the Creative Commons Attribution 4.0 License.
the Creative Commons Attribution 4.0 License.
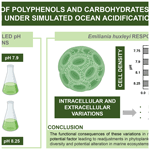
Variations of polyphenols and carbohydrates of Emiliania huxleyi grown under simulated ocean acidification conditions
Milagros Rico
Paula Santiago-Díaz
Guillermo Samperio-Ramos
Melchor González-Dávila
Juana Magdalena Santana-Casiano
Cultures of the coccolithophore Emiliania huxleyi were grown under four different CO2-controlled pH conditions (7.75, 7.90, 8.10, and 8.25) to explore variations in extra- and intracellular polyphenols and carbohydrates in response to different ocean acidification scenarios. Acidification did not significantly affect final cell densities and carbohydrate contents. Intra- and extracellular phenolic compounds were identified and quantified by reverse-phase high-performance liquid chromatography (RP-HPLC), with the highest concentrations of total exuded phenolics at a pH of 8.25 (43 ± 3 nM) and 7.75 (18.0 ± 0.9 nM). Accumulation of intracellular phenolic compounds was observed in cells with decreasing pH, reaching the maximum level (9.24 ± 0.19 attomole per cell) at the lowest pH (7.75). The phenolic profiles presented significant changes in exuded epicatechin and protocatechuic acid (p < 0.05 and 0.01, respectively) and intracellular vanillic acid (p < 0.001), which play an essential role in the availability of trace metals. A significant increase in chlorophyll a content was observed in cells grown at the most acidic pH (p < 0.01), which also showed significantly higher radical inhibition activity (p < 0.01). The nature and concentration of these organic compounds present in the culture medium may influence trace metal bioavailability, affecting the biogeochemical cycling of carbon and microbial functional diversity.
- Article
(721 KB) - Full-text XML
- BibTeX
- EndNote
Microalgae play a key role in marine ecosystems, forming the basis of the marine food chain as they are responsible for almost half of the total primary production (Usher et al., 2014; Dedman et al., 2023). They constitute a potential feedstock because of their valuable metabolites such as pigments, vitamins, proteins, carbohydrates, and lipids with valued fatty acids. Marine phytoplankton, primarily diatoms and coccolithophorids groups, drive the oceanic carbon cycle by sequestering inorganic carbon from the atmosphere during photosynthesis (Marinov et al., 2010). Coccolithophores are the most abundant calcifying phytoplankton and form gigantic blooms throughout the oceans, especially in mid-latitudes. The coccolithophore Emiliania huxleyi (E. huxleyi) is the main contributor to calcareous sea sediments, making it particularly sensitive to ocean acidification and playing a crucial ecological role (Arundhathy et al., 2021; Westbroek, 1992). When exposed to elevated CO2 and low pH, the majority of coccolithophores reduce their growth rate and level of calcification, resulting in thinner coccolithphores (Kholssi et al., 2023; Mackey et al., 2015; Meier et al., 2014). However, calcification is promoted in certain species and/or strains within the same species under enhanced CO2 (Shi et al., 2009).
Global environmental changes, in particular those related to increasing temperature and decreasing pH, profoundly affect ocean ecosystems at many levels (Berge et al., 2010; Dedman et al., 2023; Kholssi et al., 2023; Lu et al., 2013). The absorption of anthropogenic CO2 into seawater alters the natural chemical equilibrium of CO2–carbonate system, resulting in a decrease of the chemical bases in seawater, increasing protons (H+) and lowering its pH in a process termed “ocean acidification”, with adverse consequences for marine ecosystems and human societies (Bates et al., 2014; Jiang et al., 2023; Lida et al., 2021). In fact, pre-industrial seawater pH (8.25) has already dropped to 8.10 and is expected to reach a pH of 7.85 in this century (Jacobson, 2005).
Different algal species show different optimal pH ranges for maximum growth (Hoppe et al., 2011; Kholssi et al., 2023). Changes in environmental pH could have consequences on the competitiveness of both sensitive and tolerant microalgae in mixed phytoplankton communities, modifying their structure, composition, and distribution, which are crucial in mitigating global environmental change by fixing and transporting carbon from the upper to the deep ocean in the major global carbon sink (Eltanahy and Torky, 2021; Kholssi et al., 2023; Marinov et al., 2010). Vasconcelos et al. (2002) found that exudates from Phaeodactylum tricornutum (P. tricornutum) diatoms caused a toxic effect on E. huxleyi, while those from Enteromorpha spp. caused an increase in final cell yield, concluding that specific exudates produced by the bloom of one algal species may favour or inhibit the local growth of other species. Such changes could also affect the species at a higher trophic level, resulting in a potential shift in biodiversity (Jin and Kirk, 2018). Spisla et al. (2021) reported that extreme CO2 events modify the composition of particulate organic matter in the ocean, which leads to a substantial reorganization of the planktonic community, affecting multiple trophic levels from phytoplankton to primary and secondary consumers (Nelson et al., 2020; Trombetta et al., 2019). Barcelos e Ramos et al. (2022) showed that coexistence with other microorganisms modifies the response of E. huxleyi subjected to high CO2 concentration, markedly decreasing its growth rate and cellular inorganic carbon and increasing its organic carbon in the presence of the Bacteria Idiomarina abyssalis and Brachybacterium sp.
Changes in phytoplankton communities due to variation in seawater acidity alter the composition of the organic ligands that these communities release into the surrounding environment (Samperio-Ramos et al., 2017). These ligands are crucial for acquiring micronutrients, sequestering toxic metals, and establishing electrochemical gradients that result in changes in the speciation, bioavailability, and toxicity of trace metals (Harmesa et al., 2022; Santana-Casiano et al., 2014). Iron is an essential micronutrient for phytoplankton involved in fundamental cellular processes (Raven et al., 1999; Hogle et al., 2014), controlling productivity, species composition, and trophic structure of microbial communities over large regions of the ocean (González et al., 2019; Hunter and Boyd, 2007). Complexation with organic compounds is one of the mechanisms for maintaining dissolved iron concentrations above its inorganic solubility (Hunter and Boyd, 2007; Shaked et al., 2020). A decrease in seawater pH from 8.1 to 7.4 will increase Fe(III) solubility by approximately 40 %, which could have a large impact on biogeochemical cycles (Morel and Price, 2003; Millero et al., 2009). In addition, some research work has shown that the residence time of the reduced form of essential trace metals increases as their oxidation rate decreases under acidifying conditions (Pérez-Almeida et al., 2022; Santana-Casiano et al., 2014).
