the Creative Commons Attribution 4.0 License.
the Creative Commons Attribution 4.0 License.
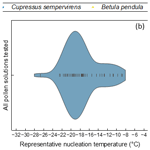
High interspecific variability in ice nucleation activity suggests pollen ice nucleators are incidental
Nina L. H. Kinney
Charles A. Hepburn
Matthew I. Gibson
Daniel Ballesteros
Thomas F. Whale
Ice-nucleating macromolecules (INMs) produced by plant pollen can nucleate ice at warm temperatures and may play an important role in weather- and climate-relevant cloud glaciation. INMs have also proved useful for mammalian cell and tissue model cryopreservation. The high ice nucleation (IN) activity of some INMs indicates an underlying biological function, either freezing tolerance or bioprecipitation-mediated dispersal. Here, using the largest study of pollen ice nucleation to date, we show that phylogenetic proximity, spermatophyte subdivision, primary growth biome, pollination season, primary pollination method, desiccation tolerance and native growth elevation do not account for the IN activity of INMs released from different plant species' pollen. The results suggest that these macromolecules are produced by plants for a purpose unrelated to ice nucleation and have an incidental ability to nucleate ice. This ability may have been adapted by some species for specific biological purposes, producing exceptional ice nucleators. Pollen INMs may be more active, widespread in nature, and diverse than previously thought.
- Article
(1078 KB) - Full-text XML
-
Supplement
(998 KB) - BibTeX
- EndNote
Below 0 °C ice is the thermodynamically stable form of water, yet liquid water is routinely observed to persist to temperatures as low as −40 °C because the nucleation of ice is severely kinetically hindered. In nature ice forms usually heterogeneously, stimulated by contact with a surface which can reduce the free energy barrier to nucleation. Of the many substances known to nucleate ice, the particles found to induce ice formation at the warmest temperatures are of biological origin (Szyrmer and Zawadzki, 1997; O'Sullivan et al., 2015; Knopf and Alpert, 2023; Cornwell et al., 2023). The identification of ice nucleators associated with various organisms, from bacteria (Lindow et al., 1982; Maki et al., 1974; Lukas et al., 2022) to lichens (Kieft, 1988; Eufemio et al., 2023) and plants (Gross et al., 1988; Brush et al., 1994; Felgitsch et al., 2018), is of biological and atmospheric interest. Better understanding the properties of airborne ice-nucleating particles (INPs), and particularly those active at warmer temperatures where secondary ice production mechanisms ensue (Hallett and Mossop, 1974), is essential to evaluate their atmospheric impact and reduce uncertainties in understanding weather and climate (DeMott and Prenni, 2010; Murray et al., 2012, 2021; Kanji et al., 2017; Hummel et al., 2018; Huang et al., 2021). Pollen grains are the male gametophytes of the seed plants or spermatophytes, a clade that is divided into gymnosperms (plants with unenclosed or “naked seeds”) and angiosperms (or flowering plants). Angiosperms predominantly rely on mutualistic relationships with animal pollinators for transporting pollen to the female stigma. There, pollen develops a pollinic tube that transports the spermatic nuclei into the ovule, where the female gametophyte is located and fertilisation occurs, enabling the production of seeds. Anemophily (wind pollination) is the primary pollination method for most gymnosperms and around 10 % of angiosperm species (Friedman and Barrett, 2009). Seasonally, anemophilous plants release large quantities of pollen into the air. Pollen grains and smaller pollen fragments are classed as primary biological aerosol particles and are known to disperse in the atmosphere (Després et al., 2012). Records of the long-distance transport of pollen and its presence in rain, hail and snow samples have appeared in historical literature (Bessey, 1883; Potter and Rowley, 1960; Gregory, 1961), pointing to the possible relevance of pollen for ice nucleation in mixed-phase clouds.
It is established that the pollen of some plants releases water-soluble ice-nucleating macromolecules (INMs) that can promote ice nucleation (Pummer et al., 2012; Dreischmeier et al., 2017). The ice nucleation (IN) activity of pollen grains in suspension is found to agree with the IN activity of pollen solutions following removal of the grains and insoluble fragments by filtration (Pummer et al., 2012; Dreischmeier et al., 2017; Gute et al., 2020), indicating that pollen INMs readily separate from their parent pollen grains on contact with water. The IN activity of pollen samples can therefore be evaluated by measuring nucleation temperatures of filtered pollen solutions. The quantity of soluble material in a filtered pollen solution has been recorded for a handful of pollen types (Pummer et al., 2012; Murray et al., 2022), but the proportion of this material that nucleates ice is estimated to be far smaller (Murray et al., 2022). Both the chemical nature and biological function of these INMs remain unclear. Growing evidence suggests that polysaccharides are not exclusively responsible for pollens' ability to nucleate ice. Burkart et al. (2021) and Wieland et al. (2024) present evidence that proteins in Betula pendula pollen solution play a role in its IN activity. Improved understanding of the biological function of pollen INMs would facilitate prediction of their likely spatial and temporal distribution in the atmosphere. There are two obvious hypotheses for the biological function of pollen ice nucleators.
Firstly, intrinsically ice-nucleating pollen grains may be more able to survive freezing temperatures, either during development or on dispersal. This would improve their ability to survive freezing conditions to the point of fulfilling their purpose of fertilising female plant gametophytes. Some plants are known to survive cold temperatures through so-called “freeze tolerance” where extracellular ice formation at warm temperatures is encouraged (Zachariassen and Kristiansen, 2000). Extracellular ice formation enables intracellular dehydration which favours solidification of cells in a glassy state, facilitating post-thaw survival rather than damaging intracellular ice formation. A similar mechanism may be employed by pollen grains, particularly when they survive drying and the formation of the glassy state (e.g. desiccation tolerant or “orthodox” pollen). The idea that there may be some relationship between the IN activity of pollen and cold tolerance was first posited by Diehl et al. (2002) and von Blohn et al. (2005). This was based on their observation that high IN activity, of eight pollen samples tested across both studies, seemed to correlate with earlier plant pollination times and therefore pollen transport occurring when it was colder. Pummer et al. (2012) similarly noted an apparent dependence of IN activity on geographical distribution and pollination times of plants, with some exceptions. It is worth noting here that pollen INMs have proven to be useful for cryopreservation of mammalian cell suspensions, monolayers and spheroids, where control of ice nucleation yields greatly improved outcomes (Murray et al., 2022, 2023; Gao et al., 2023). While there are important differences in the biology of animal and plant cells, the fundamental point that avoidance of intracellular ice formation is critical carries across both kingdoms.
Secondly, it may be that pollen INMs favour bioprecipitation, where the INPs generated by pollen promote the formation of hydrometeors and the distribution of pollen via the water cycle. The concept of bioprecipitation as an ecological advantage has been discussed for other organisms associated with potent ice nucleators (Morris et al., 2004; Kunert et al., 2019). It is not clear what role these INMs play in preserving the viability of pollen grains, which are susceptible to rupturing under atmospheric conditions (Grote et al., 2003; Taylor et al., 2004; Wozniak et al., 2018; Subba et al., 2023). Yet, various pine pollens have been shown to retain capacity for germination following immersion freezing (Williams, 2013).
Finally, it should be recognised that pollen INMs may have arisen incidentally, serving some other biological function and nucleating ice by happenstance. The concept of adaptive biological ice nucleators, where IN activity affords an advantage that indicates selectivity in evolution, was explored by Lundheim (2002). Ice-active molecules found in plant tissues control nucleation temperature and ice growth, underpinning mechanisms of frost tolerance or avoidance (Ambroise et al., 2020; Zachariassen and Kristiansen, 2000). There are examples in nature of ice nucleators present where control over the formation of ice clearly helps to protect against frost damage, such as the presence of ice nucleators in the extracellular space that increase with cold exposure (Zachariassen and Hammel, 1976). While it is difficult to dismiss the possibility that IN activity is an incidental property, it is well known that some biological ice nucleators provide a distinct biological advantage; for others the case is less clear.
