the Creative Commons Attribution 4.0 License.
the Creative Commons Attribution 4.0 License.
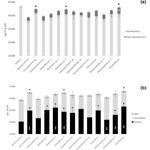
Non-mycorrhizal root-associated fungi increase soil C stocks and stability via diverse mechanisms
Emiko K. Stuart
Laura Castañeda-Gómez
Wolfram Buss
Jeff R. Powell
Yolima Carrillo
While various root-associated fungi could facilitate soil carbon (C) storage and therefore aid climate change mitigation, so far research in this area has largely focused on mycorrhizal fungi, and potential impacts and mechanisms for other fungi are largely unknown. Here, with the aim of identifying novel organisms that could be introduced to crop plants to promote C sequestration, we assessed the soil C storage potential of 12 root-associated, non-mycorrhizal fungal isolates (spanning nine genera and selected from a wide pool based on traits potentially linked to soil C accrual) and investigated fungal, plant and microbial mediators. We grew wheat plants inoculated with individual isolates in chambers allowing continuous 13C labelling. After harvest, we quantified C storage potential by measuring pools of different origin (plant vs. soil) and different stability with long-term soil incubations and size/density fractionation. We assessed plant and microbial community responses as well as fungal physiological and morphological traits in a parallel in vitro study. While inoculation with 3 of the 12 isolates resulted in significant total soil C increases, soil C stability improved under inoculation with most isolates – as a result of increases in resistant C pools and decreases in labile pools and respired C. Further, these increases in soil C stability were positively associated with various fungal traits and plant growth responses, including greater fungal hyphal density and plant biomass, indicating multiple direct and indirect mechanisms for fungal impacts on soil C storage. We found more evidence for metabolic inhibition of microbial decomposition than for physical limitation under the fungal treatments. Our study provides the first direct experimental evidence in plant–soil systems that inoculation with specific non-mycorrhizal fungal strains can improve soil C storage, primarily by stabilising existing C. By identifying specific fungi and traits that hold promise for enhancing soil C storage, our study highlights the potential of non-mycorrhizal fungi in C sequestration and the need to study the mechanisms underpinning it.
- Article
(2825 KB) - Full-text XML
-
Supplement
(356 KB) - BibTeX
- EndNote
Despite soils having the capacity to sequester large amounts of atmospheric CO2 and mitigate catastrophic climate change, the full potential of soil carbon (C) sequestration is yet to be realised (Field and Raupach, 2004; Scharlemann et al., 2014; Schlesinger, 1990). Moreover, rather than being protected, soils are becoming increasingly degraded globally due to intensive agricultural practices – a situation that may worsen as C loss potentially accelerates with future climate scenarios (Hannula and Morriën, 2022; Lal, 2018). While soil C sequestration is becoming more broadly recognised as an important climate mitigation strategy and as an approach to recover the multiple ecosystem services provided by soil C (Kopittke et al., 2022), its successful implementation first requires understanding of processes underpinning the storage of C in soil (Dynarski et al., 2020; Smith and Wan, 2019; Von Unger and Emmer, 2018). Knowledge of soil C storage has improved substantially in recent years, with it now being understood as resulting from the balance of multiple, dynamic processes (that are further complicated by the pedoclimatic context) determining C inputs to soil and their stabilisation (i.e. resistance to decay; Cotrufo and Lavallee, 2022; Derrien et al., 2023; Dignac et al., 2005; Dynarski et al., 2020; Jackson et al., 2017; Schmidt et al., 2011). Soil microbes act as key participants of these processes, as the stability of soil C is regulated primarily via their abilities to mineralise soil organic matter. Thus, soil microbes determine how long C of plant or microbial origin persists in soil and can also influence how much C is available for stabilisation from their necromass and from plant inputs. However, the soil microbial community is complex and largely unknown and hence is referred to as a “black box” (Mishra et al., 2023; Tiedje et al., 1999). Within this black box, fungi, both free-living and plant-associated, are considered particularly important for soil C storage; however, their impacts on soil C storage are both multifaceted and diverse.
The complexity in fungal impacts on soil C storage firstly arises from their abilities to influence both soil C inputs and their stability via multiple direct and indirect mechanisms occurring simultaneously (Hannula and Morriën, 2022; Kallenbach et al., 2016; Liang et al., 2019; Starke et al., 2021). In general, fungi that are present in soil (1) all produce hyphae and with them hyphal C inputs; (2) can alter plant health, growth, and C chemistry and allocation to soil; and (3) can influence the rest of the soil microbial community structure and composition, thus impacting fungal-, plant-, and microbial-derived C, respectively (Clocchiatti et al., 2020; Hannula and Morriën, 2022; Rai and Agarkar, 2016; Stuart et al., 2022). All of these inputs, but particularly fungal and plant C, are potentially available for soil C storage, but they require stabilisation in order to persist in soil long term. The broad and efficient enzymatic capabilities and extensive mycelial structure of fungi, as compared to the rest of the microbial community, allow them to competitively obtain soil C and transform it so that it can be readily sorbed and stabilised onto mineral surfaces (Boer et al., 2005; Hannula and Morriën, 2022). In addition, fungal necromass is considered to have a particularly strong affinity for mineral surfaces and is therefore an important source of stabilisable C (Sokol et al., 2019). The impact of fungi on soil structure and spatial heterogeneity, including promoting aggregate formation by enmeshing soil particles with their hyphae and producing various extracellular biopolymers, further protects C by physically constraining microbial decomposition, leading to greater persistence (Berg and Mcclaugherty, 2014; Dynarski et al., 2020; Kleber et al., 2011; Lehmann et al., 2017; Lützow et al., 2006; Schmidt et al., 2011).
These various impacts of fungi on soil C storage are further complicated by fungal diversity, which occurs at the inter-genus level, at the inter-species level, and even down to the sub-species level (Andrade et al., 2016; Hiscox et al., 2015; Johnson et al., 2012; Juan-Ovejero et al., 2020; Plett et al., 2021). In plant–soil ecosystems, fungi exist either as free-living saprotrophs or as plant-associated fungi, including mycorrhizal, endophytic, and parasitic fungi (Rai and Agarkar, 2016). Saprotrophic fungi are often assumed to promote soil C output, as they decompose soil organic matter due to being outcompeted by mycorrhizal fungi for plant C exudates, but as decomposition can increase the availability of C to be sorbed onto mineral surfaces, thereby fostering soil C stability, their net impacts on soil C storage may need further exploration (Frąc et al., 2018; Hannula and Morriën, 2022; Lehmann and Rillig, 2015). Meanwhile, much of the research on the impacts of plant-associated fungi on soil C has focused on mycorrhizal fungi, particularly arbuscular mycorrhizal fungi and ectomycorrhizal fungi due to their dominance in their respective habitats (Jackson et al., 2017; Smith and Read, 2008). These fungi have impacts, in addition to the general fungal impacts outlined above, on the inputs and stabilisation of C. As they transform and funnel plant C belowground, mycorrhizal fungi can increase and modify the quality of C inputs, for example by synthesising melanin for cell walls, which is considered to be highly stable and has been associated with decreased hyphal decomposability and increased soil C content (Fernandez and Kennedy, 2018; Fernandez and Koide, 2013; Zak et al., 2019; Zhu and Michael Miller, 2003). Due to their nutrient requirements and abilities to mine soil resources, they are thought to be strong competitors against saprotrophs for not only plant C, but also soil nutrients, thereby suppressing microbial respiration and resulting in greater C stability (Gadgil and Gadgil, 1971; Averill and Hawkes, 2016). Some mycorrhizal fungi have limited abilities to directly and partially decay organic matter, and they can also prime saprotrophic microbes to decompose pre-existing soil C, thus having the potential to decrease C stability – though their net impact on soil C storage is not well understood (Frey, 2019). Despite the large diversity amongst fungi in plant–soil ecosystems, influences of non-mycorrhizal fungi, particularly other plant-associated fungi, on soil C storage have been studied in less detail compared to mycorrhizal fungi but do hold promise. For example, endophytic fungi could potentially be important for soil C storage due to their abilities to produce melanin and promote plant growth (Berthelot et al., 2017; He et al., 2019; Mandyam and Jumpponen, 2005; Rai and Agarkar, 2016). However, similar to mycorrhizal fungi, there are conflicting reports regarding their lifestyles, benefits or harms imposed on host plants, or enzymatic and nutrient acquisition ability or even whether they produce extra-radical mycelium, suggesting there may be wide functional variation or plasticity within this fungal group (Addy et al., 2005; Mukasa Mugerwa and Mcgee, 2017; Rai and Agarkar, 2016). To better understand the diversity of fungal impacts on soil C storage, particularly soil C stability, focus is also needed on fungal types other than mycorrhizal fungi.
There is growing interest in searching and screening for organisms that, in addition to supporting plant productivity, may improve soil C storage in agricultural systems (Kaminsky et al., 2019; Islam et al., 2021; Salomon et al., 2022). Thus far, mycorrhizal fungi have received much attention in this area due to their better known impacts on plant health and soil C. However, as discussed above, other fungal types may also offer advantages to soil C storage and plant productivity but have been largely unexplored. With this objective in mind, in the current study we aimed to determine the net impacts of inoculation with diverse non-mycorrhizal fungi on soil C formation (by impacting the origin of soil C) and stability (by impacting C pools, dynamics, and fractions) and to investigate the mechanisms underpinning these impacts, both direct and indirect. We assessed 12 separate fungal species (spanning nine genera in the orders Chaetosphaeriales, Helotiales, and Pleosporales), isolated from roots collected from multiple soil environments across Australia and screened for traits that may support plant growth and soil C storage, such as capabilities to capture and solubilise nutrients from the soil. These fungi were selected with the specific aim of identifying novel organisms that could potentially be introduced to crop plants to improve soil C accrual. In a pot study, we inoculated spring wheat (Triticum aestivum), an important cereal crop, with 1 of the 12 fungi and grew the plants for a full life cycle in 13C-depleted CO2 growth chambers to homogeneously label the plants during the full growth cycle, in order to distinguish soil C from plant-derived soil C. Following harvest, we assessed the total C and its isotopic composition, assessed the C distribution among pools of different stabilities (labile, intermediate, and resistant) via 4-month soil incubations, and evaluated the contribution of soil and plant C to these pools using isotopic analysis. These incubation-based assessments were accompanied by size and density fractionation analyses to quantify mineral-associated organic matter (MAOM), aggregate carbon (AggC), and particulate organic matter (POM). We then measured traits of the fungi and the plant and microbial community to explore the potential direct and indirect mechanisms behind these impacts, respectively. We hypothesised that, if a fungal species increased the total soil C storage, this would be due primarily to increasing plant C inputs by supporting plant growth and also to stabilising existing soil C – so that fungi-driven increases in total soil C would be associated with more stable pools and fractions of C. We expected that these changes to soil C would be associated with fungal traits, alluding to direct mechanisms, as well as to increases in plant growth and shifts in microbial community composition, alluding to indirect mechanisms.
