., Behnke, M. I., Rutlidge, H., Oudone, P., Meredith, K., O'Carroll, D. M., Santos, I. R., Marjo, C. E., Spencer, R. G. M., McKenna, A., M., and Baker, A.: A new conceptual framework for the transformation of groundwater dissolved organic matter, Nat. Commun., 13, 2153, https://doi.org/10.1038/s41467-022-29711-9, 2022.
Micallef, A.: Global database of offshore freshened groundwater records, Zenodo [data set], https://doi.org/10.5281/zenodo.4247833, 2020.
Micallef, A., Person, M., Haroon, A., Weymer, B. A., Jegen, M., Schwalenberg, K., Faghih, Z., Duan, S., Cohen, D., Mountjoy, J. J., Woelz, S., Gable, S. W., Averes, T., and Tiwari, A. K.: 3D characterisation and quantification of an offshore freshened groundwater system in the Canterbury Bight, Nat. Commun., 11, 1372, https://doi.org/10.1038/s41467-020-14770-7, 2020.
Micallef, A., Person, M., Berndt, C., Bertoni, C., Cohen, D., Dugan, B., Evans, R., Haroon, A., Hensen, C., Jegen, M., Key, K., Kooi, H., Liebetrau, V., Lofi, J., Mailloux, B. J., Martin-Nagle, R., Michael, H. A., Müller, T., Schmidt, M., Schwalenberg, K., Trembath-Reichert, E., Weymer, B. A., Zhang, Y., and Thomas, A.: Offshore freshened groundwater in continental margins, Rev. Geophys., 58, e2020RG000706, https://doi.org/10.1029/2020RG000706, 2021.
Michael, H. A., Scott, K. C., Koneshloo, M., Yu, X., Khan, M. R., and Li, K.: Geologic influence on groundwater salinity drives large seawater circulation through the continental shelf, Geophys. Res. Lett., 43, 10782–10791, https://doi.org/10.1002/2016GL070863, 2016.
Moore, W. S.: The Effect of Submarine Groundwater Discharge on the Ocean, Annu. Rev. Mar. Sci., 2, 59–88, 2010.
Moore, W. S. and Joye, S. B.: Saltwater Intrusion and Submarine Groundwater Discharge: Acceleration of Biogeochemical Reactions in Changing Coastal Aquifers, Front. Earth Sci., 9, 600710, https://doi.org/10.3389/feart.2021.600710, 2021.
Moore, W. S., Beck, M., Riedel, T., Rutgers van der Loeff, M., Dellwig, O., Shaw, T. J., Schnetger, B., and Brumsack, H.-J.: Radium-based pore water fluxes of silica, alkalinity, manganese, DOC, and uranium: a decade of studies in the German Wadden Sea, Geochim. Cosmochim. Ac., 75, 6535–6555, 2011.
Moosdorf, N., Böttcher, M. E., Adyasari, D., Erkul, E., Gilfedder, B. S., Greskowiak, J., Jenner, A.-K., Kotwicki, L., Massmann, G., Müller-Petke, M., Oehler, T., Post, V., Prien, R., Scholten, J., Siemon, B., Ehlert von Ahn, C. M., Walther, M., Waska, H., Wunderlich, T., and Mallast, U.: A State-Of-The-Art Perspective on the Characterization of Subterranean Estuaries at the Regional Scale, Front. Earth Sci., 9, 601293, https://doi.org/10.3389/feart.2021.601293, 2021.
Mountain, G.: Portable hires multi-channel seismic shot data from the New Jersey slope acquired during the r/v oceanus expedition oc270 (1995), nterdisciplinary Earth Data Alliance (IEDA) [data set], https://doi.org/10.1594/IEDA/307762, 2008.
Müller, H., von Dobeneck, T., Nehmiz, W., and Hamer, K.: Near-surface electromagnetic, rock magnetic, and geochemical fingerprinting of submarine freshwater seepage at Eckernförde Bay (SW Baltic Sea), Geo-Mar. Lett., 31, 123–140, https://doi.org/10.1007/s00367-010-0220-0, 2011.