Carbohydrates are one of the major components of the dissolved organic carbon (DOC) pool in marine environments, representing 3 %–50 % of dissolved and colloidal organic matter (Giljan et al., 2023; Hassler et al., 2011; Wang et al., 2006). Phenolic compounds are secondary metabolites synthesized as a defence mechanism of organisms exposed to abiotic stresses (salinity, metal toxicity, heat, acidification, cold, extreme light, nutrient deficiency, UV radiation) (López et al., 2015; Parvin et al., 2022). Their antioxidant nature enhances tolerance of organisms to adverse climatic conditions that induce an increase in reactive oxygen species (ROSs) (Gauthier et al., 2020; Vázquez et al., 2022). Both types of compounds can influence the iron chemistry and bioavailability (Pérez-Almeida et al., 2022; Santana-Casiano et al., 2014). Catechin, sinapic acid, and gallic acid were found to be weak Fe-binding ligands that increased the persistence of dissolved Fe, regenerating Fe(II) in seawater from 0.05 % to 11.92 % (González et al., 2019). Hassler et al. (2011) reported that the addition of glucuronic acid to natural planktonic assemblages increased iron bioavailability for eukaryotic phytoplankton. Furthermore, iron bioavailability also increased when three different saccharides were used in cultured and natural eukaryotic phytoplankton populations, suggesting that this is a generalizable phenomenon. The metal reducing and chelating properties of polysaccharides are highly dependent on several factors, with the presence of covalently and non-covalently bound phenolic and protein components being the main contributing factor, allowing them to exhibit radical scavenging activity (RSA) and metal reduction capacity that they would not exhibit if devoid of these components (Fernandes and Coimbra, 2023).
The effect of pH changes on the speciation of metal–organic complexes and on the redox kinetics of metals in the marine environment is not as well characterized as that of inorganic ligands due to the heterogeneous composition of dissolved organic matter and their unknown structure. Therefore, studying the nature of these organic ligands will allow a comprehensive understanding of the consequences of acidification on ocean biogeochemical processes.
This work aimed to determine how marine acidification may affect the composition of cells and exudates from Emiliania huxleyi. Therefore, cultures of E. huxleyi were grown at four different pH conditions (7.75, 7.90, 8.10, and 8.25) reached by bubbling CO2 in the culture seawater (Samperio-Ramos et al., 2017). The four experimental scenarios represent interglacial, close to contemporary, and two future ocean acidification conditions based on the Intergovernmental Panel on Climate Change projections (IPCC, 2014). Intra- and extracellular phenolic compounds (gallic acid (GAL), protocatechuic acid (PCA), p-coumaric acid (COU), ferulic acid (FA), catechin (CAT), vanillic acid (VAN), epicatechin (ECAT), syringic acid (SYR), rutin (RU), and gentisic acid (GA)) were identified and quantified by reverse-phase high-performance liquid chromatography (RP-HPLC). The total carbohydrate content of cells was quantified using the phenol–sulfuric acid (PSA) assay (Dubois et al., 1956). The presence of antioxidants was assessed by the antioxidant activity of cell extracts and exudates determined by measuring their iron reducing power (FRAP) and their ability to inhibit the stable radical 2,2-diphenyl-1-picrylhydrazyl (DPPH) (Sethi et al., 2020).
2.1 Chemicals
Methanol (HPLC gradient grade) was purchased from Scharlab (Barcelona, Spain). Formic acid (synthesis grade), Fe3Cl • 6H2O, and FeSO4 • 7H2O were supplied by Panreac (Barcelona, Spain) and D-Glucose (Glc), phenol, DPPH, and 2,4,6-tri(2-pyridyl)-triazine (TPTZ) by Sigma-Aldrich (St. Louis, MO, USA). Polyphenol standards were supplied as follows: GAL, PCA, COU, FA, CAT, VAN, ECAT, and SYR by Sigma-Aldrich Chemie (Steinheim, Germany) and RU and GA by Merck (Darmstadt, Germany). Ultrapure water was obtained from a Milli-Q system from Millipore (Bedford, MA, USA).
2.2 Cultures
Axenic cultures of E. huxleyi (strain RCC1238) were supplied by the Spanish Bank of Algae (BEA) in f/2 medium. The E. huxleyi coccolithophore was cultured for 8 d with an initial cell density of 106 cells L−1 in an incubator clean chamber (Friocell FC111) at a constant temperature of 25 °C. The light intensity was 200 µmol photons m−2 under a complete photoperiod (24 h). Different pCO2-controlled seawater pH conditions (7.75, 7.90, 8.10, 35 and 8.25) were used and measured on the free hydrogen ion scale pHF = log[H+] with a ROSS combination glass body electrode calibrated daily with TRIS buffer solutions. A gaseous mixture of CO2-free air and pure CO2 was bubbled in the culture medium to CO2 levels of 900 µatm (pH 7.75), 600 µatm (pH 7.90), 350 µatm (pH 8.10), and 225 µatm (pH 8.25). Two gas cylinders were used for each incubator. To ensure quasi-constant seawater carbonate chemistry, a solenoid valve connected to both gas cylinders (pure CO2-free air cylinder and pure CO2 cylinder) and a pH controller modulates the CO2 flow rate once the desired pH is reached, keeping it constant (± 0.02) (Samperio-Ramos et al., 2017). The culture medium was sterile-filtered (0.1 µm) North Atlantic seawater (S=36.48) obtained at the European Station for Time-Series in the Ocean of the Canary Islands (ESTOC; 29°10′ N, 15°30′ W). To maintain suspended cells and homogeneity of CO2 in solution, the cultures were shaken at 60 rpm with a Teflon-coated magnetic stirrer. All materials were cleaned following a standard protocol (Achterberg et al., 2001) and subsequently autoclaved at 121 °C for 30 min prior to use. Once the seawater reached the desired pCO2/pH value, coccolithophorids were inoculated into the batch cultures. Stock cultures were maintained in the experimental conditions for 48 h before starting each experiment, which allowed acclimation to the CO2 concentration. Five experimental batch cultures were carried out at each pH treatment. Seawater enriched with exudates was filtered using acid-cleaned and combusted polycarbonate (Nucleopore) syringe filters (0.45 µm) to prevent cell breakage. Cells were frozen and stored at −80 °C.