To date, pollen samples from only 30 plant species have been tested in immersion mode ice nucleation experiments across the literature, scratching the surface of spermatophyte diversity (Diehl et al., 2002; von Blohn et al., 2005; Pummer et al., 2012; Tong et al., 2015; Dreischmeier et al., 2017; Gute and Abbatt, 2020). Most pollen tested (83 %) is considered desiccation tolerant, and measurements of desiccation sensitive (“recalcitrant”) pollen have been restricted to the grasses (Poaceae). Notably lacking are measurements of the IN activity of pollen from primarily animal-pollinated plant species. Understandably, atmospheric studies have focused on pollen types which are likely to be seasonally abundant in the air and that could impact cloud properties.
We have collected 51 pollen samples from 50 distinct plant species, harnessing the extensive living collection held by the Royal Botanic Gardens, Kew, UK, at their Richmond and Wakehurst sites, and tested their IN activities using a microlitre droplet freezing assay. This instrument, described in detail by Whale (2024), freezes around fifty 1 µL droplets allowing determination of the IN activity of a sample. We evaluate the IN activity of pollen solutions (also commonly referred to as pollen-washing waters) which are prepared by filtering aqueous pollen suspensions, removing pollen grains and insoluble fragments and leaving only soluble extracts in solution. Previous investigations have focussed mainly on measuring the IN activity of pollen from wind-pollinated trees, with some grass species (Gute and Abbatt, 2020; Duan et al., 2023). In this study we explicitly aimed to obtain pollen from a cross section of the plant phylogenetic tree. In doing so, we also examined species occupying various ecological niches and possessing a range of traits. Table S1 in the Supplement reports details of collections and of the classifications of other plant characteristics reported later in this article.
Droplet fraction frozen curves for five of the tested species are presented in Fig. 1a. Similar plots for all 51 tested samples are shown in Fig. S1. It can be seen in these two figures that the freezing temperatures for pollen solutions range from as warm as −5.5 °C to cold temperatures near the homogeneous freezing threshold for 1 µL droplets. These cold-temperature points are in some cases close to the background freezing temperature of the instrument used, shown in Fig. 1a. Figure 1b shows the cumulative number of nucleation sites per gram pollen, nm(T), for all pollen samples, calculated using Eq. (2). Immersion mode ice nucleation measurements of five filtered pollen solutions resulted in mean freezing temperatures above −10 °C, with the first droplets freezing as warm as −5.5 °C for Pinus mugo. Seventeen samples had at least some droplets freezing above −10 °C (see Supplement Figs. S1 and S2). Only one other sample has previously been found to have a mean freezing temperature value above −10 °C (Gute and Abbatt, 2020) meaning these observations of high temperature IN activity are clearly of substantial interest, showing that multiple species can produce highly active INMs. Figure 1c shows the frequency of occurrence nm(T)=105 g−1 across the 51 pollen samples tested. This threshold, henceforth Trep, was chosen as a reasonable response variable for statistical analysis. The choice of this threshold is justified in the methods section. The majority of species cross Trep in the range of −16 to −22 °C with the peak at −19 °C. A considerable number of species cross Trep at warmer temperatures. To summarise, all pollen solutions tested nucleated ice to some measurable extent. Some nucleate ice at much warmer temperatures, with many droplets freezing above −10 °C. Pollen from Musa rubra, a species of wild banana, was a cold outlier. We now investigate whether phylogenetic relationships or various environmental factors can account for the observed variation in ice nucleation temperature between different pollen solutions.
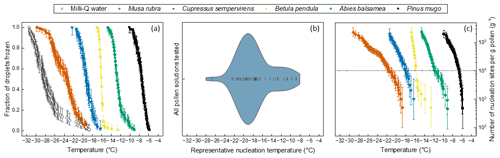
Figure 1Summary of the variation observed in the IN activity of pollen samples tested. (a) Fraction of droplets frozen against temperature for 1 µL droplets of Milli-Q water background and five species' pollen solutions, selected to show the extent of the variation in activity: Musa rubra, Cupressus sempervirens, Betula pendula, Abies balsamea and Pinus mugo. (b) Violin plot showing the distribution of representative nucleation temperature, Trep (T at nm(T)=105 g−1), of pollen solutions for all 51 samples tested. (c) Number of nucleation sites per gram pollen, nm(T), against temperature for Musa rubra, Cupressus sempervirens, Betula pendula, Abies balsamea and Pinus mugo solutions with dotted line at nm(T)=105 g−1 corresponding to the representative nucleation temperature points.
2.1 Phylogenetic study
To establish the phylogenetic relationships between the species tested, we used Plants of the World Online database (Royal Botanic Gardens Kew, 2023) to find accepted species names and phyloT to generate corresponding National Center for Biotechnology Information (NCBI) tree elements, which were then visualised using the Interactive Tree Of Life (iTOL). Of the 51 pollen collections in this study, we include results for 50 distinct species in the phylogenetic study. Cedrus atlantica f. glauca is distinguished from Cedrus atlantica at a secondary taxon level, below variety, so it is not a distinct species with an individual NCBI number and was therefore omitted from this analysis. For the artificial hybrid Nymphaea “Kew's Stowaway Blues”, the NCBI number used for the phylogenetic tree corresponds to a parent of the hybrid: Nymphaea carpentariae.
We aimed to measure nucleation temperatures of pollen samples from across the phylogeny. While we have collected samples from representatives of most major plant families, there are some notable absences. Figure 2 shows a phylogenetic tree with the level of IN activity associated with the named species pollen indicated by the length of the blue bars; a long bar indicates high IN activity and a short bar indicates low IN activity. The length of the blue bar was calculated from the representative nucleation temperature of the named species' pollen minus the lowest representative nucleation temperature value (Musa rubra Trep) plus one. This visualisation makes clear that the presence of INMs in pollen is not exclusive to a single plant taxon and confirms it is a more general pollen feature, as suggested by von Blohn et al. (2005). In total, there is no clear relationship between phylogenetic proximity and IN activity of the 50 species' pollen tested in this study.
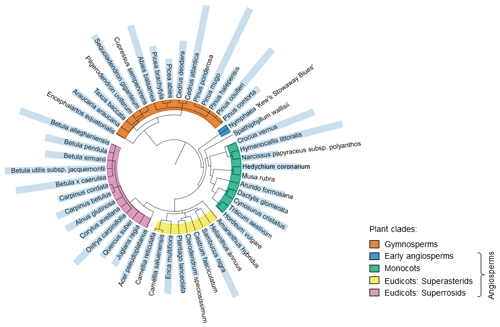
Figure 2Phylogenetic tree showing the level of IN activity associated with the named species pollen indicated by the length of the blue bars; a short bar indicates low IN activity and vice versa. The length of the blue bar is calculated from the representative nucleation temperature of the named species' pollen minus the lowest representative nucleation temperature value (Musa rubra Trep) plus one. The main plant clades of species sampled for this study are indicated by coloured arcs. Orange is used for gymnosperms and other colours for angiosperms: blue (early angiosperms), green (monocots), yellow (eudicots: superasterids) and pink (eudicots: superrosids).
It is at least possible that testing a greater number of pollen samples would reveal trends in activity that the present study does not identify. Given that most of our representative nucleation temperature results fell between −17 and −22 °C, as shown in Fig. 1b, it is unsurprising that there is such general consistency in IN activity across the phylogenetic tree. We note however that the more active samples are not clustered in any one area of the phylogenetic tree suggesting that exceptional pollen IN activity is not associated with a specific clade. As closely related plants can occupy quite different ecological niches, it remains possible that environmental factors tend to determine a pollen's ability to nucleate ice. As such, we next divide the set of plants examined by various characteristics to determine whether these impact IN activity of the pollen INMs the plants produce.