Null, K. A., Knee, K. L., Crook, E. D., de Sieyes, N. R., Rebolledo-Vieyra, M., Hernández-Terrones, L., and Paytan, A.: Composition and fluxes of submarine groundwater along the Caribbean coast of the Yucatan Peninsula, Cont. Shelf Res., 77, 38–50, https://doi.org/10.1016/j.csr.2014.01.011, 2014.
Oberle, F. K. J., Prouty, N. G., Swarzenski, P. W., and Storlazzi, C. D.: High-resolution observations of submarine groundwater discharge reveal the fine spatial and temporal scales of nutrient exposure on a coral reef: Faga'alu, AS, Coral Reefs, 41, 849–854, https://doi.org/10.1007/s00338-022-02245-8, 2022.
Oehler, T., Bakti, H., Lubis, R. F., Purwoarminta, A., Delinom, R., and Moosdorf, N.: Nutrient dynamics in submarine groundwater discharge through a coral reef (western Lombok, Indonesia), Limnol. Oceanogr., 64, 2646–2661, 2019.
Oehler, T., Ramasamy, M., Mintu, E. G., Babu, S. D. S., Dähnke, K., Ankele, M., Böttcher, M. E., Santos, I. R., and Moosdorf, N.: Tropical Beaches Attenuate Groundwater Nitrogen Pollution Flowing to the Ocean, Environ. Sci. Technol., 55, 8432–8438, 2021.
Paldor, A., Katz, O., Aharonov, E., Weinstein, Y., Roditi-Elasar, M., Lazar, A., and Lazar, B.: Deep submarine groundwater discharge–evidence from Achziv submarine canyon at the exposure of the Judea group confined aquifer, Eastern Mediterranean, J. Geophys. Res.-Oceans, 125, e2019JC015435, https://doi.org/10.1029/2019JC015435, 2020.
Peterson, C. D., Jol, H. M., Percy, D., and Perkins, R.: Use of Ground Penetrating Radar, Hydrogeochemical Testing, and Aquifer Characterization to Establish Shallow Groundwater Supply to the Rehabilitated Ni-les' tun Unit Floodplain: Bandon Marsh, Coquille Estuary, Oregon, USA, J. Geogr. Geol., 12, 25–49, 2020.
Pisternick, T., Lilkendey, J., Audit-Manna, A., Dumur Neelayya, D., Neehaul, Y., and Moosdorf, N.: Submarine groundwater springs are characterized by distinct fish communities, Mar. Ecol., 41, e12610, https://doi.org/10.1111/maec.12610, 2020.
Pohlman, J. W.: The biogeochemistry of anchialine caves: progress and possibilities, Hydrobiologia, 677, 33–51, 2011.
Pondthai, P., Everett, M. E., Micallef, A., Weymer, B. A., Faghih, Z., Haroon, A., and Jegen, M.: 3D Characterization of a Coastal Freshwater Aquifer in SE Malta (Mediterranean Sea) by Time-Domain Electromagnetics, Water, 12, 1566, https://doi.org/10.3390/w12061566, 2020.
Post, V. E. A., Groen, J., Kooi, H., Person, M., Ge, S., and Edmunds, W. M.: Offshore fresh groundwater reserves as a global phenomenon, Nature, 504, 71–78, https://doi.org/10.1038/nature12858, 2013.
Purkamo, L., Milene, C., von Ahn, E., Jilbert, T., Muniruzzaman, M., Bange, H. W., Jenner, A.-K., Böttcher, M. E., and Virtasalo, J. J.: Impact of submarine groundwater discharge on biogeochemistry and microbial communities in pockmarks, Geochim. Cosmochim. Ac., 334, 14–44, 2022.
Reading, M. J., Tait, D. R., Maher, D. T., Jeffrey, L. C., Correa, R. E., Tucker, J. P., Shishaye, H. A., and Santos, I. R.: Submarine groundwater discharge drives nitrous oxide source/sink dynamics in a metropolitan estuary, Limnol. Oceanogr., 66, 1665–1686, https://doi.org/10.1002/lno.11710, 2021.
Rocha, C., Robinson, C. E., Santos, I. R., Waska, H., Michael, H. A., and Bokuniewicz, H. J.: A place for subterranean estuaries in the coastal zone, Estuar. Coast. Shelf S., 250, 107167, https://doi.org/10.1016/j.ecss.2021.107167 2021.