The measurements and calculation of the carbon dioxide system for this study were previously described by Samperio-Ramos et al. (2017). Carbonate chemistry was monitored continuously in the experimental media and determined from pH, total alkalinity (TA), and total dissolved inorganic carbon (DIC) (González-Dávila et al., 2011; Samperio-Ramos et al., 2017). Briefly, pH in the treatments was measured on the free hydrogen ion scale (pH = ), by immersing Orion ROSS combination glass electrodes in the experimental media. The electrodes were calibrated daily using TRIS buffer solutions. The equilibration of the gas in the media of each treatment was achieved after a maximum of 24 h, observed by the evolution of pH. TA and DIC were measured at the beginning and end of the experiment on days 0 and 8, utilizing a VINDTA 3C system as described in detail by González-Dávila et al. (2011). TA was determined by potentiometric titration with standardized 0.25 M hydrochloric acid (0.45 M in NaCl) until the endpoint of carbonic acid was reached (Mintrop et al., 2000). DIC was analysed via a coulometric procedure after phosphoric acid addition. Certified reference material (provided by A. Dickson at Scripps Institution of Oceanography) was employed to assess the performance of the titration system, yielding an accuracy of ± 1.5 and ± 1.0 µmol kg−1 for TA and DIC, respectively. The Seawater Carbonate package (SeaCarb version 3.0), developed for RStudio software (R Development Core Team; Gattuso et al., 2021), was employed to calculate the values of pCO2, using the experimental results of pH, dissolved inorganic carbon, and total alkalinity and considering the carbonic acid dissociation constants of Millero et al. (2006). A more detailed description is given by Samperio-Ramos et al. (2017).
2.3 Cells and exudates extract preparation prior to HPLC, carbohydrates, and antioxidant activities quantifications
Cells cultured for 8 d in 700 mL of seawater were freeze-dried and suspended in 5 mL of methanol. The samples were sonicated for 10 min, stirred for 5 min, and centrifuged at 7000 rpm for 15 min. The supernatant was separated, and the residue was extracted with 15 mL of methanol for 30 min and centrifuged again. All supernatants were collected and evaporated to dryness, and the resulting residue was dissolved in 5 mL of methanol. These samples were used for phenolic compound identification, DPPH inhibition, and FRAP value determinations. For carbohydrates, freeze-dried cells were suspended in 5 mL of acidified water (pH = 2). The samples were sonicated for 10 min, stirred for 5 min, and centrifuged at 7000 rpm for 15 min, and the supernatant was separated.
Seawater samples (700 mL) enriched with exudates were previously subjected to solid-phase extraction (SPE) at a flow rate of 2 mL min−1 using Macherey-Nagel Chromabond Easy cartridges (500 mg). The retained analytes were eluted with 12 mL of methanol, which was subsequently evaporated on a rotary evaporator. The residue was dissolved in 300 µL of methanol and filtered through a 0.22 µm filter to be injected into the HPLC equipment. These samples were used to quantify phenolic compounds and DPPH inhibition values.
2.4 Chlorophyll a
Chlorophyll a (Chl a) was determined according to Branisa et al. (2014) with modifications. Frozen cells were suspended in 5 mL of acetone : hexane (3 : 2) and sonicated for 3 min in an ice water bath. Homogenates were centrifuged at 7500 rpm for 5 min, and the absorbance (A) of supernatants was measured at 645 and 663 nm. Chl a was expressed as femtogram per cell and quantified spectrophotometrically according to following equation: Chl a (mg per 100 mL) = 0.999 × .
2.5 Phenolic profile of E. huxleyi cells and exudates
Methanol extract of cells (2 mL) prepared as described in Sect. 2.3 was evaporated; the residue was dissolved in methanol (300 µL) and filtered with a syringe filter (0.2 µm). Chromatographic analysis of cells and exudates was carried out according to Santiago-Díaz et al. (2023), with JASCO LC-4000 HPLC equipment provided with a PU-4180 quaternary pump, an AS-4150 autosampler, an MD-4015 photodiode array detector, an LC-Net ll interface, a Phenomenex C18 column (250 mm × 4.6 mm, 5 µm), and a Phenomenex guard column maintained at 30 °C. The elution was performed with water containing 0.1 % formic acid (phase A) and methanol (phase B), with a flow rate of 1 mL min−1 and injection volume of 10 µL. The gradient elution method for A was from 0 to 5 min, 75 % isocratic; from 5 to 30 min, from 75 % to 40 % linear gradient; and finally, the column was washed and reconditioned. For quantification, simultaneous monitoring was set at 270 nm (GAL, PCA, CAT, VAN, RU, ECAT, and SYR) and 324 nm (GA, COU, and FA). Samples were analysed in triplicate, and the results were expressed as attomole per cell (amol per cell).
2.6 Determination of carbohydrate content
The total carbohydrate contents in E. huxleyi cells were determined following the phenol sulfuric acid method (Dubois et al., 1956) with some modifications. The cells extract (1.5 mL) was concentrated, and the residue was dissolved in 0.8 mL of water. The reaction was carried out by mixing 80 µL of the sample or the standard with 150 µL of phenol (5 %) and 1 mL of concentrated sulfuric acid. The resulting solution was heated at 100 °C for 5 min, and the absorbance was recorded at 490 nm in a UV–visible spectrophotometer. The results were expressed as femtomole of glucose equivalent per cell (fmol Glc eq per cell) calculated from the calibration curve of 0.14 to 2.78 mM glucose, , and R2=0.9971.
2.7 Antioxidant activities of algae and exudates
The antioxidant properties of the cell extracts were evaluated according to Sethi et al. (2020) with modifications. The ability to reduce complex TPTZ-Fe(III) to TPTZ-Fe(II) by donating an electron was measured as follows: the FRAP reagent was prepared with acetate buffer solution 0.3 M (pH 3.6), 10 mM of TPTZ in HCl (40 mM), and 2.5 mL of FeCl3 • 6H2O solution (20 mM) (in the ratio of 10 : 1 : 1). Methanol extracts (50 µL) were mixed with 1 mL of freshly prepared and pre-warmed FRAP reagent (37 °C) for 13 min. The mixture was introduced in an ice bath, and the absorbance was measured at 593 nm. Results were expressed as femtomole of reduced Fe(III) per cell and calculated from a calibration curve constructed with FeSO4 • 7H2O concentrations ranging from 0.15 to 2.20 mM (regression line equation , R2=0.996). The estimation was carried out in triplicate, and the results were averaged.