2.2 Plant characteristics and pollen ice nucleation ability
Figure 3 shows boxplots for the 51 pollen samples studied, divided by spermatophyte subdivision, primary growth biome, pollination season, primary pollination method, pollen desiccation tolerance and categorised growth elevation to ascertain whether these factors influence the IN activity of pollen. The following subsections discuss the dependency of IN activity on individual plant features. In this case our selected plant features, the explanatory variables, have complex relationships and cannot be considered independent. For example, wind pollination is prevalent amongst gymnosperm species, and tropical species are more likely to be pollinated by animals. We therefore did not measure the joint dependency of plant features on nucleation temperature and instead looked at individual variable influence.
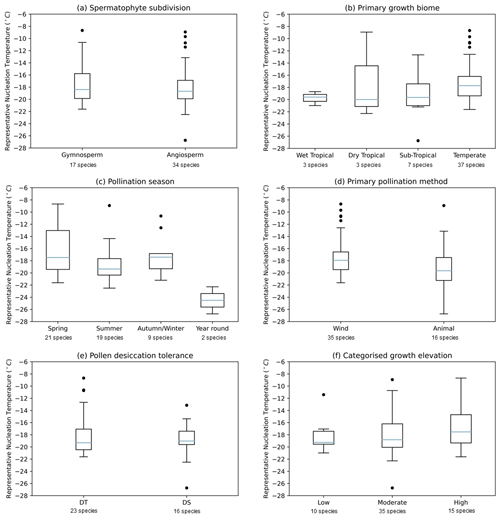
Figure 3Boxplots showing the distribution of temperature at nm(T)=100 000 g−1 for 51 pollen solutions grouped by (a) spermatophyte subdivision, (b) primary growth biome, (c) pollination season, (d) primary pollination method, (e) desiccation tolerance of pollen grains and (f) categorised growth elevation. The centre line of each box shows the median value for the dataset, the box shows the interquartile range, the whiskers show the maximum and minimum values, while points show outliers.
2.3 Spermatophyte subdivision
The spermatophytes, or seed-bearing plants, are commonly subdivided into two major groups: the angiosperms and gymnosperms (see Fig. 2). Angiosperms, the flowering plants, are the most ecologically diverse group, employing a wide variety of pollination strategies (Bell et al., 2010). Gymnosperms, on the other hand, are non-flowering and are considered to comprise the more “ancient” lineages (Wang and Ran, 2014). The conifers are the largest of four extant gymnosperm groups, bearing cones which release pollen to be carried by the wind. Figure 3a shows a similar distribution in IN activity between angiosperm and gymnosperm species. The independent t test failed to reject the hypothesis that there is a significant difference in mean representative nucleation temperature between angiosperms and gymnosperms (t=0.673, p=0.504). This further evidences the variability apparent across the phylogeny of seed-bearing plants. There are properties that are known to have arisen distinctly in separate plant lineages; for example wind pollination is thought to have evolved at least 65 times (Culley et al., 2002; Stephens et al., 2023). However, the apparent ubiquity of these INMs in pollen across all spermatophytes tested implies that these molecules have been around at least since the divergence of angiosperms and gymnosperms, thought to have occurred between 167 and 199 million years ago (Bell et al., 2010).
2.4 Primary growth biome
The biome for each plant species was assigned according to that given in the Plants of the World Online database (Royal Botanic Gardens Kew, 2023). The 50 species tested in this study included representatives from a total of four biomes: seasonally dry tropical, wet tropical, sub-tropical and temperate. The artificial hybrid water lily Nymphaea “Kew's Stowaway Blues” was excluded from the boxplot in Fig. 3b. The independent t test failed to reject the hypothesis that there is a significant difference in mean representative nucleation temperature between sub-tropical and temperate biomes (, p=0.128); wet tropical and dry tropical groups were omitted from the t test as these categories contained data for only three species each. The warmest nucleation temperature points appear in the temperate group (see Fig. 3b). However, highly IN active samples from dry tropical and sub-tropical plants were also observed.
2.5 Pollination season
It had been suggested, based on observations in previous studies of an apparent correlation between cold temperature exposure and high IN activity, that a study of various seasonal and geographic pollen sources should be made (von Blohn et al., 2005). We used a host of online sources to assign pollination seasons, corresponding to the pollination times of plants recorded in their native growth regions, to the 50 species tested. Only two species were classified as pollinating in autumn, so these were grouped with the winter species. The ANOVA test failed to reject that there is a significant difference in mean representative nucleation temperature between Spring, summer and autumn/winter pollination seasons (F=2.10, p=0.135); the year-round group was omitted from the ANOVA test as this category only contained two species. Again, exceptional nucleators were spread across spring, summer and autumn/winter groups. Figure 3c shows no clear difference between spring, summer and autumn/winter distributions. Year-round pollinators nucleated ice poorly. However, we cannot draw a conclusion from this due to the small number of species in this category.
2.6 Primary pollination method
Wind pollination is prevalent amongst gymnosperms, although there are notable exceptions, for instance beetle pollination of Encephalartos spp. (Gregory, 1961). Approximately 88 % of angiosperm species globally are pollinated by animals, most usually insects although also by other invertebrates and vertebrates (Ollerton et al., 2011). As such Fig. 3d closely resembles Fig. 3a, which divides the dataset by spermatophyte subdivision. The interquartile range representing IN activity is similar for wind- and animal-pollinated species. However, the lowest nucleation temperature points are found in the animal-pollinated group. The independent t test failed to reject that there is a significant difference in mean representative nucleation temperature between wind- and animal-pollinated groups (t=1.61, p=0.114). Therefore, we cannot determine a relationship between IN activity and pollination method. It is not the case that pollens of plants adapted for wind pollination possess greater IN activity of those we have tested. While ice nucleation may be involved in some cases in depositing pollen from the atmosphere, these results do not support that there is a positive selection pressure of precipitation on IN activity of pollen in general.
2.7 Elevation range
Figure 3f shows the distribution of representative nucleation temperatures for three plant growth elevation categories. Based on available data, we categorised plant species primarily by the upper limit of growth elevation recorded for the plants in their native regions. Flexible cut-offs of below 1200 m for “low”, between 1200 and 2200 m for “moderate”, and above 2200 m for “high” were used to produce the boxplot. For Fig. 4 we used the numerical values of upper and lower limits of growth elevation for further analysis.
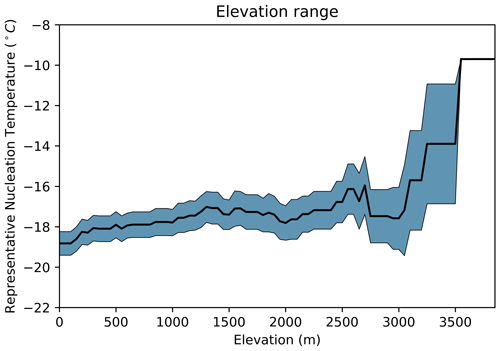
Figure 4Plot of possible growth elevation plotted against representative nucleation temperature (T at nm(T)=100 000 g−1), the values for which are reported in Table S1. The black line represents the mean across species, and the blue shaded area denotes the standard error.
The ANOVA test failed to reject that there is a significant difference in mean representative nucleation temperature between low-, moderate- and high-elevation categories (F=0.789, p=0.460). Therefore, we cannot determine a relationship between IN activity and these categories. From Fig. 3f we can see a slight increase in the median and variance of representative nucleation temperature and elevation. This relationship is shown in Fig. 4, where representative nucleation temperature is displayed against the possible growth elevation of the plant species. As elevation increases the nucleation temperature slightly increases, until around 3000 m where a sharp increase is observed. Although this indicates a relationship between elevation and nucleation temperature, due to the small number of species sampled which are found growing over 3000 m, more data are needed to substantiate this observation.
2.8 Desiccation tolerance
Desiccation tolerance of pollen is a key factor in its longevity and propensity to withstand freezing, so we considered the relationship between desiccation tolerance of pollen grains and IN activity. Categorisation of pollen desiccation tolerance was not possible for all species examined in this study. We categorised the pollen of 39 species as desiccation-tolerant (DT) or desiccation-sensitive (DS) as outlined in the Supplement. The assignments for desiccation tolerance of pollen are included in Table S1.