Rodellas, V., Garcia-Orellana, J., Masque, P., Feldman, M., and Weinstein, Y.: Submarine groundwater discharge as a major source of nutrients to the Mediterranean Sea, P. Natl. Acad. Sci. USA, 112, 3926–3930, 2015.
Roxburgh, I. S.: Thermal infrared detection of submarine springs associated with the Plymouth Limestone, Hydrolog. Sci. J., 30, 185–196, 1985.
Röper, T., Greskowiak, J., and Massmann, G.: Detecting small groundwater discharge springs using handheld thermal infrared imagery, Groundwater, 52, 936–942, 2014.
Ruiz-González, C., Rodellas, V., and Garcia-Orellana, J.: The microbial dimension of submarine groundwater discharge: current challenges and future directions, FEMS Microbiol. Rev., 45, fuab010, https://doi.org/10.1093/femsre/fuab010, 2021.
Santos, I. R., Chen, X., Lecher, A. L., Sawyer, A. H., Moosdorf, N., Rodellas, V., Tamborski, J., Cho, H.-M., Dimova, N., Sugimoto, R., Bonaglia, S., Li, H., Hajati, M.-C., and Li, L.: Submarine groundwater discharge impacts on coastal nutrient biogeochemistry, Nat. Rev. Earth. Environ, 2, 307–323, https://doi.org/10.1038/s43017-021-00152-0, 2021.
Sawyer, A. H., David, C. H., and Famiglietti, J. S.: Continental patterns of submarine groundwater discharge reveal coastal vulnerabilities, Science, 353, 7005–707, 2016.
Schubert, M., Scholten, J., Schmidt, A., Comanducci, J. F., Pham, M. K., Mallast, U., and Knoeller, K.: Submarine Groundwater Discharge at a Single Spot Location: Evaluation of Different Detection Approaches, Water, 6, 584–601, https://doi.org/10.3390/w6030584, 2014.
Shlklomanov, I. A.: World fresh water resources, in: Water in Crisis: A Guide to the World's Fresh Water Resources, edited by: Gleick, P. H., Oxford University Press, New York, 13–24, ISBN 0-19507628-1, 1993.
Siemon, B., Ibs-von Seht, M., Steuer, A., Deus, N., and Wiederhold, H.: Airborne Electromagnetic, Magnetic, and Radiometric Surveys at the German North Sea Coast Applied to Groundwater and Soil Investigations, Remote Sens., 12, 1629, https://doi.org/10.3390/rs12101629, 2020.
Sorensen, J. P. R., Aldous, P., Bunting, S. Y., McNally, S., Townsend, B. R., Barnett, M. J., Harding, T., La Ragione, R. M., Stuart, M. E., Tipper, H. J., and Pedley, S.: Seasonality of enteric viruses in groundwater-derived public water sources, Water Res., 207, 117813, https://doi.org/10.1016/j.watres.2021.117813, 2021.
Sugimoto, R., Kitagawa, K., Nishi, S., Honda, H., Yamada, M., Kobayashi, S., Shoji, J., Ohsawa, S., Taniguchi, M., and Tominaga, O.: Phytoplankton primary productivity around submarine groundwater discharge in nearshore coasts, Mar. Ecol.-Prog. Ser., 563, 25–33, 2017.
Szymczycha, B., Vogler, S., and Pempkowiak, J.: Nutrients fluxes via submarine groundwater discharge to the Bay of Puck, Southern Baltic, Sci. Total Environ., 438, 86–93, 2012.
Szymczycha, B., Borecka, M., Białk-Bielińskab, A., Siedlewicz, G., and Pazdro, K.: Submarine groundwater discharge as a source of pharmaceutical and caffeine residues in coastal ecosystem: Bay of Puck, southern Baltic Sea case study, Sci. Total Environ., 713, 136522, https://doi.org/10.1016/j.scitotenv.2020.136522, 2020.
Taniguchi, M., Burnett, W. C., Cable, J. E., and Turner, J. V.: Investigation of submarine groundwater discharge, Hydrol. Process., 16, 2115–2129, https://doi.org/10.1002/hyp.1145, 2002.