The capacity of cell extracts and exuded compounds of inhibiting DPPH radicals was determined by mixing 0.8 mL of the DPPH solution (0.053 mM) with 30 µL of cell extracts and with 50 µL for each sample of exuded compounds. The absorbance was recorded after 20 min at 515 nm using a UV–visible spectrophotometer (Shimadzu Pharmaspec 1800). The total amount of inhibited DPPH radical per cell was calculated from a calibration curve of 0.03 to 0.1 mM DPPH, , and R2=0.9996.
2.8 Statistical analysis
A Pearson's correlation test and a one-way ANOVA were performed to determine the degree of relationship between pairs of variables and statistically significant differences between measurements, respectively. Both studies were conducted using the Jamovi program (Jamovi, 2022), and p values of < 0.05 were considered statistically significant.
3.1 Carbonate chemistry parameters
Preliminary tests indicated good stability of carbonate chemistry parameters in media, obtained by the CO2 regulation system (Table 1). After CO2 equilibration, initial DIC values ranged from 1905 ± 26 to 2215 ± 16 at a TA mean value of 2386 ± 16 µmol kg−1 (ANOVA; F=1.729, p=0.2382 among treatments), corresponding to a CO2 range of 225 ± 1 and 914 ± 13 µatm. Although carbonate chemistry in CO2-manipulated experiments can be strongly affected by biological activity (Howes et al., 2017; Miller and Kelley, 2021), during our research TA and DIC remained fairly stable (t tests; p>0.05) within treatments, over the 8 d experimental period.
3.2 Cell growth
The acidification conditions did not significantly affect the final cell densities (Samperio-Ramos et al., 2017), which increased in 8 d from an initial value of 106 cells L−1 to 9.98 (± 0.53) × 107 (pH 8.25), 1.07 (± 0.13) × 108 (pH 8.10), 1.04 (± 0.07) × 108 (pH 7.90), and 9.15 (± 0.41) × 107 (pH 7.75) (Fig. 1). After 8 d, growth rates remained fairly constant in all experimental acidification conditions, indicating that the stationary phase had been reached. Heidenreich et al. (2019) also concluded that growth rates of both haploid and diploid E. huxleyi cells were unaffected by ocean acidification (culture medium adjusted to pCO2 400 vs. 1000 µatm). Fukuda et al. (2014) reported similar results for E. huxleyi harvested in CO2-enriched seawater, whose growth was unaffected. However, the authors observed different responses depending on the acidification method; i.e. E. huxleyi showed severe growth damage in experiments carried out by acidification by HCl, which also changes the seawater alkalinity, which does not happen when bubbling CO2 is used, concluding that coccolithophore E. huxleyi has the ability to respond positively to CO2 acidification. In contrast to these results, Vázquez et al. (2022) found that acidification with CO2 (1200 µatm, pH 7.62) induced lower coccolithophore growth rates than acidification reached without CO2 enrichment compared to the control (400 µatm, pH 8.10). In addition, elevated CO2 affected cell viability and promoted the ROS accumulation, effects not observed under low pH without CO2 additions.
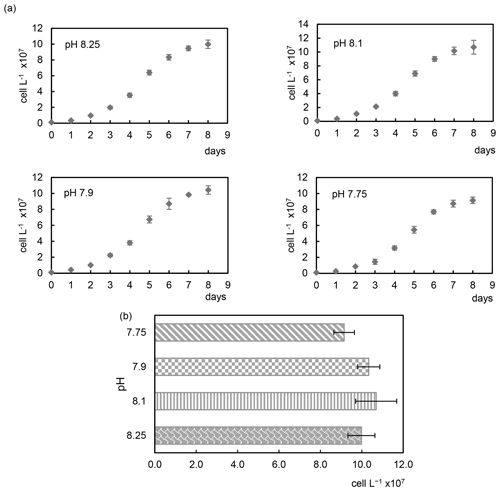
Figure 1Growth curves (a) and cell densities (b) of the coccolithophore Emiliania huxleyi cultivated under four different pH conditions.
Discrepancies found in the literature regarding acidification effects and responses of E. huxleyi coccolithophores may be due to different environmental factors and culture conditions, among other things (Gafar et al., 2019; Tong et al., 2019). Langer et al. (2009) observed substantial differences in sensitivity to acidification in four different E. huxleyi strains with different responses in all parameters tested.
Bautista-Chamizo et al. (2019) exposed microalgae Tetraselmis chuii (T. Chuii), Nannochloropsis gaditana (N. gaditana), and P. tricornutum separately to pH 6.0, 7.4, and pH 8.0 as a control, observing growth inhibition of 12 %, 61 %, and 66 % at a pH of 6.0, respectively, with T. chuii being the most resistant species to CO2 enrichment. At a pH of 7.4 only P. tricornutum showed a significant decrease in cell abundance (16 %), while no differences were found for T. chuii and N. gaditana, demonstrating that sensitivity to acidification depends on the microalgae species. However, the growth rate of the three microalgae Chlorella sp., P. tricornutum, and Chaetoceros muelleri (C. muelleri) harvested at pH levels 8.1, 7.8, and 7.5 was significantly enhanced (Jia et al., 2024). The differences observed in the growth behaviour of microalgae, mainly of P. tricornutum diatoms, in these two last studies could be due to the culture conditions. For example, in the first study, Bautista-Chamizo et al. (2019) used seawater collected in the Bay of Cádiz (Spain) (with initial pH 8.0, salinity of 34, temperature at 23 °C, and initial cell density 3×104 cells mL−1), and in the second study Jia et al. (2024) used artificial seawater prepared by mixing sea salt (Haizhixun, China) with purified water (salinity of 30 ± 1 %, temperature maintained at 18 ± 0.5 °C, initial pH at 8.1, no data on initial cell density).
3.3 Chlorophyll a
After 8 culture days, the concentration of Chl a per cell decreases with decreasing pH from 56.6 ± 2.8 fg per cell (pH 8.25) to 26.8 ± 1.4 fg per cell (pH 7.9). However, cells grown in the most acidic conditions (pH 7.75) show the highest amount of Chl a (67.3 ± 2 fg per cell), with a significant increase observed between pH 8.1 (45.1 ± 3 fg per cell) and 7.75 (p < 0.01). These results agree with those reported by Vázquez et al. (2022), who observed significantly increased cellular concentration of Chl a in the high pCO2 treatment of E. huxleyi cells (1200 µatm, pH 7.62) with respect to the control (400 µatm, pH 8.10). Crawfurd et al. (2011) also studied the effect of elevated CO2 on cultures of the diatom Thalassiosira pseudonana CCMP1335 in a pH range between 7.8 and 8.1 (760 and 380 µatm, respectively), concluding that chlorophyll content increased in cells grown at a pH of 7.8 compared to those grown at a pH of 8.1, but photosynthetic efficiency remained constant in both experiments. Higher levels of Chl a were also observed in microalgae Chlorella sp., P. tricornutum, and C. muelleri harvested at a pH of 7.8 and 7.5 than those quantified at a pH of 8.1, concluding that primary producers that can utilize HCO as the carbon source benefit from elevated CO2 concentration (Jia et al., 2024). However, Yu et al. (2022) found no significant changes in cellular and total content of Chl a of E. huxleyi cells harvested at similar pH compared to those here (between 7.72–7.76 under elevated CO2 (1000 µatm) and between 8.03 and 8.07 under atmospheric CO2). Mackey et al. (2015) concluded that photosynthetic responses to ocean acidification are highly variable throughout species and taxa, with different E. huxleyi strains exhibiting opposite responses to elevated CO2 on maximum photosynthetic rates.