By visual inspection of the distribution of representative nucleation temperatures for 39 species grouped by desiccation tolerance of their pollen, shown in the boxplot Fig. 3e, both DS pollen and DT pollen exhibit a range of IN activities. Pinus spp. pollen is classed as DT, and yet we see a broad range of IN activities across pine pollen samples tested in this study. Results of the independent t test failed to reject a significant difference in mean representative nucleation temperature between DT and DS pollen (t=0.996, p=0.326).
The pollen of plants within the banana family (Musaceae) has been characterised as DS, and the pollen of Musa rubra, a low-temperature outlier in this study, had a notably high water content on collection. Altogether, this suggests that while no evidence of a direct link between IN activity and desiccation tolerance of pollen grains has been shown here, the water content and biochemistry of the pollen grain, tied to the desiccation sensitivity, clearly have important implications for the INMs released. Therefore, it would be interesting to directly measure the desiccation tolerance and viability of specific pollen samples alongside IN measurements as desiccation sensitivity is a spectrum and is known to vary with stage of development, which may also be an important factor for INM production.
2.9 Fourier transform infrared spectroscopy
Fourier transform infrared (FTIR) spectroscopy was used to gather more information about the soluble components of pollen samples with differing IN activity. Figure 5 shows the normalised FTIR absorption spectra for freeze-dried water-soluble material from Pinus ponderosa, Betula pendula and Sambucus nigra pollen. The spectra shown in Fig. 5 are very similar to FTIR spectra results of pollen INMs reported previously (Dreischmeier et al., 2017; Pummer et al., 2013; Felgitsch et al., 2018) and are consistent with polysaccharide absorbances. Overall, the absorption spectra of soluble material recovered from different pollen samples are remarkably similar, while small variations are apparent in the fingerprint region, as noted by Gute and Abbatt (2020).
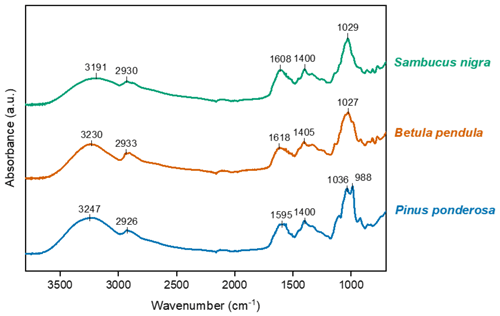
Figure 5Fourier transform infrared absorption spectra for water-soluble material from pollen of three plant species: Pinus ponderosa (low IN activity), Betula pendula (moderate IN activity) and Sambucus nigra (high IN activity). The absorption spectra have been normalised and offset along the y axis for ease of comparison.
The Pinus ponderosa sample, having the lowest ice nucleation temperature of the three, has a notable difference in the 920–1050 cm−1 region when compared with the other two samples. Changes in the 920–1000 cm−1 region have been attributed to differences in the configuration of glycosidic linkages (Nikonenko et al., 2005). Gute and Abbatt (2020) examined absorption spectra for pollen samples from 11 tree species, comparing with IN activity, and attributed the peak between 965 and 1004 cm−1 to the bending mode. Interestingly, they also observed a weak correlation of this signal with mean freezing temperature.
The structure of polysaccharides is characteristically complex, which renders structural analysis more challenging compared with other biomolecules like proteins (Hong et al., 2021). Diversity in the monosaccharides and linkages comprising polysaccharides may partially explain the extent of the variation in IN activity of different samples. It must also be noted that proteins are present in pollen solutions (Pummer et al., 2013; Burkart et al., 2021), and associated absorption signals may be masked by stronger polysaccharide absorbances. Further investigation of the structure and variation of proteinaceous material across species' pollen would be of value to disentangle the role of protein and polysaccharide constituents in the measured IN activity. Further work is needed to better identify the key structures that result in the differences in ice nucleation temperature.
2.10 Other observations and caveats
We found, as observed in previous studies, that the IN activity of pollen suspensions (pre-filtration) was largely consistent with the filtered pollen solutions, clearly indicating the separation of the INMs from the pollen grains and insoluble fragments. Results of time series ice nucleation measurements of Carpinus betulus solutions, where several pollen suspensions were filtered over a set of time intervals, indicated that the INMs separate from the grains almost immediately (see Fig. S3). There was no difference in the IN activity of Carpinus betulus solutions filtered after <1 h vs. 24 h in suspension. However, as it is not known where in the pollen grain the INMs originate, and given the huge structural diversity of pollen grains (e.g. ornamentation and number of pores in the exine), it was decided that all pollen samples would be allowed 1 d in suspension before filtration and ice nucleation measurements, consistent with methods used in previous studies (O'Sullivan et al., 2015; Pummer et al., 2012; Tong et al., 2015; Augustin et al., 2013; Daily et al., 2022).
We note that ice nucleation experiments are highly susceptible to contamination. The samples we have worked with here are of natural origin, and there must be some question regarding contamination from other sources, such as ice-nucleating bacteria. However, we note that two collections of Pinus mugo pollen were made from plants in distinct locations in different years, and the IN activity of the samples was found to be remarkably similar (see Fig. S4), which suggests such contamination is unlikely, though we cannot completely discount the possibility. Pollen solutions prepared from purchased pollen samples have previously been shown to be sterile with no microbial growth detected on agar jelly (Murray et al., 2022). Comparable results for filtered and unfiltered samples, where measured, also support that the IN activity represented is not primarily due to the microflora of the pollen. However, because the majority of pollen samples used in this study were collected from outdoor sources, we cannot entirely discount the possibility that microbiological contamination may have contributed to the IN activity of some samples. As such, microbial analysis of pollen samples might be of value in the future.
Animal-pollinated plants can afford to allocate a lesser proportion of their resources to pollen production than wind-pollinated plants; plant–pollinator relationships ensure a higher likelihood that an individual pollen grain will reach the stigma of another plant and result in fertilisation (Herrera and Pellmyr, 2002). Therefore, it is challenging to collect large quantities of pollen from many animal-pollinated species without extensive harvesting of flowers. In this study, we prioritised nucleation temperature measurements, which allowed a broad comparison of pollen from diverse plant species to be made. Limits on pollen quantities for some species restricted possibilities for further analysis, such as evaluating the quantity and composition of soluble material in each of the pollen solutions.
We have controlled for the mass of pollen added to a volume of water and time in suspension in an attempt to ensure consistency across the pollen samples tested, but this is not a direct measure of the concentration of INMs in each solution. Murray et al. (2022) compared the mass of dried soluble material from solutions of Carpinus betulus and Betula pendula pollen solutions, finding that for both types approximately 0.7 % of the total mass of the samples was soluble material from pollen. However, even the quantity of soluble material is a proxy for the quantity of the INM component, which is estimated to be far smaller (Murray et al., 2022). That the activity of Pinus mugo pollen collected from plants in different locations, in different years, was so similar would seem to indicate the measurements carried out are in some capacity representative of the true pollen activity. Ultimately, these ambiguities highlight, in our view, the importance of work towards identifying the structure of the molecules responsible for the IN activity in pollen.
3.1 Pollen collection
Most pollen samples were collected from the Royal Botanic Gardens, Kew Richmond and Wakehurst sites. Collection methods were adapted according to plant type and pollen quantity. General methods used were as follows.
For cone-bearing plants, the pollen was collected without cutting the plant by covering the male cones with a self-sealing sample bag or glass vial and shaking to release the pollen into the container. For catkin-bearing angiosperms, catkins were collected and placed on paper to allow the release of pollen overnight. For other angiosperms, pollen was collected either by directing the flowers' stamens into a small glass vial and shaking, or for flowers with smaller anthers or lower pollen quantities, stamens were cut and placed on paper and pollen was collected using a small clean spatula to tease the grains free from the anthers. For grass species, the grass culm was cut close to the inflorescence. The cut samples were transported in self-sealing bags and then laid out to dry on paper. Pollen was cleaned by passing it through a 100 µm mesh sieve. Following collection, in all cases, pollen was transferred to a clean glass vial, labelled and kept refrigerated.