The overall study design consisted of a wheat growth pot experiment in which changes to soil, plant and soil microbial communities in response to fungal inoculation were assessed, together with a separate in vitro fungal growth assay to measure fungal traits that could potentially be linked to observations made in the main experiment (Fig. A1).
2.1 Experiment set-up and maintenance
Twelve fungal isolates were originally isolated from surface-sterilised roots of multiple species of grasses and shrubs from diverse natural environments across south-eastern Australia and were screened for traits that may support plant growth and soil C storage by Loam Bio Pty Ltd (Orange, New South Wales, Australia). Briefly, the screening process included assessing successful colonisation of crop plants (including wheat), testing for responses of soil properties to inoculation and assessing interactions of the fungi with other bacteria and fungi. The fungal isolates, including endophytic fungi and potentially saprotrophic or other fungi, comprised Thozetella, Paraconiothyrium, three Darksidea, Leptodontidium, Clohesyomyces, two Phialocephala, Acrocalymma, Periconia and Ophiosphaerella species.
Pure cultures of these isolates were maintained on strength potato dextrose agar (PDA). Surface-sterilised (2 % NaOCl) and moistened seeds of the Australian wheat cultivar Condo (Triticum aestivum) were incubated at room temperature for 48 h. Clay loam soil was obtained from an agricultural field where the past 10 years of land use history included wheat, barley, canola, and sorghum (4.3 % C, 0.39 % N, pH 5.85; Table B1). The soil was sieved through 2 mm and was not sterilised before use in this experiment.
The experimental set-up consisted of 12 fungal treatments (seven replicates per treatment) and an uninoculated treatment (six replicates) applied to “planted” pots, which were distributed among six CO2-controlled growth chambers (Climatron-1260; Thermoline, Wetherill Park, New South Wales, Australia). Each chamber contained one replicate per treatment for replicates 1 to 6, and replicate 7 was distributed among the chambers. The CO2-controlled growth chambers were modified using the approach by Cheng and Dijkstra (2007) to achieve continuous 13C labelling of plant tissues. Briefly, the chambers were adapted to take an influx of naturally 13C-depleted CO2 (δ13C = −31.7 ‰ ± 1.2) during the photoperiod, combined with a continuous supply of external CO2-free air and set to 450 ppm CO2 concentration. Chambers were adjusted to a 16 h/8 h photoperiod, 22 °C/17 °C, 60 % relative humidity, and 500 µmol m−2 s−1 light intensity. For planted replicates, three 7 mm agar squares from actively growing PDA fungal culture plates were placed near three sterile seeds in 2 L plastic pots (at a depth of 2–3 cm) containing 1800 g of the non-sterile soil. Uninoculated planted pots (“absent/control”) received three agar squares from uninoculated plates. Each agar square contained approximately 1.3 mg C. Smaller pots (containing 500 g of soil) for “unplanted” control pots (four replicates per treatment) were set up 3 d later using two agar squares (as they contained less soil than the planted pots) as controls for impacts of fungi in the absence of plants, adding up to 142 pots in total. After 10 d of growth, seedlings were thinned to one per pot.
Pots were regularly and uniformly watered with tap water. Pots within each chamber were randomly repositioned four times throughout the experiment. The chamber atmosphere was sampled weekly to confirm that the atmospheric CO2 was sufficiently depleted in 13C via a pump system into a Tedlar® SCV (screw cap valve) gas-sampling bag and δ13C analysis in a Picarro G2201i isotopic CO2 CH4 analyser (Picarro Inc., Santa Clara, CA, USA).
2.2 Harvest and plant biomass measurement
Once the plants had senesced and the grain had ripened, at 18 weeks of growth, wheat spikes and shoots were cut off, dried at 70 °C and weighed. The intact root-containing soil was preserved in the pots by freezing at −20 °C immediately after shoots were cut to stop all decomposer activity to retain the C status generated by the treatment until ready for subsampling and processing. After 2 d of thawing at 4 °C, soil was removed from the pots and a subsample for fractionation analysis was collected from near the root crown and oven-dried at 40 °C. The main root system was gently shaken of soil and one-third of the roots were cut, washed, patted dry and frozen at −20 °C prior to root morphology measurement. The rest of the soil was homogenised before subsample collection. A subsample for phospholipid fatty acid (PLFA) analysis was frozen at −20 °C. A subsample for soil moisture content was weighed and dried at 105 °C. A subsample for soil incubations was oven-dried at 40 °C and sieved at 2 mm, and of this, a further subsample for isotope analysis was dried at 105 °C. To obtain the total root mass, first the root / soil ratio outside the main root system was estimated by collecting the root mass of the remaining soil (after all subsampling) via wet sieving (500 µm) and oven-drying at 40 °C. The root mass of the soil subsamples was calculated using this ratio and the amount of soil in all the subsamples.
2.3 Root morphology
To evaluate root morphology, a potential indirect mechanism for fungal impacts on soil C storage, washed, dried, and frozen root subsamples were arranged with minimal overlap for digital scanning (Epson Expression 11000XL scanner, Epson, Macquarie Park, Australia). Images were analysed with WinRhizo Pro software 2015 (Regent Instruments Inc., Quebec City, Canada) to obtain root average diameter (mm), specific length as the ratio of length to dry mass (cm mg−1), tissue density as mass per unit volume (g cm−3), specific surface area as the ratio of area to dry mass (cm2 g−1), and branching as the number of forks per unit of mass (number per milligram). Following root morphology assessment, the root subsample was oven-dried at 40 °C for determination of the total root mass.
2.4 Plant and soil isotope and chemical analysis
To determine the contribution of soil- and plant-derived C to the total C in soils under wheat, isotopic compositions and the content of ground shoots and soil were assessed using an elemental analyser interfaced to a continuous-flow isotope ratio mass spectrometer (UC Davis Stable Isotope Facility, Davis, California, USA). The proportion of the original soil C present in the soil of each pot after plant growth was calculated via isotopic partitioning following Eq. (1).
δ13CSoil is the 13C isotopic composition of soil measured in each planted pot, δ13CUP-Soil is the mean 13C isotopic composition of soil in unplanted controls, and δ13CP is the 13C isotopic composition of the plant shoots in each planted pot. The plant C proportion (including C from other biological sources) was defined as 1 minus the soil C proportion. These proportions were then applied to the measured C concentrations in each pot to calculate plant- and soil-derived C amounts.
2.5 Soil incubations
To evaluate fungal impacts of fungal isolates on the C distribution across pools of different stability (labile, intermediate, and resistant), we assessed microbial CO2 production during 135 d laboratory incubations of soil harvested at the time of wheat harvest. Headspace samples from incubation jars containing 30 g soil, incubated under standard temperature and moisture conditions (25 °C and 42 % gravimetric moisture, respectively), were collected on 16 occasions over the course of 135 d. Following incubation, we fitted decay model exponential decay equations to estimate decay kinetic parameters. Kinetic parameters derived from mid- to long-term soil incubation are sensitive functional measures of changes in the distribution and stability of C pools resulting from previous exposure to experimental treatments (Carney et al., 2007; Carrillo et al., 2011; Jian et al., 2020; Langley et al., 2009; Taneva and Gonzalez-Meler, 2008). Measured CO2 production rates over time were fitted to a two-pool exponential decay model to estimate the size of the labile and intermediate C pools and their mean residence time (MRT; Cheng and Dijkstra, 2007; Wedin and Pastor, 1993). The size of the resistant pool was calculated as the difference between the total measured organic C and the sum of the estimated labile and intermediate pools. This same procedure was also applied to the portion of CO2 that was released from the originally present soil C (soil-derived C, i.e. not plant-derived C), which was determined via isotopic partitioning of plant- and soil-derived CO2. Based on these, we calculated the total CO2 released from plant- and soil-derived C during the full length of the incubation. See the Supplement for full details on incubations, isotopic partitioning, and decay curve fitting.
2.6 Soil fractionation analysis
Soil fractionation analysis was performed as an alternative method to soil incubations for understanding fungal impacts on C stability. Hereafter we refer to the pools measured via fractionation analysis as “fractions”, as opposed to “pools” measured via soil incubations. The analysis was performed according to a method developed by Poeplau et al. (2017, 2018) and adapted by Buss et al. (2021) involving high-throughput physical fractionation into conceptually designed soil C fractions – MAOM, AggC, and POM. See the Supplement for further details.
2.7 Soil PLFA analysis
Total microbial community size and composition are also potential indirect drivers of fungal impacts on soil C storage. Microbial PLFAs in soils were extracted from 2 g of freeze-dried soil harvested from the wheat growth experiment, following the high-throughput method developed and described by Buyer and Sasser (2012; see the Supplement).
2.8 In vitro fungal assessment
To assess the morphological and chemical properties of the fungal isolates (used in the wheat growth experiment) as potential drivers of fungal impacts on soil C storage, a separate in vitro plate assay was performed using PDA plates incubated in the dark at 23–25 °C (see the Supplement). The radial growth rate was calculated by measuring colony areas every 2 to 3 d using ImageJ (National Institutes of Health, Bethesda, Maryland, USA; Schneider et al., 2012). The growth rate was calculated by subtracting the colony area from an earlier sampling point from that of the following sampling point. Hyphal density was calculated as the final fungal biomass per final colony area. C and N contents were measured by Dumas combustion using an El Vario cube analyser (Elementar, Langenselbold, Germany).
2.9 Data and statistical analysis
ANOVA of soil C properties and experimental variables was performed in R (v. 4.1.2; R Core Team, 2021), followed by Dunnett's post hoc test to determine which treatment groups were significantly different to the uninoculated control group or Tukey's post hoc test to determine significant differences between the inoculated groups. Principal component analysis (PCA) of soil C property data was performed to identify soil C properties associated with fungi-driven increases in soil C. Redundancy analyses (RDAs) of soil C property data as response variables and either plant and microbial community data or using in vitro fungal assessment data as explanatory variables were performed to identify explanatory variables for fungi-driven increases in soil C and its stability. Both analyses were performed using the vegan package in R (Oksanen et al., 2022). Missing values (17 values across 46 total variables) in the PCA and RDA datasets were replaced with the treatment mean.