3.4 Phenolic contents of cells and exudates
The phenolic profiles of cell extracts and seawater samples enriched with E. huxleyi exudates are summarized in Table 2. GAL, PA, ECAT, and RU were detected in both cells and exudates, whereas VAN and COU were only detected in cells. The other polyphenols were below the limit of quantification (LOQ). Under all pH conditions, PA, ECAT, and RU were detected in the seawater enriched with exudates and VAN in cells.
Table 2Amounts of intracellular and exuded phenolic compound by cells of E. huxleyi grown under different pH conditions.
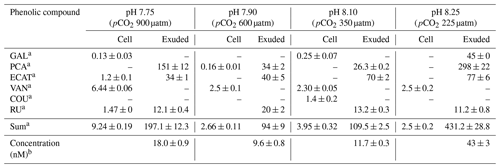
a Results are expressed as attomole per cell (means ± standard deviation of two measurements). b Results are expressed as nanomole L−1 (means ± standard deviation of two measurements). Abbreviations: GAL – gallic acid; PCA – protocatechuic acid; ECAT – epicatechin; VAN – vanillic acid; COU – p-coumaric acid; RU – rutin.
Samperio-Ramos et al. (2017) reported an increase in dissolved organic carbon (DOC) exudation by 19 % and 15 % during exponential and stationary phases, respectively, as CO2 levels increased from 225 µatm (177.06 ± 10.95 fmol C per cell per day) to 900 µatm (209.74 ± 50.00 fmol C per cell per day) in the culture medium. The authors suggested that ocean acidification could significantly enhance the release of phenolic compounds when E. huxleyi is grown under low-iron conditions. They detected phenolic compounds only in the stationary phase, and their release rate was affected by CO2 conditions, observing a strong correlation between the concentrations of produced phenolic compounds with exuded DOC, indicating that these compounds constituted a relatively constant fraction of the organic matter excreted by E. huxleyi. Extracellular release of phenolic compounds was statistically higher at a pH of 7.75 (0.41 ± 0.02 fmol C per cell per day) than at a pH of 8.1 (0.36 ± 0.02 fmol C per cell per day; Tukey contrast: t value = 2.495; p < 0.1). While Samperio-Ramos et al. (2017) studied the total polyphenol contents through the Arnow spectrophotometric assay, in this study individual polyphenols were identified (Table 2), concluding that the highest concentrations of these polyphenols identified were exuded in the cultures at a pH of 8.25 (43 ± 3 nM) and 7.75 (18.0 ± 0.9 nM) (Fig. 2).
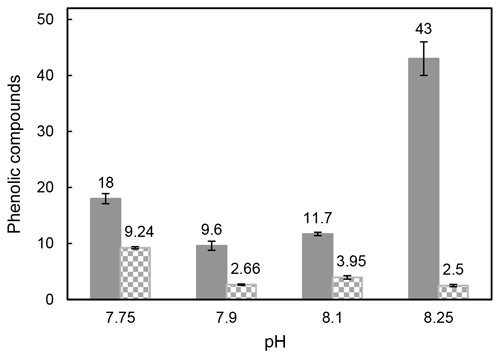
Figure 2Total sum of the identified intracellular polyphenols expressed as attomole per cell (non-solid bars) and concentration (nM) of exuded polyphenols (solid bars) by Emiliania huxleyi cells grown under reduced pH conditions after 8 culture days.
The content of phenolic compounds inside the cells increased significantly (p < 0.01) at a pH of 7.75, reaching the maximum level (9.24 ± 0.19 amol per cell) compared with that at a pH of 8.1 (Fig. 2). These results agree with those reported by Jin et al. (2015), who evidenced that phytoplankton grown under the CO2 levels predicted for the end of this century showed accumulation of phenolic compounds increased by 46 %–212 % compared to those obtained at the current CO2 level. Subsequently, zooplankton fed with phytoplankton grown in acidified seawater showed 28 % to 48 % higher phenolic content. This transfer of accumulated phenolic compounds to higher trophic levels could have serious consequences for the marine ecosystem and seafood quality. Dupont et al. (2014) have shown that survival of adult boreal shrimp (Pandalus borealis) was affected when exposed for 3 weeks at a pH of 7.5 with an increase in mortality of 63 % compared to those grown at a pH of 8.0 (pH at the sampling site), also showing changes in appearance and taste. Polyphenol accumulation has also been observed in terrestrial plants (Bai et al., 2019; Kaur et al., 2022; Lwalaba et al., 2020). In contrast, no significant changes were observed by Jia et al. (2024) in the three microalgae species Chlorella sp., P. tricornutum, and C. muelleri harvested under elevated pCO2 (pH 8.1, 7.8, and 7.5), and Arnold et al. (2012) also reported a loss of phenolics in the seagrasses Cymodocea nodosa, Ruppia maritima, and Potamogeton perfoliatus grown in acidified seawater, where the pH decreased up to 7.3, and the CO2 level increased 10-fold.