Taniguchi, M., Dulai, H., Burnett, K. M, Santos, I. R., Sugimoto, R., Stieglitz, T., Kim, G., Moorsdorf, N., and Burnett, W. C.: Submarine Groundwater Discharge: Updates on Its Measurement Techniques, Geophysical Drivers, Magnitudes, and Effects, Front. Environ. Sci., 7, 141, https://doi.org/10.3389/fenvs.2019.00141, 2019.
Taylor, R., Scanlon, B., Döll, P., Rodell, M., van Beek., R., Wada, Y., Longuevergne, L., Leblanc, M., Famiglietti, J. S., Edmunds, M., Konikow, L., Green, T. R., Chen, J., Taniguchi, M., Bierkens, M. F. P., MacDonald, A., Fan, Y., Maxwell, M., Yechieli, Y., Gurdak, J. J., Allen, D. M., Shamsudduha, M., Hiscock, K., Yeh, P. J.-F., Holman, I., and Treidel, H.: Ground water and climate change, Nat. Clim. Change, 3, 322–329, 2013.
Thomas, A. T., Reiche, S., Riedel, M., and Clauser, C.: The fate of submarine fresh groundwater reservoirs at the New Jersey shelf, USA, Hydrogeol. J., 27, 2673–2694, https://doi.org/10.1007/s10040-019-01997-y, 2019.
Thomas, A. T., von Harten, J., Jusri, T., Reiche, S., and Wellmann, F.: An integrated modeling scheme for characterizing 3D hydrogeological heterogeneity of the New Jersey shelf, Mar. Geophys. Res., 43, 1–19, 2022.
Thomas, B. F. and Famiglietti, J. S.: Identifying Climate-Induced Groundwater Depletion in GRACE Observations, Sci. Rep., 9, 4124, https://doi.org/10.1038/s41598-019-40155-y, 2019.
Van Geldern, R., Hayashi, T., Bottcher, M. E., Mottl, M., Barth, J. A. C., and Stadler, S.: Stable isotope geochemistry of pore waters and marine sediments from the New Jersey shelf: Methane formation and fluid origin, Geosphere, 9, 96–112, https://doi.org/10.1130/GES00859.1, 2013.
Van Meter, K. J., Van Cappellen, P., and Basu, N. B.: Legacy nitrogen may prevent achievement of water quality goals in the Gulf of Mexico, Science, 360, 427–430, 2018.
Varma, S. and Michael, K.: Impact of multi-purpose aquifer utilisation on a variable-density groundwater flow system in the Gippsland Basin, Australia, Hydrogeol. J., 20, 119–134, https://doi.org/10.1007/s10040-011-0800-8, 2012.
Viaroli, S., Lancia, M., and Re, V.: Microplastics contamination of groundwater: Current evidence and future perspectives. A review, Sci. Total Environ., 824, 153851, https://doi.org/10.1016/j.scitotenv.2022.153851, 2022.
Virtasalo, J. J., Schröder, J. F., Luoma, S., Majaniemi, J., Mursu, J., and Scholten, J.: Submarine groundwater discharge site in the First Salpausselkä ice-marginal formation, south Finland, Solid Earth, 10, 405–423, https://doi.org/10.5194/se-10-405-2019, 2019.
von Ahn, C. M. E., Scholten, J., Malik, C., Feldens, P., Liu, B., Dellwig, O., Jenner, A.-K., Papenmeier, S., Schmiedinger, I., Zeller, M. A., and Böttcher, M. E.: A multi-tracer study of fresh submarine and surface water sources for a temperate urbanized coastal bay, Front. Environ. Sci., 9, 642346, https://doi.org/10.3389/fenvs.2021.642346, 2021.
Waska, H. and Kim, G.: Differences in microphytobenthos and macrofaunal abundances associated with groundwater discharge in the intertidal zone, Mar. Ecol.-Prog. Ser., 407, 159–172, 2010.
Waska, H. and Kim, G.: Submarine groundwater discharge (SGD) as a main nutrient source for benthic and water-column primary production in a large intertidal environment of the Yellow Sea, J. Sea Res., 65, 103–113, https://doi.org/10.1016/j.seares.2010.08.001, 2011.