Curve fitting of CO2 rate dynamics was done using the non-linear modelling platform in JMP 16.1.0 and the bi-exponential four-parameter decay model using all replicates of a treatment. We used non-linear least square curve fitting to test whether the models were significantly different between a fungal treatment and uninoculated control, using the nls function in R.
3.1 Several non-mycorrhizal fungal species increased soil C under wheat plants
We inoculated wheat plants (Triticum aestivum) with 1 of 12 fungi (non-mycorrhizal) isolated from plant roots. After 4 months of plant growth, there was a positive but varied effect of fungal inoculation on soil C content compared to the uninoculated control group (p<0.05; Fig. 1, Table B2). This effect was not observed in soils that received the same fungi but were unplanted (p = 0.22; Fig. 1). We found significant isolate-specific increases in the soil C content of the planted treatments under inoculation with Thozetella sp., Darksidea sp. 3, and Acrocalymma sp., relative to the uninoculated control, of 9.4 % (percentage of change), 7.5 %, and 7.8 %, respectively. Nitrogen levels were generally higher in the soils of the inoculated and planted treatments compared to the uninoculated control and were generally higher in the treatments where C was also higher (Table B2).
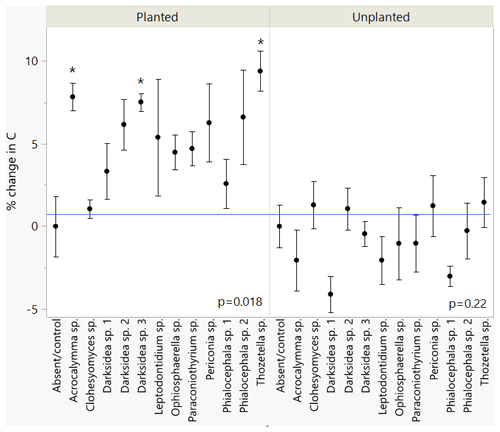
Figure 1Changes in total soil C under inoculation with different fungal isolates compared to an uninoculated control. Values indicate percentage of change relative to mean of uninoculated control (blue line). Error bars indicate standard error, n=7 for inoculated treatments, n=6 for control. ANOVA results for planted and unplanted are presented. Asterisks indicate significant differences with control (Dunnett test, p<0.05). C concentrations are presented in Table B2.
3.2 Fungi-dependent increases in soil C are associated with changes in soil C pools, origin and stability
To understand the underlying mechanisms of the fungal isolate-dependent increases in soil C content and potential shifts in the sources and stability of the resulting soil C, we performed C isotope analysis, soil incubations, and soil C fractionation analysis. Isotopic partitioning of C into plant- and soil-derived C revealed how changes in these pools contributed to changes in the total soil C (Fig. 2a, Table B2). Planting reduced the total soil C compared to the initial C prior to planting (t = 4.13, p<0.001), as expected due to C inputs stimulating decomposition (rhizosphere priming). This reduction was due to decreases in soil-derived C, which were generally not counteracted by newly added plant-derived soil C – which on average represented 3.8 % (± 0.2) of the total soil C (Fig. A2a). Soil C increases under fungal inoculation had different origins depending on the fungal treatment. One of the fungal treatments whereby the total soil C significantly increased (Thozetella sp.) tended to contain higher levels of plant-derived C (p = 0.06). However, overall, the higher total soil C content relative to controls correlated more closely with the higher soil-derived C (Pearson's R = 0.93, p<0.01) than with the plant-derived C (Pearson's R = 0.02, p = 0.83). All three fungal treatments resulting in significant increases in the total soil C showed increases in the soil-derived C, but these were not statistically significant.
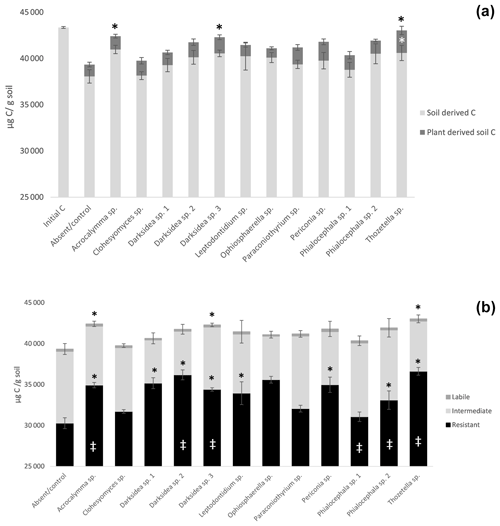
Figure 2Distribution of total soil C in plant- and soil-derived pools (a) and among labile, intermediate, and resistant pools (b) in soil under inoculation with different fungal isolates or under no inoculation (Absent/control). (a) Plant- and soil-derived C from C isotope partitioning (see Materials and methods). Black asterisks indicate significant differences in total C with control and white asterisks differences in plant-derived soil C with control (Dunnett test, p<0.1); (b) pools estimated from decay models derived from soil incubation (see Materials and methods). Crosses indicate significant differences in the dynamics of total C decomposition (decay curves models, Table B3) compared to the uninoculated control. Asterisks indicate significant differences in total C or resistant C against control (Dunnett test, p<0.05). Error bars indicate standard error of total C, n=7 for inoculated treatments, n=6 for uninoculated control. Note y axis scale.
Incubation of soils after plant harvest demonstrated impacts of several fungal species on the dynamics of C decomposition and the distribution of C among soil pools of different stability. The dynamics of total C decomposition (decay curve models derived from incubations) were significantly different to the control under half of the isolates (Table B3, Fig. A3). These included the three isolates that produced higher total C pools: Thozetella sp., Darksidea sp. 3, and Acrocalymma sp. Soil-derived C decomposition curves (from isotopic partitioning of respiration) were also significantly different to the controls under the same fungal treatments as well as Leptodontidium sp. Estimated pools from these decay curves showed significantly higher total resistant C (up to 86 % of C), compared to controls (76 % of C), under 8 of the 12 isolates, including the three treatments where total C increased the most (Figs. 2b and A2b, Table B3). In terms of other pools, MRT of the total labile C was significantly lower under inoculation with Darksidea sp. 1 compared to the control, whereas MRT of the soil-derived labile C was significantly higher under inoculation with Periconia sp. (Table B3). In terms of intermediate pool MRTs, controls and fungal treatments were not significantly different.
Soil incubations and partitioning of respiration revealed fungal effects on the degree of stability of total C, soil-derived C, and plant-derived C over time, which we assessed as the proportion of what was present at harvest that was respired over the full incubation. Significantly lower proportions of total and soil-derived C were respired under all fungal treatments compared to the controls (p<0.001; Fig. A4), indicating increased stability. In contrast, plant-derived respired C was significantly lower (more stable) than the controls with only Thozetella sp. (p<0.05).
From fractionation analysis, % C and % N of the AggC fraction, i.e. the fraction of intermediate stability whereby C is protected in aggregates, were found to have significant fungal effects, with Thozetella sp. and Periconia sp. exhibiting significantly higher levels of both C and N and Ophiosphaerella sp. and Phialocephala sp. 1 exhibiting significantly higher levels of N compared to controls (Table B4). Significant fungal effects were not observed in the MAOM and POM fractions.
We performed PCA to identify soil C properties associated with fungi-driven increases in soil C (Fig. 3). Most of the variance was explained by PC1 and PC2 (58 %). Greater total soil C (C) was closely associated with soil-derived C (SC), but not plant-derived C (PC), at the time of harvest and soil N. Soil C was also related to the resistant C pools (total, TRC, and soil-derived, SRC). The treatments with the lowest total soil C (mainly the control, followed by Clohesyomyces sp. and Phialocephala sp. 1; Fig. 1) were associated with higher proportions of the total and soil-derived C respired during incubation, indicating that the C remaining at harvest was inherently less stable. The % C of the AggC and MAOM fractions, generally considered to be more stable fractions of C, were not clearly associated with soil C or the resistant C pools, nor with any fungal treatments.
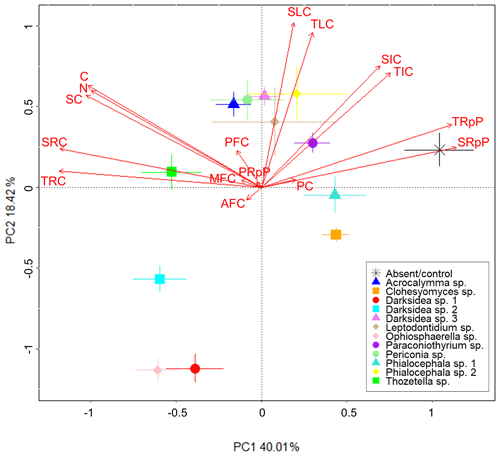
Figure 3Fungi-dependent increases in soil C largely relate to measures for soil C stability. Principal component analysis showing soil C properties (red text) associated with various fungal isolates (symbols). Soil C properties were measured via isotope analysis, soil incubations, and fractionation analysis of soil from wheat experiment. Soil C property abbreviations: AFC, aggregate C fraction % C; C, % C; MFC, MAOM fraction % C; N, % N; PC, plant-derived C (µg g−1 soil); PFC, POM fraction – % C; PRpP, plant-derived C respired proportion; SC, soil-derived C (µg g−1 soil); SIC, soil-derived intermediate C (µg C g−1 soil); SLC, soil-derived labile C (µg C g−1 soil); SRC, soil-derived resistant C (µg C g−1 soil); SRpP, soil-derived C respired proportion; TIC, total intermediate C (µg g−1 soil); TLC, total labile C (µg g−1 soil); TRC, total resistant C (µg g−1 soil); TRpP, total C respired proportion.
3.3 Fungi-dependent increases in soil C and its stability are positively associated with plant growth and microbial community composition
We assessed plant and microbial community variables, including plant biomass, shoot content, root morphology, and total microbial community size and composition derived from PLFA analysis. Overall, while variation among fungal isolates was observed, no significant differences were observed between the inoculated and uninoculated plants for any of the plant or microbial community variables measured, although the average spike mass of Thozetella-inoculated plants was significantly higher than that of uninoculated plants (Tables B5–6).