Vázquez et al. (2022) showed that CO2 enrichment aeration (1200 µatm, pH 7.62) induced metabolic stress and accumulation of ROSs in the E. huxleyi strain used in their experiments. This could explain the accumulation of phenolic compounds at a pH of 7.75 (9.24 ± 0.19 amol per cell), considered to play a significant role as a defence mechanism due to their antioxidant and ROS scavenging properties (Chvátalová et al., 2008; Gauthier et al., 2020; Shay et al., 2015). Previous studies on diatoms P. tricornutum grown under copper stress showed a significant correlation (r=0.9999; p < 0.05) between accumulated phenolic compounds and malondialdehyde (MDA), commonly produced by an increase in ROSs (Rico et al., 2024). Bautista-Chamizo et al. (2019) reported an increase in ROS production by decreasing the pH of the culture medium in single-species and multispecies toxicity assays conducted with microalgae T. Chuii, N. gaditana, and P. tricornutum. The species P. tricornutum and N. gaditana exposed to pH 7.4 and pH 6.0 exhibited a significant increase in the percentage of intracellular ROSs, which was more pronounced for N. gaditana cells at a pH of 6.0. Consistent with the loss of cell membrane integrity observed after 48 h at a pH of 6.0, 10 % and 56 % of non-viable cells were found for P. tricornutum and N. gaditana, respectively, while viable cells remained close to the control for T. chuii cells.
At the lowest pH of 7.75 in our study, the intracellular phenolic content experienced the maximum increase with respect to the initial one (3.7 times higher). More interesting is the significant increase of VAN inside the cells (p < 0.001) and its absence outside. VAN does not exhibit iron chelating ability due to the lack of catechol or galloyl moiety but inhibits Fe(II) oxidation and scavenges radicals (Chvátalová et al., 2008). On the other hand, RU, ECAT, and GAL detected inside cells only at this more acidic pH are also known radical scavengers with the ability to neutralize ROSs in the cells, with reducing power and metal chelating abilities (Chvátalová et al., 2008; Gauthier et al., 2020; Shay et al., 2015). Therefore, this increase of phenolics may be explained by their potential to scavenge harmful ROSs, whose enhanced production has been linked to the pH decrease by Vázquez et al. (2022) and Bautista-Chamizo et al. (2019).
No correlation was found between phenolic compounds inside and outside the cells considering the pH 8.25 data. The identified exuded compounds significantly decreased (p < 0.05) from 43 ± 3 nM at a pH of 8.25 to 9.6 ± 0.8 nM at a pH of 7.90 (Table 2). These results partially agree with those reported by López et al. (2015) for Dunaliella tertiolecta growing under stress conditions induced by high levels of copper, where the concentration of phenolic compounds declined from 9.4 ± 0.6 nM in seawater cultures without Cu addition to 8.4 ± 0.4 and 8.6 ± 0.4 nM in the two copper enrichment experiments and increased 1.4 times concerning the control into the cells grown under the highest Cu level. However, the extracellular phenolic compound's behaviour followed the same tendency to that described above for intracellular compounds between pH 8.1 and 7.75, where a high correlation was found between intra- and extracellular phenolic compounds (p < 0.001). Total exuded phenolic compounds identified here were significantly enhanced in this pH interval (p < 0.05) to 18.0 ± 0.9 nM at the predicted pH for future scenarios (pH 7.75) over those quantified at a pH of 8.1 (11.7 ± 0.3). Significant differences were found in PCA and ECAT amounts (p < 0.01 and 0.05, respectively), which together with RU were detected in all exudates. Wu et al. (2016) studied the interaction between Fe and 10 phenols at a pH of 8.0, finding that Fe(II) was rapidly oxidized under alkali conditions (pH 8.0 ± 0.1) even in the presence of GA, SYR, COU, VAN, and FA, which did not show any protection effect for ferrous iron in these conditions. However, caffeic acid, GAL, and PCA protected 69 %, 64 %, and 33 % of the initial concentration of Fe(II), respectively, due to the chelating capacity of the catechol and galloyl groups with Fe(II). They reported the formation of relatively stable phenolic–Fe complexes under alkaline conditions helping to weaken iron precipitation. Therefore, the high level of polyphenols with chelating ability exuded at a pH of 8.25 in this study could be due to the redox chemistry of inorganic Fe, intimately linked to the pH (Pérez-Almeida et al., 2019), specifically the low Fe concentration under these conditions. When the pH increases, the oxidation rate constants of Fe(II) also increase, and the solubility of inorganic Fe(III) decreases. Polyphenols modify Fe(II) oxidation rates by promoting the reduction of Fe(III) to Fe(II) in seawater. The effect of GAL on Fe oxidation and reduction was studied by Pérez-Almeida et al. (2022), concluding that it reduces Fe(III) to Fe(II) in seawater, with a more pronounced effect as pH decreases, allowing Fe(II) to be present for longer periods and improving its bioavailability. The authors found that 69.3 % of the initial Fe(II) was oxidized after 10 min at a pH of 8.0 in the absence of GAL, while only 37.5 % was oxidized in its presence at a concentration of 100 nM after 30 min. The reduction of Fe(III) to Fe(II) by GAL was faster as pH decreased. The same results were observed for catechin and sinapic acid, which also favoured the regeneration of Fe(II) in seawater, increasing the amount of regenerated Fe(II) as pH decreased, concluding that acidification may contribute to an increase in the level of reduced iron in the environment (Santana-Casiano et al., 2014). This could be the reason for the decrease of GAL and the remaining phenolic compounds in the extracellular medium until a pH of 7.9 is reached, where acidification does not seem to produce extreme adverse effects in E. huxleyi, as observed in other species (Bautista-Chamizo et al., 2019), and the increase of their presence inside the cell as the pH decreases. Changes in the bioavailability of Fe and other trace metals linked to pH variation could affect the control of phytoplankton growth and have a major influence on the biogeochemical cycling of carbon and other bioactive elements in the ocean (Shaked et al., 2020; Tagliabue et al., 2017).