Additional samples to supplement direct collections were purchased from Pharmallerga®. Details of pollen collection are included in Table S1.
3.2 Pollen solution preparation
Pollen grains were added to Milli-Q® water at a concentration of 2 wt % or 0.2 wt %, where the quantity of pollen collected would not allow for a sufficient volume of 2 wt % solution for nucleation measurements. Pollen suspensions were refrigerated overnight and then filtered using a 0.2 µm pore size filter into a clean glass vial the following day. Ice nucleation measurements of the solutions were conducted following filtration.
3.3 Ice nucleation experiments
To measure the IN activity of pollen solutions, arrays of 1 µL droplets were cooled on a purpose-built cold stage, similar to that described by Whale (2024), and the nucleation temperature of each droplet was recorded. The instrument used for these experiments consists of a TEC1-12704 Peltier thermoelectric cooler, connected to a recirculating chiller, driven by a Meerstetter TEC-1091-PT100 Precision Peltier Controller. A 40×40 mm aluminium plate, onto which a glass slide is placed, is bonded to the Peltier using Arctic Cooling MX-4 thermal compound. Two independent Netsushin PT100 platinum resistance thermometers (NR-141-100S-2-1.0-10-2000PLi-A-3) fixed within the cold stage, read by a PicoTech PT-104 Platinum Resistance Data Logger, continuously monitor the stage's temperature to within ±0.1 °C.
Using a Sartorius Picus® electronic micropipette, approximately 50×1 µL droplets of pollen solution were transferred onto a 22 mm diameter Hampton Research HR3-231 siliconised glass slide, placed on the aluminium plate of the cold stage. The droplets were enclosed by a screw lid top with a window, through which a video camera recorded footage of the droplets as they were cooled. A continuous dry-air flow was passed over the droplets to control the relative humidity, preventing frost growth affecting nucleation temperatures. The cooling (stage temperature and rate of change of temperature) was controlled via a custom LabVIEW program.
For these experiments, the droplet arrays were cooled at a rate of 2 °C min−1. This cooling rate was chosen for convenience and is similar to that used in many previous studies (e.g. Whale, 2024). The onset of freezing was detected by an increase droplet opacity. The recorded temperature corresponding to the first frame where freezing became visible was taken as the nucleation temperature of the droplet. Two sets of approximately 50×1 µL droplet arrays were cooled to freezing for each pollen sample, to enable comparison of IN activity by fraction of droplets frozen against temperature.
3.4 Analysis of droplet nucleation temperatures
The fraction of droplets frozen, fice(T), is given by
where n(T) is the number of frozen droplets and N is the total number of droplets.
The cumulative number of nucleation sites per gram pollen, nm(T), is calculated from the fraction of droplets frozen, fice(T), and the mass of pollen in each droplet of the pollen suspension, M, in grams by following equation:
So, where the concentration of the pollen suspension is 2 wt % (0.02 g mL−1) and the droplet volume is 0.001 mL, the mass of pollen contributing to each droplet M= 0.02 mg. nm(T) can therefore be found, in g−1, by
For further analysis, a single temperature value representing the droplet nucleation temperatures of each pollen solution was required. The mean freezing temperature, T50, is commonly used for this. In this case, due to very limited quantities of some species' pollen, 10 of the 51 solutions tested had to be prepared at the lower concentration of 0.2 wt %. As T50 does not account for concentrations, which vary for the solutions tested in our experiments, here we used a representative nucleation temperature, Trep, which is the temperature value corresponding to nm(T)=100 000 g−1. To find Trep for a given solution, we first calculated the values for the number of nucleation sites per gram pollen, nm(T), for each recorded droplet freezing event and then calculated the value of Trep by linear interpolation from the two nearest known points.
To calculate confidence intervals for the data produced, we have calculated the Kaplan–Meier estimator for our droplet freezing experiment using
where nu is the number of unfrozen droplets at temperature T, and dT is the number of droplets that freeze at temperature T. Note that fice(T) is equal to . The asymptotic variance of may be calculated as
where the sums are calculated between T and the first value of T at which a freezing event occurs. The confidence intervals are then given by , where is the inverse of the standard normal cumulative distribution, which takes values of 1.96 for the 95 % confidence used in this study. Confidence intervals for nm(T) were calculated by applying Eq. (2) to the absolute values of the upper and lower confidence intervals for fice(T). Calculations were performed using Stata 17 (StataCorp, 2021).
3.5 Statistical analysis
Representative nucleation temperatures, Trep (T at nm(T)=100 000 g−1), were used to evaluate the influence of various plant features, our explanatory variables, on IN activity. Independent t tests and ANOVA tests were used to evaluate whether the groups (e.g. animal-pollinated, wind-pollinated) within the explanatory variables (e.g. pollination method) showed significant difference in representative nucleation temperatures. Independent t tests were used for the variables with two groups, and ANOVA tests were used for the variables with more than two groups. These tests were selected based on the distribution of data points and the similarity in variance between groups. The joint dependency of explanatory variables on Trep was not measured due to the high dependency between explanatory variables. All tests were performed in Python using the scipy.stats package.
3.6 Fourier transform infrared spectroscopy
Concentrated (20 wt %) pollen suspensions of Pinus ponderosa, Betula pendula and Sambucus nigra pollen, purchased from Pharmallerga®, were prepared by adding 1 g pollen to 4 mL Milli-Q® water. After 24 h in the refrigerator, the suspensions were filtered through 0.2 µm pore size syringe filters. Following filtration, each pollen solution was freeze-dried. The infrared absorption spectra of the dried soluble material from the solutions were recorded using the Agilent Technologies Cary 630 FTIR Spectrometer.
To the best of our knowledge, this is the most comprehensive survey of ice nucleation by pollen conducted to date. Multiple species, spread across the phylogeny of seed-bearing plants and across ecological niches, can produce INMs that nucleate ice at warm temperatures, above −10 °C. Previously, only one measurement had found pollen INMs nucleating at such warm temperatures: that of Gute and Abbatt (2020) on Alnus incana pollen. However, our results suggest that many species' pollen may produce such active ice nucleators.
Almost all pollen types tested produced INMs capable of inducing ice nucleation at moderate temperatures between around −16 and −22 °C, as shown by the high-density peak in the centre of Fig. 1c. We found no association between phylogenetic proximity and IN activity. Exceptional pollen INMs are distributed across lineages. We found some weak trends with environmental factors. Temperate pollens tend to nucleate ice better than tropical ones. Wind-dispersed pollens tend to nucleate ice better than animal-dispersed ones. Pollen from high-elevation plants tends to nucleate ice better than that from low-elevation plants. We found none of these trends to be statistically significant, however.
The evidence we have collected suggests that the INMs are ubiquitous in plant pollen. It seems to us likely that the IN activity of pollen may be conserved incidentally from an ancient ancestor. It is hard to conceive of a reason for INMs produced by the pollen of a tropical, entomophilous plant to nucleate ice. Such a pollen would never be exposed to freezing temperatures. Yet, pollen from Hymenocallis littoralis produced notably efficient ice nucleators. Indeed, exceptional ice nucleators are found in pollens from plants occupying all niches investigated.
Classical nucleation theory (CNT) suggests that larger nucleator surfaces are capable of inducing ice nucleation at higher temperatures than smaller ones. Experimentally determined sizes of INMs are found to agree with critical ice cluster size calculated according to CNT (Pummer et al., 2015). This implies that molecules of ∼300 kDa must form to account for the observation of ice nucleation above −10 °C by a substantial subset of the pollen solutions we have tested (Pummer et al., 2015). We suggest that some plants have evolved the ability to produce either aggregates or larger versions of the INMs in order to nucleate ice well for some biological function. It is also possible that larger molecules serve some other purpose and that IN activity is entirely incidental. As some pollen types which have no conceivable use for effective ice nucleators nevertheless possess them, it seems quite likely that these large molecules or molecular aggregates serve some other biological function or that such size imposes a negligible evolutionary disadvantage.