To identify plant and microbial community variables potentially involved in the fungal isolate-dependent changes in soil C properties, we performed RDA using plant and microbial community data and the soil C property data used in the PCA (Fig. 4). The variance explained by RDA1 and RDA2 was 14.28 % and 4.72 %, respectively. The cluster of soil C properties that were found to be closely associated with Thozetella sp. in the PCA (e.g. soil-derived C, resistant C pools; Fig. 3) also trended positively with plant biomass and growth (spike and shoot mass, shoot ratio, and root fork number) and with the PLFA-assessed fungal / bacterial ratio. Acrocalymma sp. and Darksidea sp. 3 were more associated with root growth traits and were also associated with plant-derived C. The low soil C treatments (uninoculated control, Clohesyomyces sp., and Phialocephala sp. 1) and their associated soil C properties (i.e. respired C) were related to shoot C and N.
3.4 Fungi-dependent increases in soil C and its stability are associated with denser fungal hyphae and a higher fungal ratio
Fungal isolates showed strong differentiation in all of the in vitro assessed variables relating to growth and content (statistically significant effects on all variables, p<0.001; Table B7). Biomass, colony area, and growth rate tended to be positively associated variables and were higher in several treatments including Acrocalymma sp., Darksidea sp. 3, and Phialocephala sp. 1. In contrast, Thozetella sp. and Clohesyomyces sp. tended to have lower values for these variables, but Thozetella sp. had a significantly higher hyphal density than all the other treatments.
We performed a separate RDA to identify fungal variables potentially involved in increases in fungi-dependent soil %C and its stability, using in vitro fungal assessment data and the soil C property data (Fig. 5). Compared to the RDA using plant and microbial community data (Fig. 4), greater proportions of variance were explained in this RDA by RDA1 and RDA2 (21.1 % and 9 %, respectively). Fungal colony area and hyphal density were close to opposite in their direction, with the high soil C treatment Thozetella sp. closely associated with hyphal density and the low soil C treatment Clohesyomyces sp. more associated with colony area. Similarly, the fungal colony maximum growth time and rate (denoting slower and faster fungal growth, respectively) were in opposing directions. Along this axis, the high soil C treatment Darksidea sp. 3 was closely associated with the maximum fungal growth rate. Respired C proportions were closely associated with fungal N content and were opposite to the resistant C fractions, which were associated with the fungal ratio and hyphal density.
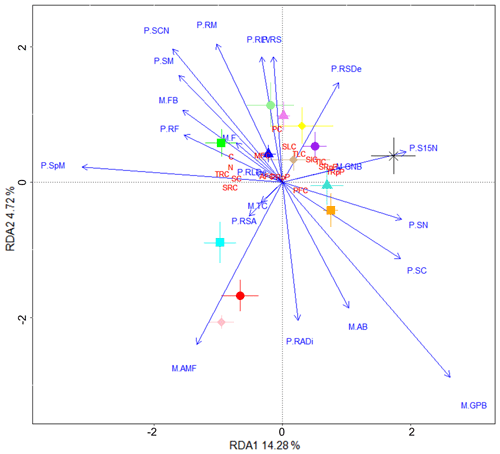
Figure 4Fungal treatments resulting in increased soil C and its stability are associated with plant growth. Redundancy analysis showing microbial community and plant variables (blue text) driving changes in soil C properties (red text) associated with various fungal isolates (symbols). Soil C properties were measured via isotope analysis, soil incubations, and fractionation analysis of soil from wheat experiment. Microbial community and plant variables were measured using samples harvested from the wheat experiment. Microbial community (M.) and plant (P.) variable abbreviations: M.AB, actinobacteria (% of total community); M.AMF, arbuscular mycorrhizal fungi (% of total community); M.F, fungi (% of total community); M.FB, fungal to bacterial biomass ratio; M.GNB, gram negative bacteria (% of total community); M.GPB, gram positive bacteria (% of total community); M.TC, total community size (µg PLFA g−1 soil); P.RADi, root average diameter (mm); P.RF, root fork number (g−1); P.RLDe, root length density (cm g−1); P.RLV, root length per volume (cm m−3); P.RM, root mass (g); P.RS, root/shoot ratio; P.RSA, root specific surface area (cm2 g−1); P.RSDe, root specific density (g cm−3); P.S15N, shoot δ15N (‰); P.SC, shoot % C; P.SCN, shoot ratio; P.SM, shoot mass (g); P.SN, shoot % N; P.SpM, total spike mass (g). Soil C properties: AFC, aggregate C fraction – % C; C, % C; MFC, MAOM fraction – % C; N, % N; PC, plant-derived C (µg g−1 soil); PFC, POM fraction – % C; PRpP, plant-derived C respired proportion; SC, soil-derived C (µg g−1 soil); SIC, soil-derived intermediate C (µg C g−1 soil); SLC, soil-derived labile C (µg C g−1 soil); SRC, soil-derived resistant C (µg C g−1 soil); SRpP, soil-derived C respired proportion; TIC, total intermediate C (µg g−1 soil); TLC, total labile C (µg g−1 soil); TRC, total resistant C (µg g−1 soil); TRpP, total C respired proportion.
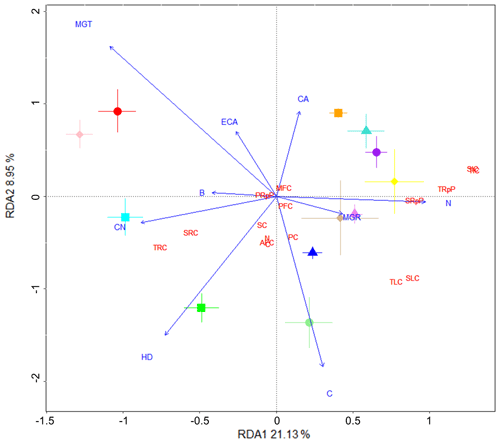
Figure 5Fungal isolates involved in increased soil C and its stability have denser hyphae. Redundancy analysis (RDA) showing the fungal variables (blue text) driving changes in soil C properties (red text) associated with the various fungal isolates (symbols). Soil C properties were measured via isotope analysis, soil incubations, and fractionation analysis of soil from wheat experiment. Fungal variables were measured in an in vitro plate assay and values were averaged for the RDA. Fungal (F.) variable abbreviations: F.B, biomass (g); F.C, % C; F.CA, final colony area (cm2); F.CN, ratio; F.ECA, estimated final colony area (cm2); F.HD, hyphal density (mg cm−2); F.MGR, maximum growth rate (cm−2 day); F.MGT, time to maximum growth (days); F.N, % N. Soil C properties: AFC, aggregate C fraction – % C; C, % C; MFC, MAOM fraction – % C; N, % N; PC, plant-derived C (µg g−1 soil); PFC, POM fraction – % C; PRpP, plant-derived C respired proportion; SC, soil-derived C (µg g−1 soil); SIC, soil-derived intermediate C (µg C g−1 soil); SLC, soil-derived labile C (µg C g−1 soil); SRC, soil-derived resistant C (µg C g−1 soil); SRpP, soil-derived C respired proportion; TIC, total intermediate C (µg g−1 soil); TLC, total labile C (µg g−1 soil); TRC, total resistant C (µg g−1 soil); TRpP, total C respired proportion.
Discussions on soil C sequestration as a climate change strategy have largely focused on one side of the soil C storage system – increasing C inputs into soil (promoting soil C formation). However, increased soil C storage can also be achieved through reductions in soil C outputs. In this study, we focused our attention on fungi that have the potential to improve soil C storage but that are often overlooked in this area of research, using a high-resolution, multifaceted approach combining isotopic labelling, soil incubations, and soil fractionation analysis as well as an in vitro study in parallel. Our study supports the notion that inoculation with non-mycorrhizal root-associated fungi can improve soil C storage via multiple direct and indirect mechanisms determining C inputs and stabilisation. Mechanisms that increased the stability of the existing C were more common across the diverse fungal treatments than those increasing the input of new C.
Despite our finding that bulk soil C increased significantly under only three fungal treatments, in support of our hypothesis, our incubations revealed significant increases in directly and functionally assessed soil C stability (i.e. increases in resistant pools and decreases in respired C during incubation) under most of the fungal treatments, with the stabilised C being the original soil C and not new inputs of C. Thus, as well as contributing to evidence that fungal inoculation can lead to increased soil C content (e.g. Kallenbach et al., 2016), our study provides direct evidence from plant–fungi soil systems for non-mycorrhizal fungi-driven improvements to soil C storage, primarily via enhanced stability of soil C. This is emphasised by our findings that the treatments whereby the soil C content was lowest (control, Clohesyomyces sp., and Phialocephala sp. 1) were associated with higher proportions of the total and soil-derived C respired during incubation, indicating that the C remaining at harvest under these treatments was inherently more prone to decomposition (i.e. less stable). Increased stability of soil C primarily results from inhibition of microbial decomposition (Cotrufo and Lavallee, 2022), which can occur for a variety of reasons, including reduced saprotrophic activity due to microbes being out-competed for nutrients (Boer et al., 2005); increased input of fungal, more readily stabilised C (Sokol et al., 2019); and increased soil aggregation (Lehmann et al., 2020). We investigated multiple potential mediators for the observed increases in soil C stability in our study and found some leads. We found that the increased fungal ratio and hyphal density may be important for the stability of soil C (while fungal N corresponded to decreased stability). Fungi with denser hyphae can promote soil aggregation, as soil particles become more entangled and stabilised in dense hyphae (Dignac et al., 2017). Our study substantiates previous assertions that fungal trait expression is relevant to soil C stability: fungi that exhibited an exploitative growth strategy (denser hyphae) were found to be more closely associated with soil C stability, while fungi that exhibited a more exploratory strategy (faster growth) were positively associated with respired C and less stable C pools (Camenzind et al., 2020; Fernandez et al., 2019; Fernandez and Koide, 2013; Jackson et al., 2017; Lehmann et al., 2020; Schmidt et al., 2011; Zanne et al., 2020). These findings support the notion that an exploitative growth strategy may be more conducive to competition with saprotrophs for nutrients, leading to reduced decomposition (Bödeker et al., 2016).