Waska, H., Geskowiak, J., Ahrens, J., Beck, M., Ahmerkamp, S., Böning, P., Brusmack, H. J., Degenhardt, J., Ehlert, C., Engelen, B., Grünebaum, N., Holtappels, M., Pahnke, K., Marchant, H. K., Massmann, G., Meier, D., Schnetger, B., Schwalfenberg, K., Simon, H., Vandieken, V., Tzielinski, O., and Dittmar, T.: Spatial and temporal patterns of pore water chemistry in the inter-tidal zone of a high energy beach, Front. Mar. Sci., 6, 154, https://doi.org/10.3389/fmars.2019.00154, 2019.
Weymer, B. A., Everett, M. E., Smet, T. S., and Houser, C.: Review of electromagnetic induction for mapping barrier island framework geology, Sediment. Geol., 321, 11–24, 2015.
Weymer, B. A., Wernette, P. A., Everett, M. E., Pondthai, P., Jegen, M., and Micallef, A.: Multi-layered high permeability conduits connecting onshore and offshore coastal aquifers, Front. Mar. Sci., 7, 531293, https://doi.org/10.3389/fmars.2020.531293, 2020.
Whiticar, M. J.: Diagenetic relationships of methanogenesis, nutrients, acoustic turbidity, pockmarks and freshwater seepages in Eckernförde Bay, Mar. Geol., 182, 29–53, https://doi.org/10.1016/S0025-3227(01)00227-4, 2002.
Wilson, J. and Rocha, C.: Regional scale assessment of Submarine Groundwater Discharge in Ireland combining medium resolution satellite imagery and geochemical tracing techniques, Remote Sens. Environ., 119, 21–34, https://doi.org/10.1016/j.rse.2011.11.018, 2012.
Worzewski, T., Jegen, M., and Swidinsky, A.: Approximation for the 2D coast effect on marine magnetotelluric data, Geophys. J. Int., 189, 357–368, https://doi.org/10.1111/j.1365-246X.2012.05385.x, 2012.
Yu, X. and Michael, H. A.: Mechanisms, configuration typology, and vulnerability of pumping-induced seawater intrusion in heterogeneous aquifers, Adv. Water Resour., 128, 117–128, https://doi.org/10.1016/j.advwatres.2019.04.013, 2019a.
Yu, X. and Michael, H. A.: Offshore pumping impacts onshore groundwater resources and land subsidence, Geophys. Res. Lett., 46, 2553–2562, https://doi.org/10.1029/2019GL081910, 2019b.
Zamrsky, D., Essink, G. H. O., Sutanudjaja, E. H., van Beek, L. R., and Bierkens, M. F.: Offshore fresh groundwater in coastal unconsolidated sediment systems as a potential fresh water source in the 21st century, Environ. Res. Lett., 17, 014021, https://doi.org/10.1088/1748-9326/ac4073, 2021.
Zhao, S., Xu, B., Yao, Q., Burnett, W. C., Charette, M. A., Su, R., Lian, E., and Yu, Z.: Nutrient-rich submarine groundwater discharge fuels the largest green tide in the world, Sci. Total Environ., 770, 144845, https://doi.org/10.1016/j.scitotenv.2020.144845, 2021.
Zipperle, A. and Reise, K.: Freshwater springs on intertidal sand flats cause a switch in dominance among polychaete worms, J. Sea Res., 54, 143–150, https://doi.org/10.1016/j.seares.2005.01.003, 2005.
- Abstract
- Dedication
- Background
- Distribution and variability of groundwater fluxes
- Environmental impacts and resource prospects
- Conceptual and technological approaches for assessing land–ocean groundwater connectivity
- Future research directions
- Data availability
- Author contributions
- Competing interests
- Disclaimer
- Acknowledgements
- Financial support
- Review statement
- References
- Abstract
- Dedication
- Background
- Distribution and variability of groundwater fluxes
- Environmental impacts and resource prospects
- Conceptual and technological approaches for assessing land–ocean groundwater connectivity
- Future research directions
- Data availability
- Author contributions
- Competing interests
- Disclaimer
- Acknowledgements
- Financial support
- Review statement
- References