3.5 Total carbohydrate content of cells
Total carbohydrates in cells and exudates of E. huxleyi are shown in Table 3. No correlation was found between intra- and extracellular contents. During the exponential phase, the contribution of dissolved carbohydrates to excreted DOC was higher (18 %–37 %) than during the stationary phase (14 %–23 %), significantly increased as time elapsed from the exponential to the stationary phase (Samperio-Ramos et al., 2017). However, acidification of the culture medium with CO2 did not affect the levels of carbohydrates exuded per cell in any of the three growth phases, as these levels did not change significantly in any of them as the pH dropped to pH 7.75. The number of total intracellular carbohydrates also remained constant between pH 8.25 and pH 7.75. The coccolithophore E. huxleyi has shown diverse metabolic responses to ocean acidification and to combinations of ocean acidification with other environmental factors with significant differences between strains (Gafar et al., 2019; Langer et al., 2009; Mackey et al., 2015; Tong et al., 2019). Fukuda et al. (2014) observed increased production and storage of polysaccharides stimulated by acidification with CO2 enrichment. Jones et al. (2013) compared the response of the coccolithophore species E. huxleyi cultured at two pH conditions reached by bubbling CO2 (pH 7.94 and 7.47 at the time of the harvesting), when as little as 5 % of DIC was consumed, indicating low chemical shift throughout the experiments. The higher CO2 level evidenced cellular responses to stress such as decreased growth rates, but proteins associated with many key metabolic processes remained unaltered, thus maintaining many biological functions. Xie et al. (2021) studied the effects of high and low DIC concentration (from 900 to 4930 µmol kg−1) and reduced pH value (from 8.04 to 7.70) on physiological rhythms, element contents, and macromolecules of the coccolithophore Emiliania huxleyi, concluding that its response is highly dependent on the DIC. Compared to high pH conditions, low pH and DIC concentration led to increases in particulate organic carbon (POC) and particulate organic nitrogen (PON) contents with less impact on protein and carbohydrate contents; however, high DIC and low pH reduced POC, PON, protein, and carbohydrate contents. These results partially agree with those reported by Jia et al. (2024), who found that lowering seawater pH up to 7.8 and 7.5 promoted protein synthesis in microalgae Chlorella sp., P. tricornutum, and C. muelleri compared to those harvested at a pH of 8.1, concluding that acidification improves the efficiency of carbon assimilation and provides more carbon skeletons for amino acids and protein synthesis.
Table 3Intracellular and extracellular carbohydrates of Emiliania huxleyi harvested under different pH conditions.
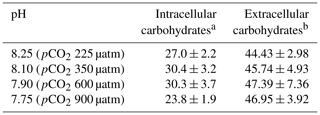
a The results are expressed as femtomole glucose equivalent per cell. b Extracellular released carbohydrates from Samperio-Ramos et al. (2017) (femtomole C per cell per day). The results are expressed as means ± standard deviation of three measurements.
Grosse et al. (2020) investigated the effects of seawater acidification on dissolved and particulate amino acids and carbohydrates in arctic and sub-arctic planktonic communities in two large-scale experiments in a pH range similar to ours here (control mesocosm: pCO2 185 µatm/pH 8.32; mesocosm pCO2 between 270 and 1420 µatm/pH 8.18–7.51). The authors concluded that the relative composition of amino acids and carbohydrates did not change as a direct consequence of increased pCO2, and the observed changes depended mainly on the composition of the phytoplankton community. Araujo and García (2005) reported that CO2 addition to the culture seawater lowered the carbohydrate content of marine diatom Chaetoceros cf. wighamii. Thornton (2009) observed that the planktonic diatom Chaetoceros muelleri grown at a pH of 6.8, 7.4, 7.9, and 8.2 showed a decreased proportion of total carbohydrate within the cells and increased levels of dissolved exuded carbohydrates into the surrounding medium with the decrease in pH. Thornton (2009) used a different method to maintain pH, a biological buffer (25 mM HEPES; Sigma-Aldrich, St. Louis, MO, USA) and daily titration with the addition of a small amount of HCl, which changes the seawater alkalinity.
Similar effects to those observed here have been found in the response of macroalgae to elevating ocean acidification. Gao et al. (2017) reported that pCO2 does not affect the carbohydrate content of algae Ulva rigida investigated under pH 7.95 and 7.55. Barakat et al. (2021) studied the effect of the acid stress on the green alga Ulva fasciata subjected to four levels of pCO2, 280, 550, 750, and 1050 µatm (pH values 7.2, 7.6, 7.86, 8.1 (control)) and found similar production of carbohydrates at the three lowest pH values (46.13 %, 46.96 %, and 46.04 % of dry weight, respectively), while the control (pH 8.1) showed 42.37 % of dry weight.
3.6 Antioxidant activities
Table 4 shows the antioxidant activities of extracts of cells E. huxleyi grown in seawater enriched with several levels of CO2 and those of the compounds exuded by these cells. Cells with the highest phenolic content (pH 7.75) gave the most increased scavenging activity (8.1 ± 0.1 fmol per cell) (p < 0.01), indicating that these cells grown in the most acidified media produce relevant amounts of antioxidants with ROS scavenging ability. In fact, a high correlation was found between the phenolics identified inside the cells and their antioxidant activity (p < 0.01). These results partially agree with those reported by Santiago-Díaz et al. (2023), who found enhanced radical scavenging activities and production of polyphenols in diatoms P. tricornutum grown under Cu stress as the Cu level increased. However, the ability to reduce iron (FRAP) remains almost constant in the cells and correlates with the intracellular phenolic content (p < 0.05). The FRAP test is based on the transfer of an electron, whereas the DPPH test includes the transfer of both a hydrogen atom and an electron. These different chemical reactions mechanisms and their kinetics could explain the differences between FRAP and DPPH results. In addition, the antioxidant activity of extracts and exudates depends on their complex mixture, ratios of components, and their interactions, which may be synergistic, additive, or antagonistic, as well as the assay kinetics (Šamec et al., 2021; Sethi et al., 2020). Concerning the exudates, no significant differences were found in their RSA despite the fact that significant changes in the total exuded phenolic compounds per cell were detected (p < 0.05).
Table 4Antioxidant activities of exudates and extracts of E. huxleyi cells grown in seawater enriched with CO2.
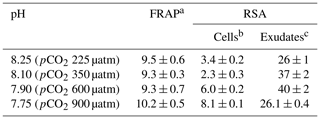
a Results are expressed as femtomole of reduced Fe(III) per cell. b The results are expressed as femtomole of inhibited DPPH per cell. c The results are expressed as nanomole of inhibited DPPH L−1. All results are expressed as means ± standard deviation of three measurements. Abbreviations: FRAP – ferric-reducing antioxidant power; RSA – radical scavenging activity.
The results found in the literature on the effect of acid stress on the biochemical composition of microorganisms and their exudates are apparently contradictory. However, a large number of factors (initial cell density, grown phase, culture media composition, light photoperiod and light intensity applied during culture, pH, among others) strongly influence the toxicity of pollutants in algal bioassay stimulating and/or inhibiting the production of different metabolites through the regulation of metabolic pathways (Barcelos e Ramos et al., 2010; Santiago-Díaz et al., 2023; Singh and Shrivastava, 2016; Tandon et al., 2017; Zhang et al., 2019) and making the responses of marine organisms to ocean acidification highly complex and dependent on these factors (Grosse et al., 2020). Changes in seawater pH influence the protonation of biological molecules and could modify their charge and negatively affect metabolic processes. Several mechanisms have been described to maintain the pH inside cells, such as changing the lipid composition of the cytoplasmic membrane or increasing the production of cyclopropane fatty acids to reduce proton permeability (Lund et al., 2020).