Our PLFA-assessed finding regarding the fungal / bacterial ratio points towards a second likely mechanism for the increases in soil C stability – a greater proportion of fungal C, which becomes stabilisable necromass. Fungal necromass is a significant source of soil C inputs and can in some cases make up the majority of SOM (soil organic matter) (Wang et al., 2021). Substrates with high ratios, such as fungal biomass or necromass, are generally associated with reduced decomposition rates, although the ratio is not the sole determinant of substrate decomposition and ratios can in fact be altered by, rather than alter the activity of, soil microbial communities (Marañón-Jiménez et al., 2021; Smith and Wan, 2019; Schnecker et al., 2019). Compared with other substrates, however, necromass is a particularly stabilisable form of C as it can bind to the surfaces of MAOM or be stabilised on aggregates, where it is physically protected from decomposition (Sokol et al., 2019). For these reasons, we expected to see positive associations between soil C stability and aggregates and MAOM soil fractions, which are regarded as signifying increased and longer-term stability (Dynarski et al., 2020; Hemingway et al., 2019; Islam et al., 2022; Poeplau et al., 2017, 2018). However, in our study, these fractions were not strongly associated with soil C content or its distribution in pools, nor were they as influential in differences between fungal treatments. While this lends support to the notion that microbial decomposition of soil C was metabolically inhibited (as discussed above) rather than physically limited, our findings may be explained to some extent by the methodology. A potential explanation for our findings is that, although fungal necromass may have been abundant, the experimental conditions may have been unsupportive of MAOM formation (e.g. the high C content of the unplanted soil may have meant that the MAOM content was already at the saturation level and that the new MAOM was not able to form). Other potential explanations are that the MAOM fraction could possibly take longer than the experimental time frame to change substantially or that the MAOM estimation method may carry greater error, thus making detection of responses more difficult. Nonetheless, our study detected increases in total C and C stability that were not associated with the MAOM, suggesting that soil fractionation analyses do not entirely accurately reflect the natural soil C distribution and stability which can be detected functionally via soil incubations. Further studies utilising the combined approach of soil incubations and soil fractionation analysis, such as studies using soil with a lower C content or studies over a longer time period, may shed light on how findings from the two methods can be compared. However, our findings call for caution in directly equating operationally defined MAOM pools and their size with C stability and suggest that functionally assessing C dynamics may be more effective in some cases.
In terms of improvements to soil C content, of the three fungal treatments whereby soil C increases were significant, only one was accompanied by increases in plant-derived C (Thozetella sp.). While we expected that there would be some variation in the fungal impacts on soil C storage due to the diversity amongst the fungi included in this study, this finding is in contrast to our expectation that increases in plant-derived C would be the main mechanism involved in C increase. As plant growth promotion and changes in nutrient uptake are well-known characteristics of some fungi (Hossain et al., 2017), the increase in plant-derived C with Thozetella sp. may have been related to the increases in the quantity or quality of plant inputs related to the shifts in plant variables of Thozetella sp. (spike mass, shoot biomass, and shoot ratio). Our results from the isotopic partitioning of respiration from soil incubations further indicate that the plant-derived C present in soil which contributed to the total soil C increase under inoculation with Thozetella sp. was more stable compared to the control or other treatments. Fungal-derived C could also have contributed to the size and stability of plant-derived C if the fungi took up plant-derived C. Thus, in addition to increasing plant inputs, Thozetella sp. appears to have been more active in stabilising those inputs via the mechanisms discussed above.
Our study addresses key knowledge gaps in the ways fungi affect soil C storage. We have explicitly demonstrated that inoculation with non-mycorrhizal fungi can improve soil C content and, moreover, soil C stability – supporting the general agreement in this field that microbial transformations of soil C and microbially driven changes to soil structure are as important as, if not more important than, the characteristics of the inputs themselves for soil C storage (Dynarski et al., 2020; Hannula and Morriën, 2022). When it comes to evaluating the potential of fungi to support soil C storage, our findings indicate that it is important to consider not only increases in soil C, but also their impact on the stability of C. Among the diverse fungi studied, these improvements in soil C stability largely resulted from reductions in C outputs by increasing stable C pools and the resistance of existing soil C to decomposition. We emphasise that these findings from our study are net outcomes of fungal inoculation, which can impact soil C either via direct mechanisms or indirect mechanisms, including interactions of the fungi with the surrounding soil ecosystem. While potential mechanisms behind the improvements in soil C stability depended on fungal identity, our study points towards metabolic inhibition (rather than physical limitation) of microbial decomposition for which growth characteristics such as the density of fungal hyphae and the fungal ratio may be important indicators – thus, fungal trait expression may be a proxy for fungal influences on soil C storage. However, more work is needed to test whether or not physical limitation of microbial decomposition leads to enhanced soil C stability by these fungi. More rarely, the improvements to soil C storage involved the effects of fungal inoculation on host plant growth and C inputs (directly as plant or plant-derived fungal C). While the total soil C content increased significantly only under a minority of fungal treatments, the significant and common fungi-driven increases in stability we observed could potentially lead to even greater increases in soil C content and its persistence over time – however, experiments with longer time frames are needed to test this idea. This study and continued work will advance knowledge of these mechanisms and support the search and potential implementation of root-associated fungi to improve soil C storage, which will aid soil C sequestration strategies.
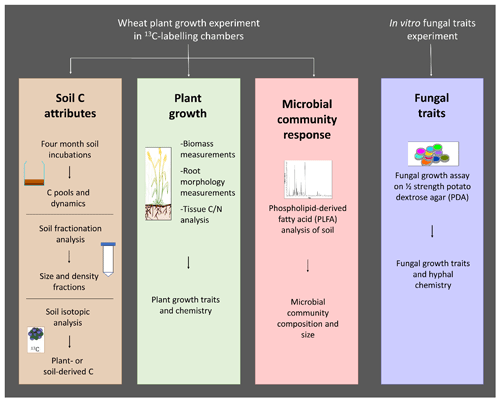
Figure A1Overview of the study design, measured traits, and methodology used. C: carbon; N: nitrogen.
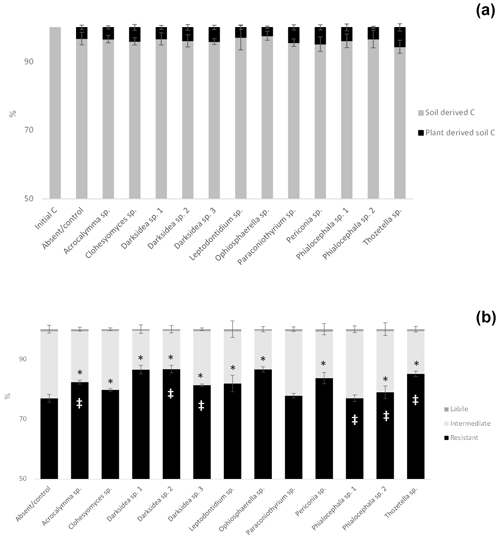
Figure A2Percentage distribution of total soil C in soil- and plant-derived pools (a) and among labile, intermediate and resistant pools in soil under inoculation with different fungal isolates or under no inoculation (absent/control) (b). (a) Percentages of soil- and plant-derived C from C isotope partitioning (see Materials and methods). (b) Percentage distributions of pools estimated from decay models derived from soil incubations (see Materials and methods). Crosses indicate significant differences in the dynamics of total C decomposition (decay curve models, Table B3) compared to the uninoculated control. Asterisks indicate significant differences in total C or resistant C against control (Dunnett test, p<0.05). Error bars indicate the standard error of total C: n=7 for inoculated treatments, and n=6 for uninoculated control. Note the y-axis scale.
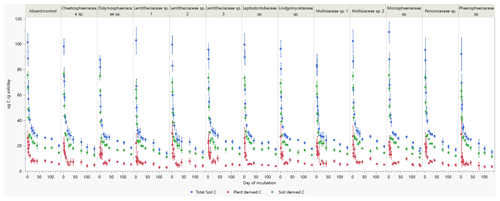
Figure A3Total soil respiration and its soil- and plant-derived components during laboratory soil incubations of soils collected after plant growth with inoculation of 12 fungal species and a control (absent/control). Data points are means (n=7 for inoculated pots; n=6 for controls). Soil and plant components are calculated from isotopic partitioning based on planted and unplanted soil δ13C. Error bars are the standard errors. Family (genus): Chaetosphaeriaceae sp. (Thozetella sp.); Didymosphaeriaceae sp. (Paraconiothyrium sp.); Lentitheciaceae sp. 1 (Darksidea sp. 1); Lentitheciaceae sp. 2 (Darksidea sp. 2); Lentitheciaceae sp. 3 (Darksidea sp. 3); Leptodontidiaceae sp. (Leptodontidium sp.); Lindgomycetaceae sp. (Clohesyomyces sp.); Mollisiaceae sp. 1 (Phialocephala sp. 1); Mollisiaceae sp. 2 (Phialocephala sp. 2); Morosphaeriaceae sp. (Acrocalymma sp.); Periconiaceae sp. (Periconia sp.); Phaeosphaeriaceae sp. (Ophiosphaerella sp.)
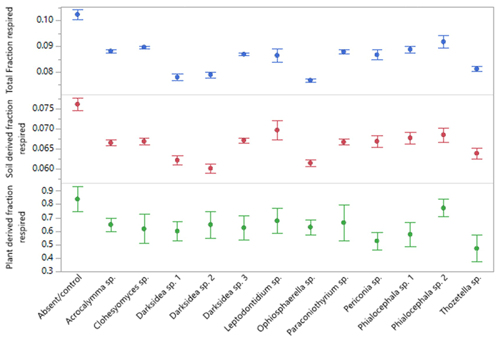
Figure A4Fraction of soil carbon (C) respired over the course of 135 d incubation of soils under wheat and 12 types of fungal inoculum. Total C is all C respired, and soil- and plant-derived C was obtained from isotopic partitioning of respiration over time (see Materials and methods). Values are the means of n=7 for treatments and n=6 for control. Error bars are the standard error.
Table B1Chemical and physical analysis of pre-planted soil used in the wheat experiment. Analysis was performed by the Environmental Analysis Laboratory (East Lismore, Australia).
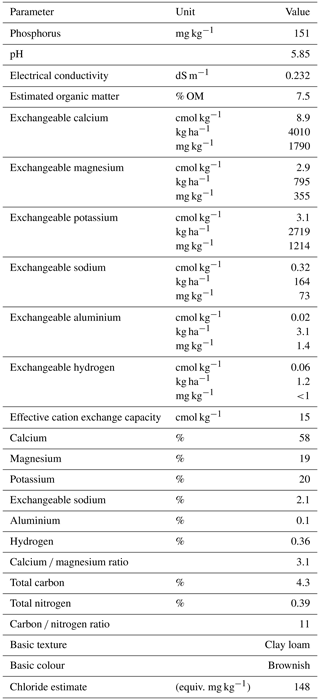
Table B2Properties of soil in which inoculated wheat plants were grown for 4 months. P values from ANOVA are displayed in the bottom row. Asterisks or crosses in other rows (if present) indicate significant differences to uninoculated controls as determined via Dunnett's post hoc test († p<0.1, * p<0.05, p<0.01, p<0.001). C: carbon; N: nitrogen.