We think that the most likely explanation for our results is that a macromolecule associated with pollen has an intrinsic, incidental ability to nucleate ice effectively. We hypothesise that some plant species, notably those which live at high elevations, have adapted this macromolecule to nucleate ice particularly effectively for some biological purpose.
High-temperature ice nucleators can have a disproportionate effect on cloud properties as secondary ice production mechanisms are more prevalent at warm temperatures above about −10 °C (Korolev and Leisner, 2020). We propose that pollen should now be considered as a possible source of highly active INPs in the atmosphere. Previous studies have quoted an estimate of global pollen emissions to the atmosphere of 84.5 Tg yr−1, based on a generalisation of pollen grains released by a single species, Zea mays (Jacobson and Streets, 2009). Using an aerosol–climate model incorporating a simplified emission parameterisation based on this estimate, Hoose et al. (2010a, b) found pollen, considered together with other primary biological aerosol particles, has little impact on atmospheric ice nucleation on a global scale. It has been suggested that regional impact may be far greater (Williams and Després, 2017). More recently, studies have considered the potential for sub-pollen particles (SPPs), generated by pollen rupture under atmospheric conditions (Steiner and Solmon, 2018; Subba et al., 2023), to act as persisting carriers for pollen INMs compounding their atmospheric relevance (Burkart et al., 2021). Due to their size, SPPs can accumulate in the atmosphere to a greater extent than whole pollen grains; simulations estimate SPP concentrations are 4–6 orders of magnitude higher than pollen grain concentrations (Werchner et al., 2022). Given that SPPs can significantly influence ice particle number density, impacting mixed-phase cloud composition and precipitation, when considered as highly efficient ice nuclei (Werchner et al., 2022), the possibility that a consequential proportion of pollen material holds highly active ice nucleators must now be considered.
Supporting data are available in the Supplement and online at https://wrap.warwick.ac.uk/186384 (Kinney et al., 2024).
The supplement related to this article is available online at: https://doi.org/10.5194/bg-21-3201-2024-supplement.
NLHK, CAH, DB and TFW contributed to pollen sample collection. NLHK conducted ice nucleation experiments and performed data analysis. CAH advised on and conducted statistical analysis. MIG, DB and TFW supervised the project. NLHK and TFW wrote the original manuscript draft. All authors provided critical feedback on the manuscript.
Thomas F. Whale, Matthew I. Gibson and Nina L. H. Kinney are named inventors on a patent application relating to this work (reference GB 2115794.6).
Publisher’s note: Copernicus Publications remains neutral with regard to jurisdictional claims made in the text, published maps, institutional affiliations, or any other geographical representation in this paper. While Copernicus Publications makes every effort to include appropriate place names, the final responsibility lies with the authors.
Nina L. H. Kinney received funding from the Natural Environment Research Council and the CENTA Doctoral Training Partnership in the form of a PhD studentship (grant no. NE/S007350/1). Thomas F. Whale received funding from the Leverhulme Trust and the University of Warwick in the form of an early-career fellowship (grant no. ECF-2018-127). Matthew I. Gibson received funding from the European Research Council (ERC), under the European Union's Horizon 2020 Research and Innovation programme (grant no. 866056), and the Royal Society in the form of an industry fellowship (grant no. 191037) in partnership with Cytiva. The Royal Botanic Gardens, Kew, receive grant-in-aid from the UK government (Department for Environment, Food and Rural Affairs).
This paper was edited by Paul Stoy and reviewed by Hinrich Grothe and Cindy Morris.
Ambroise, V., Legay, S., Guerriero, G., Hausman, J. F., Cuypers, A., and Sergeant, K.: The Roots of Plant Frost Hardiness and Tolerance, Plant Cell Physiol., 61, 3–20, https://doi.org/10.1093/PCP/PCZ196, 2020.
Augustin, S., Wex, H., Niedermeier, D., Pummer, B., Grothe, H., Hartmann, S., Tomsche, L., Clauss, T., Voigtländer, J., Ignatius, K., and Stratmann, F.: Immersion freezing of birch pollen washing water, Atmos. Chem. Phys., 13, 10989–11003, https://doi.org/10.5194/acp-13-10989-2013, 2013.
Bell, C. D., Soltis, D. E., and Soltis, P. S.: The age and diversification of the angiosperms re-revisited, Am. J. Bot., 97, 1296–1303, https://doi.org/10.3732/AJB.0900346, 2010.
Bessey, C. E.: Remarkable fall of pine pollen, Am. Nat., 17, 658–658, https://doi.org/10.1086/273405, 1883.
Brush, R. A., Griffith, M., and Mlynarz, A.: Characterization and Quantification of lntrinsic Ice Nucleators in Winter Rye (Secale cereale) Leaves', Plant Physiol., 104, 725–735, 1994.
Burkart, J., Gratzl, J., Seifried, T. M., Bieber, P., and Grothe, H.: Isolation of subpollen particles (SPPs) of birch: SPPs are potential carriers of ice nucleating macromolecules, Biogeosciences, 18, 5751–5765, https://doi.org/10.5194/bg-18-5751-2021, 2021.
Cornwell, G. C., McCluskey, C. S., Hill, T. C. J., Levin, E. T., Rothfuss, N. E., Tai, S.-L., Petters, M. D., DeMott, P. J., Kreidenweis, S., Prather, K. A., and Burrows, S. M.: Bioaerosols are the dominant source of warm-temperature immersion-mode INPs and drive uncertainties in INP predictability, Sci. Adv., 9, eadg3715, https://doi.org/10.1126/SCIADV.ADG3715, 2023.
Culley, T. M., Weller, S. G., and Sakai, A. K.: The evolution of wind pollination in angiosperms, Trends Ecol. Evol., 17, 361–369, https://doi.org/10.1016/S0169-5347(02)02540-5, 2002.
Daily, M. I., Tarn, M. D., Whale, T. F., and Murray, B. J.: An evaluation of the heat test for the ice-nucleating ability of minerals and biological material, Atmos. Meas. Tech., 15, 2635–2665, https://doi.org/10.5194/amt-15-2635-2022, 2022.
DeMott, P. J. and Prenni, A. J.: New Directions: Need for defining the numbers and sources of biological aerosols acting as ice nuclei, Atmos. Environ., 44, 1944–1945, https://doi.org/10.1016/J.ATMOSENV.2010.02.032, 2010.
Després, V. R., Alex Huffman, J., Burrows, S. M., Hoose, C., Safatov, A. S., Buryak, G., Fröhlich-Nowoisky, J., Elbert, W., Andreae, M. O., Pöschl, U., and Jaenicke, R.: Primary biological aerosol particles in the atmosphere: a review, Stock. Uni Press, 64, https://doi.org/10.3402/TELLUSB.V64I0.15598, 2012.
Diehl, K., Matthias-Maser, S., Jaenicke, R., and Mitra, S. K.: The ice nucleating ability of pollen: Part II. Laboratory studies in immersion and contact freezing modes, Atmos. Res., 61, 125–133, https://doi.org/10.1016/S0169-8095(01)00132-6, 2002.
Dreischmeier, K., Budke, C., Wiehemeier, L., Kottke, T., and Koop, T.: Boreal pollen contain ice-nucleating as well as ice-binding “antifreeze” polysaccharides, Sci. Rep., 7, 41890, https://doi.org/10.1038/srep41890, 2017.
Duan, P., Hu, W., Wu, Z., Bi, K., Zhu, J., and Fu, P.: Ice nucleation activity of airborne pollen: A short review of results from laboratory experiments, Atmos. Res., 285, 106659, https://doi.org/10.1016/j.atmosres.2023.106659, 2023.