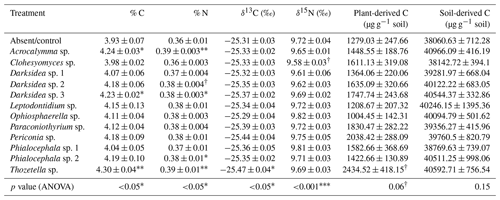
Table B3Model fit, model comparisons, pool sizes (resistant, intermediate and labile) and pool mean residence times (labile and intermediate) estimated from four parameter exponential decay models fitted to CO2 released over 135 d incubations of soil under wheat and fungal inocula. Total C is C in all the CO2 released, and soil-derived C is C of non-plant origin calculated through isotopic partitioning of CO2 based on plant and CO2 δ13C. Asterisks indicate significant difference with uninoculated controls (‡ p<0.1, * p<0.05, p<0.01, p<0.001). † indicates variables for which no statistical test was possible as they were estimated from average curves per treatment. For details of the parameter estimation and isotopic partitioning, see the Materials and methods section. C: carbon; MRT: mean residence time.
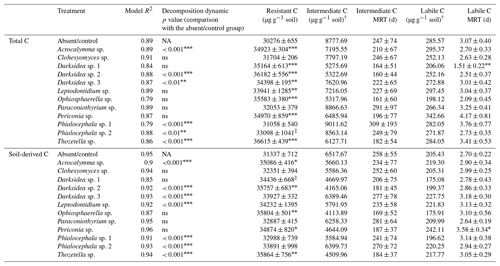
Table B4Properties of C fractions of soil in which inoculated wheat plants were grown for 4 months. Properties were measured using soil fractionation analysis. p values from ANOVA are displayed in the bottom row. Asterisks and crosses in the other rows (if present) indicate significant differences to uninoculated controls as determined via Dunnett's post hoc test († p<0.1, * p<0.05, p<0.01, p<0.001). C: carbon; N: nitrogen; AggC: aggregate carbon; MAOM: mineral-associated organic matter; POM: particulate organic matter.
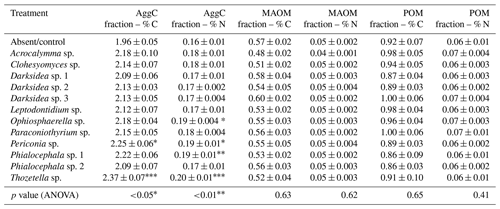
Table B5Plant variables potentially influencing soil (in which inoculated wheat plants were grown for 4 months). p values from ANOVA are displayed in the bottom rows. Asterisks and crosses in the other rows (if present) indicate significant differences to uninoculated controls as determined via Dunnett's post hoc test († p<0.1, * p<0.05, p<0.01, p<0.001). C: carbon; N: nitrogen.
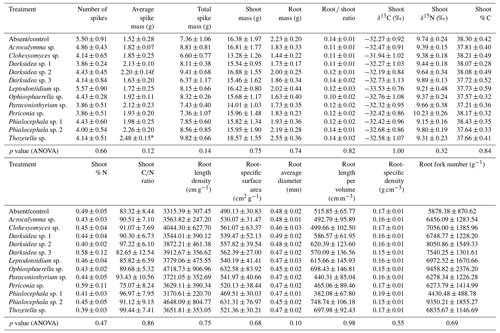
Table B6Microbial community variables potentially influencing soil (in which inoculated wheat plants were grown for 4 months). p values from ANOVA are displayed in the bottom row. Asterisks and crosses in the other rows (if present) indicate significant differences to uninoculated controls as determined via Dunnett's post hoc test († p<0.1, * p<0.05, p<0.01, p<0.001).
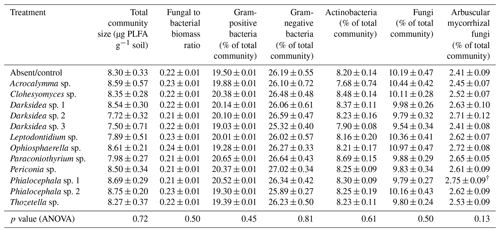
Table B7Fungal variables potentially influencing soil (in which inoculated wheat plants were grown for 4 months). p values from ANOVA are displayed in the bottom row (‡ p<0.1, * p<0.05, p<0.01, p<0.001). Different letters indicate significant differences between treatments as determined via Tukey's post hoc test. † indicates variables calculated using treatment averages. C: carbon; N: nitrogen.
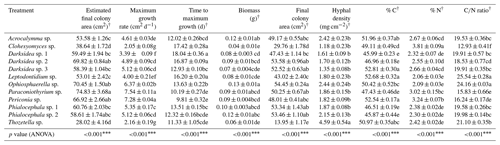
All of the data are published within this paper and are in the Supplement. The raw data used to create the figures are available on request.
The supplement related to this article is available online at: https://doi.org/10.5194/bg-21-1037-2024-supplement.
YC, JRP, and LCG designed the study. EKS, LCG, YC, and WB performed the research. ESK and YC analysed the data. EKS wrote the first draft of the manuscript, and all the authors contributed to revisions.
The research was partially funded by SoilCQuest2031, who provided the fungal cultures and soil. This funding was provided independently of research findings. SoilCQuest2031 did not attempt to influence the interpretations or conclusions of the work. The authors declare that the research was conducted in the absence of any commercial or financial relationships that could be construed as a potential conflict of interest.
Publisher’s note: Copernicus Publications remains neutral with regard to jurisdictional claims made in the text, published maps, institutional affiliations, or any other geographical representation in this paper. While Copernicus Publications makes every effort to include appropriate place names, the final responsibility lies with the authors.
We acknowledge assistance from Guy Webb and Suresh Subashchandrabose for providing the soils and cultures. We also thank Andrew Gherlenda for assistance with the growth chamber experiment; Russell Thomson for help with non-linear least square curve fitting; the UC Davis Stable Isotope Facility; the Environmental Analysis Laboratory and Pushpinder Matta for running the nutrient analyses; and Sophia Bruna, Hui Zhang, and Asel Weerasekara for help with the experiment harvest.
This project was supported by the Western Sydney University Research Partnerships Program and by SoilCQuest2031 (Orange, New South Wales, Australia).
This paper was edited by Kees Jan van Groenigen and reviewed by Ashley Lang and Guopeng Liang.
Addy, H. D., Piercey, M. M., and Currah, R. S.: Microfungal endophytes in roots, Can. J. Botany, 83, 1–13, https://doi.org/10.1139/b04-171, 2005.
Andrade, R., Pascoal, C., and Cássio, F.: Effects of inter and intraspecific diversity and genetic divergence of aquatic fungal communities on leaf litter decomposition – a microcosm experiment, FEMS Microbiol. Ecol., 92, fiw102, https://doi.org/10.1093/femsec/fiw102, 2016.
Averill, C. and Hawkes, C. V.: Ectomycorrhizal fungi slow soil carbon cycling, Ecol. Lett., 19, 937–947, https://doi.org/10.1111/ele.12631, 2016.
Berg, B. and McClaugherty, C.: Plant Litter. Decomposition, Humus Formation, Carbon Sequestration, 1, Springer, Berlin, Heidelberg, Germany, 286 pp., https://doi.org/10.1007/978-3-662-05349-2, 2014.
Berthelot, C., Perrin, Y., Leyval, C., and Blaudez, D.: Melanization and ageing are not drawbacks for successful agro-transformation of dark septate endophytes, Fungal Biol.-UK, 121, 652–663, https://doi.org/10.1016/j.funbio.2017.04.004, 2017.
Bödeker, I. T. M., Lindahl, B. D., Olson, Å., and Clemmensen, K. E.: Mycorrhizal and saprotrophic fungal guilds compete for the same organic substrates but affect decomposition differently, Funct. Ecol., 30, 1967–1978, https://doi.org/10.1111/1365-2435.12677, 2016.
Boer, W. d., Folman, L. B., Summerbell, R. C., and Boddy, L.: Living in a fungal world: impact of fungi on soil bacterial niche development, FEMS Microbiol. Rev., 29, 795–811, https://doi.org/10.1016/j.femsre.2004.11.005, 2005.
Buss, W., Sharma, R., Ferguson, S., Borevitz, J.: Soil organic carbon fractionation and metagenomics pipeline to link carbon content and stability with microbial composition – First results investigating fungal endophytes, bioRxiv [preprint], https://doi.org/10.1101/2021.12.19.473394, 2021.
Buyer, J. S. and Sasser, M.: High throughput phospholipid fatty acid analysis of soils, Appl. Soil Ecol., 61, 127–130, https://doi.org/10.1016/j.apsoil.2012.06.005, 2012.
Camenzind, T., Lehmann, A., Ahland, J., Rumpel, S., and Rillig, M. C.: Trait-based approaches reveal fungal adaptations to nutrient-limiting conditions, Environ. Microbiol., 22, 3548–3560, https://doi.org/10.1111/1462-2920.15132, 2020.
Carney, K. M., Hungate, B. A., Drake, B. G., and Megonigal, J. P.: Altered soil microbial community at elevated CO2 leads to loss of soil carbon, P. Natl. Acad. Sci. USA, 104, 4990–4995, https://doi.org/10.1073/pnas.0610045104, 2007.
Carrillo, Y., Pendall, E., Dijkstra, F. A., Morgan, J. A., and Newcomb, J. M.: Response of soil organic matter pools to elevated CO2 and warming in a semi-arid grassland, Plant Soil, 347, 339–350, https://doi.org/10.1007/s11104-011-0853-4, 2011.
Cheng, W. and Dijkstra, F. A.: Theoretical proof and empirical confirmation of a continuous labeling method using naturally 13C-depleted carbon dioxide, J. Integr. Plant Biol., 49, 401–407, https://doi.org/10.1111/j.1744-7909.2007.00387.x, 2007.
Clocchiatti, A., Hannula, S. E., van den Berg, M., Korthals, G., and de Boer, W.: The hidden potential of saprotrophic fungi in arable soil: Patterns of short-term stimulation by organic amendments, Appl. Soil Ecol., 147, 103434, https://doi.org/10.1016/j.apsoil.2019.103434, 2020.
Cotrufo, M. F. and Lavallee, J. M.: Soil organic matter formation, persistence, and functioning: A synthesis of current understanding to inform its conservation and regeneration, in: Advances in Agronomy, edited by: Sparks, D. L., Academic Press, 1–66, https://doi.org/10.1016/bs.agron.2021.11.002, 2022.