Eufemio, R. J., de Almeida Ribeiro, I., Sformo, T. L., Laursen, G. A., Molinero, V., Fröhlich-Nowoisky, J., Bonn, M., and Meister, K.: Lichen species across Alaska produce highly active and stable ice nucleators, Biogeosciences, 20, 2805–2812, https://doi.org/10.5194/bg-20-2805-2023, 2023.
Felgitsch, L., Baloh, P., Burkart, J., Mayr, M., Momken, M. E., Seifried, T. M., Winkler, P., Schmale III, D. G., and Grothe, H.: Birch leaves and branches as a source of ice-nucleating macromolecules, Atmos. Chem. Phys., 18, 16063–16079, https://doi.org/10.5194/acp-18-16063-2018, 2018.
Friedman, J. and Barrett, S. C. H.: Wind of change: new insights on the ecology and evolution of pollination and mating in wind-pollinated plants, Ann. Bot., 103, 1515–1527, https://doi.org/10.1093/AOB/MCP035, 2009.
Gao, Y., Bissoyi, A., Kinney, N. L. H., Whale, T. F., Guo, Q., and Gibson, M. I.: Proline-conditioning and chemically-programmed ice nucleation protects spheroids during cryopreservation, Chem. Commun., 59, 9086–9089, https://doi.org/10.1039/D3CC02252H, 2023.
Gregory, P. H.: The microbiology of the atmosphere, Leonard Hill Books, https://doi.org/10.5962/bhl.title.7291, 1961.
Gross, D. C., Proebsting, E. L., and Maccrindle-Zimmerman, H.: Development, Distribution, and Characteristics of Intrinsic, Nonbacterial Ice Nuclei in Prunus Wood, Plant Physiol., 88, 915–922, https://doi.org/10.1104/PP.88.3.915, 1988.
Grote, M., Valenta, R., and Reichelt, R.: Abortive pollen germination: a mechanism of allergen release in birch, alder, and hazel revealed by immunogold electron microscopy, J. Allergy Clin. Immun., 111, 1017–1023, https://doi.org/10.1067/MAI.2003.1452, 2003.
Gute, E. and Abbatt, J. P. D.: Ice nucleating behavior of different tree pollen in the immersion mode, Atmos. Environ., 231, 117488, https://doi.org/10.1016/j.atmosenv.2020.117488, 2020.
Gute, E., David, R. O., Kanji, Z. A., and Abbatt, J. P. D.: Ice Nucleation Ability of Tree Pollen Altered by Atmospheric Processing, ACS Earth Sp. Chem., 4, 2312–2319, https://doi.org/10.1021/acsearthspacechem.0c00218, 2020.
Hallett, J. and Mossop, S. C.: Production of secondary ice particles during the riming process, Nature, 249, 26–28, https://doi.org/10.1038/249026a0, 1974.
Herrera, C. M. and Pellmyr, O.: Plant Animal Interactions: An Evolutionary Approach, John Wiley & Sons, ISBN 978-0-632-05267-7, 2002.
Hong, T., Yin, J. Y., Nie, S. P., and Xie, M. Y.: Applications of infrared spectroscopy in polysaccharide structural analysis: Progress, challenge and perspective, Food Chem. X, 12, 100168, https://doi.org/10.1016/J.FOCHX.2021.100168, 2021.
Hoose, C., Kristjánsson, J. E., Chen, J. P., and Hazra, A.: A Classical-Theory-Based Parameterization of Heterogeneous Ice Nucleation by Mineral Dust, Soot, and Biological Particles in a Global Climate Model, J. Atmos. Sci., 67, 2483–2503, https://doi.org/10.1175/2010JAS3425.1, 2010a.
Hoose, C., Kristjánsson, J. E., and Burrows, S. M.: How important is biological ice nucleation in clouds on a global scale?, Environ. Res. Lett., 5, 024009, https://doi.org/10.1088/1748-9326/5/2/024009, 2010b.
Huang, S., Hu, W., Chen, J., Wu, Z., Zhang, D., and Fu, P.: Overview of biological ice nucleating particles in the atmosphere, Environ. Int., 146, 106197, https://doi.org/10.1016/j.envint.2020.106197, 2021.
Hummel, M., Hoose, C., Pummer, B., Schaupp, C., Fröhlich-Nowoisky, J., and Möhler, O.: Simulating the influence of primary biological aerosol particles on clouds by heterogeneous ice nucleation, Atmos. Chem. Phys., 18, 15437–15450, https://doi.org/10.5194/acp-18-15437-2018, 2018.
Jacobson, M. Z. and Streets, D. G.: Influence of future anthropogenic emissions on climate, natural emissions, and air quality, J. Geophys. Res.-Atmos., 114, 8118, https://doi.org/10.1029/2008JD011476, 2009.
Kanji, Z. A., Ladino, L. A., Wex, H., Boose, Y., Burkert-Kohn, M., Cziczo, D. J., and Krämer, M.: Overview of Ice Nucleating Particles, Meteorol. Monogr., 58, 1.1–1.33, https://doi.org/10.1175/AMSMONOGRAPHS-D-16-0006.1, 2017.
Kieft, T. L.: Ice nucleation activity in lichens, Appl. Environ. Microbiol., 54, 1678–1681, https://doi.org/10.1128/AEM.54.7.1678-1681.1988, 1988.
Kinney, N. L. H., Hepburn, C. A., Gibson, M. I., Ballesteros, D., and Whale, T. F.: Data for High interspecific variability in ice nucleation activity suggests pollen ice nucleators are incidental, University of Warwick [data set], https://wrap.warwick.ac.uk/186384 (last access: 3 July 2024), 2024.
Knopf, D. A. and Alpert, P. A.: Atmospheric ice nucleation, Nat. Rev. Phys., 54, 203–217, https://doi.org/10.1038/s42254-023-00570-7, 2023.
Korolev, A. and Leisner, T.: Review of experimental studies of secondary ice production, Atmos. Chem. Phys., 20, 11767–11797, https://doi.org/10.5194/acp-20-11767-2020, 2020.
Kunert, A. T., Pöhlker, M. L., Tang, K., Krevert, C. S., Wieder, C., Speth, K. R., Hanson, L. E., Morris, C. E., Schmale III, D. G., Pöschl, U., and Fröhlich-Nowoisky, J.: Macromolecular fungal ice nuclei in Fusarium: effects of physical and chemical processing, Biogeosciences, 16, 4647–4659, https://doi.org/10.5194/bg-16-4647-2019, 2019.
Lindow, S. E., Arny, D. C., and Upper, C. D.: Bacterial Ice Nucleation: A Factor in Frost Injury to Plants, Plant Physiol., 70, 1084–1089, https://doi.org/10.1104/PP.70.4.1084, 1982.
Lukas, M., Schwidetzky, R., Eufemio, R. J., Bonn, M., and Meister, K.: Toward Understanding Bacterial Ice Nucleation, J. Phys. Chem. B, 126, 1861–1867, https://doi.org/10.1021/acs.jpcb.1c09342, 2022.
Lundheim, R.: Physiological and ecological significance of biological ice nucleators, Philos. T. Roy. Soc. B, 357, 937–943, https://doi.org/10.1098/RSTB.2002.1082, 2002.
Maki, L. R., Galyan, E. L., Chang-Chien, M.-M., and Caldwell, D. R.: Ice nucleation induced by pseudomonas syringae, Appl. Microbiol., 28, 456–459, https://doi.org/10.1128/AM.28.3.456-459.1974, 1974.
Morris, C. E., Georgakopoulos, D. G., and Sands, D. C.: Ice nucleation active bacteria and their potential role in precipitation, J. Phys. IV, 121, 87–103, https://doi.org/10.1051/JP4:2004121004, 2004.
Murray, B. J., O'sullivan, D., Atkinson, J. D., and Webb, M. E.: Ice nucleation by particles immersed in supercooled cloud droplets, Chem. Soc. Rev., 41, 6519–6554, https://doi.org/10.1039/c2cs35200a, 2012.