Derrien, D., Barré, P., Basile-Doelsch, I., Cécillon, L., Chabbi, A., Crème, A., Fontaine, S., Henneron, L., Janot, N., Lashermes, G., Quénéa, K., Rees, F., and Dignac, M.-F.: Current controversies on mechanisms controlling soil carbon storage: implications for interactions with practitioners and policy-makers. A review, Agron. Sustain. Dev., 43, 28 pp., https://doi.org/10.1007/s13593-023-00876-x, 2023.
Dignac, M.-F., Bahri, H., Rumpel, C., Rasse, D., Bardoux, G., Balesdent, J., Cyril, G., Chenu, C., and Mariotti, A.: Carbon-13 natural abundance as a tool to study the dynamics of lignin monomers in soil: An appraisal at the Closeaux experimental field (France), Geoderma, 128, 3–17, https://doi.org/10.1016/j.geoderma.2004.12.022, 2005.
Dignac, M.-F., Derrien, D., Barré, P., Barot, S., Cécillon, L., Chenu, C., Chevallier, T., Freschet, G. T., Garnier, P., Guenet, B., Hedde, M., Klumpp, K., Lashermes, G., Maron, P.-A., Nunan, N., Roumet, C., and Basile-Doelsch, I.: Increasing soil carbon storage: mechanisms, effects of agricultural practices and proxies. A review, Agron. Sustain. Dev., 37, 27 pp., https://doi.org/10.1007/s13593-017-0421-2, 2017.
Dynarski, K. A., Bossio, D. A., and Scow, K. M.: Dynamic stability of soil carbon: reassessing the “permanence” of soil carbon sequestration, Front. Environ. Sci., 8, 514701, https://doi.org/10.3389/fenvs.2020.514701, 2020.
Fernandez, C. W. and Kennedy, P. G.: Melanization of mycorrhizal fungal necromass structures microbial decomposer communities, J. Ecol., 106, 468–479, https://doi.org/10.1111/1365-2745.12920, 2018.
Fernandez, C. W. and Koide, R. T.: The function of melanin in the ectomycorrhizal fungus Cenococcum geophilum under water stress, Fungal Ecol., 6, 479–486, https://doi.org/10.1016/j.funeco.2013.08.004, 2013.
Fernandez, C. W., Heckman, K., Kolka, R., and Kennedy, P. G.: Melanin mitigates the accelerated decay of mycorrhizal necromass with peatland warming, Ecol. Lett., 22, 498–505, https://doi.org/10.1111/ele.13209, 2019.
Field, C. and Raupach, M.: The global carbon cycle: integrating humans, climate, and the natural world, SCOPE, Island Press, ISBN: 1559635266, 2004.
Frąc, M., Hannula, S. E., Bełka, M., and Jędryczka, M.: Fungal biodiversity and their role in soil health, Front. Microbiol., 9, 707, https://doi.org/10.3389/fmicb.2018.00707, 2018.
Frey, S. D.: Mycorrhizal fungi as mediators of soil organic matter dynamics, Annu. Rev. Ecol. Evol. S., 50, 237–259, https://doi.org/10.1146/annurev-ecolsys-110617-062331, 2019.
Gadgil, R. L. and Gadgil, P. D.: Mycorrhiza and litter decomposition, Nature, 233, 133–133, https://doi.org/10.1038/233133a0, 1971.
Hannula, S. E. and Morriën, E.: Will fungi solve the carbon dilemma?, Geoderma, 413, 115767, https://doi.org/10.1016/j.geoderma.2022.115767, 2022.
He, C., Wang, W., and Hou, J.: Characterization of dark septate endophytic fungi and improve the performance of liquorice under organic residue treatment, Front. Microbiol., 10, 1364, https://doi.org/10.3389/fmicb.2019.01364, 2019.
Hemingway, J. D., Rothman, D. H., Grant, K. E., Rosengard, S. Z., Eglinton, T. I., Derry, L. A., and Galy, V. V.: Mineral protection regulates long-term global preservation of natural organic carbon, Nature, 570, 228–231, https://doi.org/10.1038/s41586-019-1280-6, 2019.
Hiscox, J., Savoury, M., Vaughan, I. P., Müller, C. T., and Boddy, L.: Antagonistic fungal interactions influence carbon dioxide evolution from decomposing wood, Fungal Ecol., 14, 24–32, https://doi.org/10.1016/j.funeco.2014.11.001, 2015.
Hossain, M. M., Sultana, F., and Islam, S.: Plant Growth-Promoting Fungi (PGPF): Phytostimulation and Induced Systemic Resistance, in: Plant-Microbe Interactions in Agro-Ecological Perspectives: Volume 2: Microbial Interactions and Agro-Ecological Impacts, edited by: Singh, D. P., Singh, H. B., and Prabha, R., Springer, Singapore, 135–191, https://doi.org/10.1007/978-981-10-6593-4_6, 2017.
Islam, M. N., Germida, J. J., and Walley, F. L.: Survival of a commercial AM fungal inoculant and its impact on indigenous AM fungal communities in field soils, Appl. Soil Ecol., 166, 103979, https://doi.org/10.1016/j.apsoil.2021.103979, 2021.
Islam, M. R., Singh, B., and Dijkstra, F. A.: Stabilisation of soil organic matter: interactions between clay and microbes, Biogeochemistry, 160, 145–158, https://doi.org/10.1007/s10533-022-00956-2, 2022.
Jackson, R. B., Lajtha, K., Crow, S. E., Hugelius, G., Kramer, M. G., and Piñeiro, G.: The ecology of soil carbon: pools, vulnerabilities, and biotic and abiotic controls, Annu. Rev. Ecol. Evol. S., 48, 419–445, https://doi.org/10.1146/annurev-ecolsys-112414-054234, 2017.
Jian, S., Li, J., Wang, G., Kluber, L. A., Schadt, C. W., Liang, J., and Mayes, M. A.: Multi-year incubation experiments boost confidence in model projections of long-term soil carbon dynamics, Nat. Commun., 11, 5864, https://doi.org/10.1038/s41467-020-19428-y, 2020.
Johnson, D., Martin, F., Cairney, J. W. G., and Anderson, I. C.: The importance of individuals: intraspecific diversity of mycorrhizal plants and fungi in ecosystems, New Phytol., 194, 614–628, https://doi.org/10.1111/j.1469-8137.2012.04087.x, 2012.
Juan-Ovejero, R., Briones, M. J. I., and Öpik, M.: Fungal diversity in peatlands and its contribution to carbon cycling, Appl. Soil Ecol., 146, 103393, https://doi.org/10.1016/j.apsoil.2019.103393, 2020.
Kallenbach, C. M., Frey, S. D., and Grandy, A. S.: Direct evidence for microbial-derived soil organic matter formation and its ecophysiological controls, Nat. Commun., 7, 13630, https://doi.org/10.1038/ncomms13630, 2016.
Kaminsky, L. M., Trexler, R. V., Malik, R. J., Hockett, K. L., and Bell, T. H.: The inherent conflicts in developing soil microbial inoculants, Trends Biotechnol., 37, 140–151, https://doi.org/10.1016/j.tibtech.2018.11.011, 2019.
Kleber, M., Nico, P. S., Plante, A., Filley, T., Kramer, M., Swanston, C., and Sollins, P.: Old and stable soil organic matter is not necessarily chemically recalcitrant: implications for modeling concepts and temperature sensitivity, Glob. Change Biol., 17, 1097–1107, https://doi.org/10.1111/j.1365-2486.2010.02278.x, 2011.
Kopittke, P. M., Berhe, A. A., Carrillo, Y., Cavagnaro, T. R., Chen, D., Chen, Q.-L., Román Dobarco, M., Dijkstra, F. A., Field, D. J., Grundy, M. J., He, J.-Z., Hoyle, F. C., Kögel-Knabner, I., Lam, S. K., Marschner, P., Martinez, C., McBratney, A. B., McDonald-Madden, E., Menzies, N. W., Mosley, L. M., Mueller, C. W., Murphy, D. V., Nielsen, U. N., O'Donnell, A. G., Pendall, E., Pett-Ridge, J., Rumpel, C., Young, I. M., and Minasny, B.: Ensuring planetary survival: the centrality of organic carbon in balancing the multifunctional nature of soils, Crit. Rev. Environ. Sci. Technol., 52, 4308–4324, https://doi.org/10.1080/10643389.2021.2024484, 2022.
Lal, R.: Digging deeper: A holistic perspective of factors affecting soil organic carbon sequestration in agroecosystems, Glob. Change Biol., 24, 3285–3301, https://doi.org/10.1111/gcb.14054, 2018.
Langley, J. A., McKinley, D. C., Wolf, A. A., Hungate, B. A., Drake, B. G., and Megonigal, J. P.: Priming depletes soil carbon and releases nitrogen in a scrub-oak ecosystem exposed to elevated CO2, Soil Biol. Biochem., 41, 54–60, https://doi.org/10.1016/j.soilbio.2008.09.016, 2009.
Lehmann, A. and Rillig, M. C.: Arbuscular mycorrhizal contribution to copper, manganese and iron nutrient concentrations in crops – A meta-analysis, Soil Biol. Biochem., 81, 147–158, https://doi.org/10.1016/j.soilbio.2014.11.013, 2015.
Lehmann, A., Zheng, W., and Rillig, M. C.: Soil biota contributions to soil aggregation, Nat. Ecol. Evol., 1, 1828–1835, https://doi.org/10.1038/s41559-017-0344-y, 2017.
Lehmann, A., Zheng, W., Ryo, M., Soutschek, K., Roy, J., Rongstock, R., Maaß, S., and Rillig, M. C.: Fungal traits important for soil aggregation, Front. Microbiol., 10, 2904, https://doi.org/10.3389/fmicb.2019.02904, 2020.
Liang, C., Amelung, W., Lehmann, J., and Kästner, M.: Quantitative assessment of microbial necromass contribution to soil organic matter, Glob. Change Biol., 25, 3578–3590, https://doi.org/10.1111/gcb.14781, 2019.
Lützow, M. v., Kögel-Knabner, I., Ekschmitt, K., Matzner, E., Guggenberger, G., Marschner, B., and Flessa, H.: Stabilization of organic matter in temperate soils: mechanisms and their relevance under different soil conditions – a review, Eur. J. Soil Sci., 57, 426–445, https://doi.org/10.1111/j.1365-2389.2006.00809.x, 2006.