Murray, B. J., Carslaw, K. S., and Field, P. R.: Opinion: Cloud-phase climate feedback and the importance of ice-nucleating particles, Atmos. Chem. Phys., 21, 665–679, https://doi.org/10.5194/acp-21-665-2021, 2021.
Murray, K. A., Kinney, N. L. H., Griffiths, C. A., Hasan, M., Gibson, M. I., and Whale, T. F.: Pollen derived macromolecules serve as a new class of ice-nucleating cryoprotectants, Sci. Rep., 12, 12295, https://doi.org/10.1038/s41598-022-15545-4, 2022.
Murray, K. A., Gao, Y., Griffiths, C. A., Kinney, N. L. H., Guo, Q., Gibson, M. I., and Whale, T. F.: Chemically Induced Extracellular Ice Nucleation Reduces Intracellular Ice Formation Enabling 2D and 3D Cellular Cryopreservation, JACS Au, 3, 1314–1320, https://doi.org/10.1021/JACSAU.3C00056, 2023.
Nikonenko, N. A., Buslov, D. K., Sushko, N. I., and Zhbankov, R. G.: Spectroscopic manifestation of stretching vibrations of glycosidic linkage in polysaccharides, J. Mol. Struct., 752, 20–24, https://doi.org/10.1016/J.MOLSTRUC.2005.05.015, 2005.
Ollerton, J., Winfree, R., and Tarrant, S.: How many flowering plants are pollinated by animals?, Oikos, 120, 321–326, https://doi.org/10.1111/J.1600-0706.2010.18644.X, 2011.
O'Sullivan, D., Murray, B. J., Ross, J. F., Whale, T. F., Price, H. C., Atkinson, J. D., Umo, N. S., and Webb, M. E.: The relevance of nanoscale biological fragments for ice nucleation in clouds, Sci. Rep., 5, 8082, https://doi.org/10.1038/srep08082, 2015.
Potter, L. D. and Rowley, J.: Pollen Rain and Vegetation, San Augustin Plains, New Mexico, Gazette, 122, 1–25, 1960.
Pummer, B. G., Bauer, H., Bernardi, J., Bleicher, S., and Grothe, H.: Suspendable macromolecules are responsible for ice nucleation activity of birch and conifer pollen, Atmos. Chem. Phys., 12, 2541–2550, https://doi.org/10.5194/acp-12-2541-2012, 2012.
Pummer, B. G., Bauer, H., Bernardi, J., Chazallon, B., Facq, S., Lendl, B., Whitmore, K., and Grothe, H.: Chemistry and morphology of dried-up pollen suspension residues, J. Raman Spectrosc., 44, 1654–1658, https://doi.org/10.1002/jrs.4395, 2013.
Pummer, B. G., Budke, C., Augustin-Bauditz, S., Niedermeier, D., Felgitsch, L., Kampf, C. J., Huber, R. G., Liedl, K. R., Loerting, T., Moschen, T., Schauperl, M., Tollinger, M., Morris, C. E., Wex, H., Grothe, H., Pöschl, U., Koop, T., and Fröhlich-Nowoisky, J.: Ice nucleation by water-soluble macromolecules, Atmos. Chem. Phys., 15, 4077–4091, https://doi.org/10.5194/acp-15-4077-2015, 2015.
Royal Botanic Gardens Kew: Plants of the World Online, http://www.plantsoftheworldonline.org/ (last access: 1 November 2023), 2023.
StataCorp: Stata Statistical Software: Release 17, College Station, TX, StataCorp LLC., 2021.
Steiner, A. L. and Solmon, F.: Pollen Rupture and Its Impact on Precipitation in Clean Continental Conditions, Geophys. Res. Lett., 45, 7156–7164, https://doi.org/10.1029/2018GL077692, 2018.
Stephens, R. E., Gallagher, R. V., Dun, L., Cornwell, W., and Sauquet, H.: Insect pollination for most of angiosperm evolutionary history, New Phytol., 240, 880–891, https://doi.org/10.1111/NPH.18993, 2023.
Subba, T., Zhang, Y., and Steiner, A. L.: Simulating the Transport and Rupture of Pollen in the Atmosphere, J. Adv. Model. Earth Sy., 15, e2022MS003329, https://doi.org/10.1029/2022MS003329, 2023.
Szyrmer, W. and Zawadzki, I.: Biogenic and Anthropogenic Sources of Ice-Forming Nuclei: A Review, B. Am. Meteor. Soc., 78, 209–228, https://doi.org/10.1175/1520-0477(1997)078<0209:BAASOI>2.0.CO;2, 1997.
Taylor, P. E., Flagan, R. C., Miguel, A. G., Valenta, R., and Glovsky, M. M.: Birch pollen rupture and the release of aerosols of respirable allergens, Clin. Exp. Allergy, 34, 1591–1596, https://doi.org/10.1111/j.1365-2222.2004.02078.x, 2004.
Tong, H.-J., Ouyang, B., Nikolovski, N., Lienhard, D. M., Pope, F. D., and Kalberer, M.: A new electrodynamic balance (EDB) design for low-temperature studies: application to immersion freezing of pollen extract bioaerosols, Atmos. Meas. Tech., 8, 1183–1195, https://doi.org/10.5194/amt-8-1183-2015, 2015.
von Blohn, N., Mitra, S. K., Diehl, K., and Borrmann, S.: The ice nucleating ability of pollen: Part III: New laboratory studies in immersion and contact freezing modes including more pollen types, Atmos. Res., 78, 182–189, https://doi.org/10.1016/j.atmosres.2005.03.008, 2005.
Wang, X. Q. and Ran, J. H.: Evolution and biogeography of gymnosperms, Mol. Phylogenet. Evol., 75, 24–40, https://doi.org/10.1016/J.YMPEV.2014.02.005, 2014.
Werchner, S., Gute, E., Hoose, C., Kottmeier, C., Pauling, A., Vogel, H., and Vogel, B.: When Do Subpollen Particles Become Relevant for Ice Nucleation Processes in Clouds?, J. Geophys. Res.-Atmos., 127, e2021JD036340, https://doi.org/10.1029/2021JD036340, 2022.
Whale, T. F.: Quantification of the Ice Nucleation Activity of Ice-Binding Proteins Using a Microliter Droplet Freezing Experiment, Methods Mol. Biol., 2730, 121–134, https://doi.org/10.1007/978-1-0716-3503-2_9, 2024.
Wieland, F., Bothen, N., Schwidetzky, R., Seifried, T. M., Bieber, P., Pöschl, U., Meister, K., Bonn, M., Fröhlich-Nowoisky, J., and Grothe, H.: Aggregation of ice-nucleating macromolecules from Betula pendula pollen determines ice nucleation efficiency, EGUsphere [preprint], https://doi.org/10.5194/egusphere-2024-752, 2024.
Williams, C. G.: Forest tree pollen dispersal via the water cycle, Am. J. Bot., 100, 1184–1190, https://doi.org/10.3732/AJB.1300085, 2013.
Williams, C. G. and Després, V.: Northern Hemisphere forests at temperate and boreal latitudes are substantial pollen contributors to atmospheric bioaerosols, Forest Ecol. Manage., 401, 187–191, https://doi.org/10.1016/J.FORECO.2017.06.040, 2017.
Wozniak, M. C., Solmon, F., and Steiner, A. L.: Pollen Rupture and Its Impact on Precipitation in Clean Continental Conditions, Geophys. Res. Lett., 45, 7156–7164, https://doi.org/10.1029/2018GL077692, 2018.
Zachariassen, K. E. and Hammel, H. T.: Nucleating agents in the haemolymph of insects tolerant to freezing, Nature, 262, 285–287, https://doi.org/10.1038/262285a0, 1976.
Zachariassen, K. E. and Kristiansen, E.: Ice Nucleation and Antinucleation in Nature, Cryobiology, 41, 257–279, https://doi.org/10.1006/cryo.2000.2289, 2000.