Mandyam, K. and Jumpponen, A.: Seeking the elusive function of the root-colonising dark septate endophytic fungi, Stud. Mycol., 53, 173–189, https://doi.org/10.3114/sim.53.1.173, 2005.
Marañón-Jiménez, S., Radujković, D., Verbruggen, E., Grau, O., Cuntz, M., Peñuelas, J., Richter, A., Schrumpf, M., and Rebmann, C.: Shifts in the abundances of saprotrophic and ectomycorrhizal fungi with altered leaf litter inputs, Front. Plant Sci., 12, 682142, https://doi.org/10.3389/fpls.2021.682142, 2021.
Mishra, A., Singh, L., and Singh, D.: Unboxing the black box – one step forward to understand the soil microbiome: A systematic review, Microb. Ecol., 85, 669–683, https://doi.org/10.1007/s00248-022-01962-5, 2023.
Mukasa Mugerwa, T. T. and McGee, P. A.: Potential effect of melanised endophytic fungi on levels of organic carbon within an Alfisol, Soil Res., 55, 245–252, https://doi.org/10.1071/SR16006, 2017.
Oksanen, J., Simpson, G., Blanchet, F., Kindt, R., Legendre, P., Minchin, P., O'Hara, R., Solymos, P., Stevens, M., Szoecs, E., Wagner, H., Barbour, M., Bedward, M., Bolker, B., Borcard, D., Carvalho, G., Chirico, M., De Caceres, M., Durand, S., Evangelista, H., FitzJohn, R., Friendly, M., Furneaux, B., Hannigan, G., Hill, M., Lahti, L., McGlinn, D., Ouellette, M., Ribeiro Cunha, E., Smith, T., Stier, A., ter Braak, C. J. F., and Weedon, J.: vegan: Community Ecology Package, R package version 2.6-4, https://CRAN.R-project.org/package=vegan2022/180124 (last access: 18 January 2024), 2022.
Plett, K. L., Kohler, A., Lebel, T., Singan, V. R., Bauer, D., He, G., Ng, V., Grigoriev, I. V., Martin, F., Plett, J. M., and Anderson, I. C.: Intra-species genetic variability drives carbon metabolism and symbiotic host interactions in the ectomycorrhizal fungus Pisolithus microcarpus, Environ. Microbiol., 23, 2004–2020, https://doi.org/10.1111/1462-2920.15320, 2021.
Poeplau, C., Kätterer, T., Leblans, N. I. W., and Sigurdsson, B. D.: Sensitivity of soil carbon fractions and their specific stabilization mechanisms to extreme soil warming in a subarctic grassland, Glob. Change Biol., 23, 1316–1327, https://doi.org/10.1111/gcb.13491, 2017.
Poeplau, C., Don, A., Six, J., Kaiser, M., Benbi, D., Chenu, C., Cotrufo, M. F., Derrien, D., Gioacchini, P., Grand, S., Gregorich, E., Griepentrog, M., Gunina, A., Haddix, M., Kuzyakov, Y., Kühnel, A., Macdonald, L. M., Soong, J., Trigalet, S., Vermeire, M.-L., Rovira, P., van Wesemael, B., Wiesmeier, M., Yeasmin, S., Yevdokimov, I., and Nieder, R.: Isolating organic carbon fractions with varying turnover rates in temperate agricultural soils – A comprehensive method comparison, Soil Biol. Biochem., 125, 10–26, https://doi.org/10.1016/j.soilbio.2018.06.025, 2018.
R Core Team: R: A Language and Environment for Statistical Computing. R Foundation for Statistical Computing, Vienna, Austria, 2021.
Rai, M. and Agarkar, G.: Plant–fungal interactions: What triggers the fungi to switch among lifestyles?, Crit. Rev. Microbiol., 42, 428–438, https://doi.org/10.3109/1040841X.2014.958052, 2016.
Salomon, M. J., Watts-Williams, S. J., McLaughlin, M. J., Bücking, H., Singh, B. K., Hutter, I., Schneider, C., Martin, F. M., Vosatka, M., Guo, L., Ezawa, T., Saito, M., Declerck, S., Zhu, Y.-G., Bowles, T., Abbott, L. K., Smith, F. A., Cavagnaro, T. R., and van der Heijden, M. G. A.: Establishing a quality management framework for commercial inoculants containing arbuscular mycorrhizal fungi, iScience, 25, 104636, https://doi.org/10.1016/j.isci.2022.104636, 2022.
Scharlemann, J. P. W., Tanner, E. V. J., Hiederer, R., and Kapos, V.: Global soil carbon: understanding and managing the largest terrestrial carbon pool, Carbon Manag., 5, 81–91, https://doi.org/10.4155/cmt.13.77, 2014.
Schlesinger, W. H.: Evidence from chronosequence studies for a low carbon-storage potential of soils, Nature, 348, 232–234, https://doi.org/10.1038/348232a0, 1990.
Schmidt, M. W. I., Torn, M. S., Abiven, S., Dittmar, T., Guggenberger, G., Janssens, I. A., Kleber, M., Kögel-Knabner, I., Lehmann, J., Manning, D. A. C., Nannipieri, P., Rasse, D. P., Weiner, S., and Trumbore, S. E.: Persistence of soil organic matter as an ecosystem property, Nature, 478, 49–56, https://doi.org/10.1038/nature10386, 2011.
Schnecker, J., Bowles, T., Hobbie, E. A., Smith, R. G., and Grandy, A. S.: Substrate quality and concentration control decomposition and microbial strategies in a model soil system, Biogeochemistry, 144, 47–59, https://doi.org/10.1007/s10533-019-00571-8, 2019.
Schneider, C. A., Rasband, W. S., and Eliceiri, K. W.: NIH Image to ImageJ: 25 years of image analysis, Nat. Methods, 9, 671–675, https://doi.org/10.1038/nmeth.2089, 2012.
Smith, G. R. and Wan, J.: Resource-ratio theory predicts mycorrhizal control of litter decomposition, New Phytol., 223, 1595–1606, https://doi.org/10.1111/nph.15884, 2019.
Smith, S. and Read, D.: Mycorrhizal Symbiosis, Academic Press, 787 pp., https://doi.org/10.1016/B978-0-12-370526-6.X5001-6, 2008.
Sokol, N. W., Sanderman, J., and Bradford, M. A.: Pathways of mineral-associated soil organic matter formation: Integrating the role of plant carbon source, chemistry, and point of entry, Glob. Change Biol., 25, 12–24, https://doi.org/10.1111/gcb.14482, 2019.
Starke, R., Mondéjar, R. L., Human, Z. R., Navrátilová, D., Štursová, M., Větrovský, T., Olson, H. M., Orton, D. J., Callister, S. J., Lipton, M. S., Howe, A., McCue, L. A., Pennacchio, C., Grigoriev, I., and Baldrian, P.: Niche differentiation of bacteria and fungi in carbon and nitrogen cycling of different habitats in a temperate coniferous forest: A metaproteomic approach, Soil Biol. Biochem., 155, 108170, https://doi.org/10.1016/j.soilbio.2021.108170, 2021.
Stuart, E. K., Castañeda-Gómez, L., Macdonald, C. A., Wong-Bajracharya, J., Anderson, I. C., Carrillo, Y., Plett, J. M., and Plett, K. L.: Species-level identity of Pisolithus influences soil phosphorus availability for host plants and is moderated by nitrogen status, but not CO2, Soil Biol. Biochem., 165, 108520, https://doi.org/10.1016/j.soilbio.2021.108520, 2022.
Taneva, L. and Gonzalez-Meler, M. A.: Decomposition kinetics of soil carbon of different age from a forest exposed to 8 years of elevated atmospheric CO2 concentration, Soil Biol. Biochem., 40, 2670–2677, https://doi.org/10.1016/j.soilbio.2008.07.013, 2008.
Tiedje, J. M., Asuming-Brempong, S., Nüsslein, K., Marsh, T. L., and Flynn, S. J.: Opening the black box of soil microbial diversity, Appl. Soil Ecol., 13, 109–122, https://doi.org/10.1016/S0929-1393(99)00026-8, 1999.
von Unger, M. and Emmer, I.: Carbon Market Incentives to Conserve, Restore and Enhance Soil Carbon, Silvestrum Climate Associates LLC. and The Nature Conservancy, Arlington, VA, USA, https://www.nature.org/content/dam/tnc/nature/en/documents/Carbon-Market-Incentives-Report.pdf (last access: 4 September 2023), 2018.
Wang, B., Liang, C., Yao, H., Yang, E., and An, S.: The accumulation of microbial necromass carbon from litter to mineral soil and its contribution to soil organic carbon sequestration, CATENA, 207, 105622, https://doi.org/10.1016/j.catena.2021.105622, 2021.
Wedin, D. A. and Pastor, J.: Nitrogen mineralization dynamics in grass monocultures, Oecologia, 96, 186–192, https://doi.org/10.1007/BF00317731, 1993.
Zak, D. R., Pellitier, P. T., Argiroff, W., Castillo, B., James, T. Y., Nave, L. E., Averill, C., Beidler, K. V., Bhatnagar, J., Blesh, J., Classen, A. T., Craig, M., Fernandez, C. W., Gundersen, P., Johansen, R., Koide, R. T., Lilleskov, E. A., Lindahl, B. D., Nadelhoffer, K. J., Phillips, R. P., and Tunlid, A.: Exploring the role of ectomycorrhizal fungi in soil carbon dynamics, New Phytol., 223, 33–39, https://doi.org/10.1111/nph.15679, 2019.
Zanne, A. E., Abarenkov, K., Afkhami, M. E., Aguilar-Trigueros, C. A., Bates, S., Bhatnagar, J. M., Busby, P. E., Christian, N., Cornwell, W. K., Crowther, T. W., Flores-Moreno, H., Floudas, D., Gazis, R., Hibbett, D., Kennedy, P., Lindner, D. L., Maynard, D. S., Milo, A. M., Nilsson, R. H., Powell, J., Schildhauer, M., Schilling, J., and Treseder, K. K.: Fungal functional ecology: bringing a trait-based approach to plant-associated fungi, Biol. Rev., 95, 409–433, https://doi.org/10.1111/brv.12570, 2020.
Zhu, Y.-G. and Michael Miller, R.: Carbon cycling by arbuscular mycorrhizal fungi in soil–plant systems, Trends Plant Sci., 8, 407–409, https://doi.org/10.1016/S1360-1385(03)00184-5, 2003.