Changes in pH generate stress conditions, either because they drastically decrease the availability of trace metals such as iron, essential in the life cycle of microalgae, which modify the composition of their exudates to protect and internalize Fe(II) at alkaline pH where it is rapidly oxidized to Fe(III) (Wu et al., 2016), or because ROSs are increased with low pH (Bautista-Chamizo et al., 2019; Vázquez et al., 2022). On the other hand, the pH range for optimal growth varies depending on the microalgae species, and even the sensitivity of E. huxleyi to acidification appears to be strain-specific (Langer et al., 2009). Borchard and Engel (2015) found no significant differences between growth rates and primary production (composed of dissolved and particulate organic carbon) of E. huxleyi grown at current and high CO2 concentration due to its ability to acclimate.
However, numerous experimental studies show that most coccolithophores cultured at elevated CO2 reduce their level of calcification with a tendency to produce degraded or aberrant coccoliths that calcify even less and will spread in a future high-CO2 ocean (Langer et al., 2009; Lohbeck et al., 2012; Mackey et al., 2015). The ecological consequences of ocean acidification on the competitiveness of coccolithophores in mixed phytoplankton communities are unclear. The microalgae species T. Chuii, N. gaditana, and P. tricornutum showed a higher cell abundance when grown alone under acidification conditions than in multispecies toxicity tests (Bautista-Chamizo et al., 2019). In addition, although oxidative stress in N. gaditana was significantly higher in the single-species tests, the ROS levels were higher in P. tricornutum and T. chuii in the multispecies tests, suggesting that algal interactions had an effect on pH toxicity in these species but in a species-specific way. However, the effects of competition between these three species were recorded at a pH of 8, but they were eclipsed by the effect of CO2 acidification. Several studies evidenced enhanced oxidative stress in cells in acidified seawater, through an increase in ROSs that correlated significantly with the accumulation of polyphenols (Bautista-Chamizo et al., 2019; Vázquez et al., 2022). These changes may cause cascading effects in the marine food web, varying the macromolecular composition of consumers (Jia et al., 2024). Ocean acidification will directly affect marine organisms, altering the structure and functions of ecosystems. The accumulation of phenolic compounds leads to functional consequences in primary and secondary producers, with the possibility that fishery industries could be influenced as a result of progressive ocean change (Gattuso et al., 2015; Jin et al., 2015; Trombetta et al., 2019).
Acidification between the current pH of the oceans (8.1) and the future scenario of pH 7.75 leads to an increase in polyphenol production in E. huxleyi cells and their free-radical inhibitory activity. More importantly, the change in the polyphenol profile between cells and exudates and between pH conditions should be closely related not only to their antioxidant activity under stress conditions, but also to the chemistry of iron and other trace metals and their bioavailability under different pH conditions. Intra- and extracellular carbohydrate levels did not show modifications with decreasing pH. These changes in metabolites with different capacity to inhibit radicals and complex metals, whose accumulation is associated with enhanced oxidative stress, are potential factors leading to readjustments in phytoplankton community structure and diversity and possible alteration in marine ecosystems.
Data are available from the corresponding author upon reasonable request.
MR: design and conceptualization, methodology, supervision, SPE seawater concentration, preparation of samples for HPLC analysis, FRAP and DPPH activity assays, data analysis, and manuscript writing; PS-D: carbohydrate and HPLC phenolic analysis; GS-R: study of cells grown; JMS-C and MG-D: design and conceptualization of cell culture, critical review of the manuscript, project management, funding, and resourcing. All authors approved the submitted version.
The contact author has declared that none of the authors has any competing interests.
Publisher's note: Copernicus Publications remains neutral with regard to jurisdictional claims made in the text, published maps, institutional affiliations, or any other geographical representation in this paper. While Copernicus Publications makes every effort to include appropriate place names, the final responsibility lies with the authors.
This study received financial support from the FeRIA project (PID2021-123997NB-100) of the Spanish Ministerio de Ciencia e Innovación. The participation of Paula Santiago was funded through a PhD scholarship from the Universidad de Las Palmas de Gran Canaria (PIFULPGC-2019) to join the PhD programme in Oceanography and Global Change (DOYCAG). The programme is promoted by the Institute of Oceanography and Global Change (IOCAG).
This paper was edited by Cindy De Jonge and reviewed by five anonymous referees.
Achterberg, E. P., Holland, T. W., Bowie, A. R., Mantoura, R. F. C., and Worsfold, P. J.: Determination of iron in seawater, Anal. Chim. Acta, 442, 1–14, https://doi.org/10.1016/j.talanta.2018.05.071, 2001.
Araujo, S. C. and García, V. M. T.: Growth and biochemical composition of the diatom Chaetoceros cf. wighamii brightwell under different temperature, salinity and carbon dioxide levels. I. Protein, carbohydrates and lipids, Aquaculture, 246, 405–412, https://doi.org/10.1016/j.aquaculture.2005.02.051, 2005.
Arnold, T., Mealey, C., Leahey, H., Miller, A. W., Hall-Spencer, J. M., Milazzo, M., and Maers, K.: Ocean Acidification and the loss of phenolic substances in marine plants, PLoS One, 7, e35107, https://doi.org/10.1371/journal.pone.0035107, 2012.
Arundhathy, M., Jyothibabu, R., and Santhikrishnan, S.: Coccolithophores: an environmentally significant and understudied phytoplankton group in the Indian Ocean, Environ. Monit. Assess., 193, 144, https://doi.org/10.1007/s10661-020-08794-1, 2021.
Bai, Y., Wang, G., Cheng, Y., Shi, P., Yang, C., Yang, H., and Xu, Z.: Soil acidification in continuously cropped tobacco alters bacterial community structure and diversity via the accumulation of phenolic acids, Sci. Rep., 9, 12499, https://doi.org/10.1038/s41598-019-48611-5, 2019.
Barakat, K. M., El-Sayed, H. S., Khairy, H. M., El-Sheikh, M. A., Al-Rashed, S. A., Arif, I. A., and Elshobary, M. E.: Effects of ocean acidification on the growth and biochemical composition of a green alga (Ulva fasciata) and its associated microbiota, Saudi J. Biol. Sci., 28, 5106–5114, https://doi.org/10.1016/j.sjbs.2021.05.029, 2021.
Barcelos e Ramos, J., Müller, M. N., and Riebesell, U.: Short-term response of the coccolithophore Emiliania huxleyi to an abrupt change in seawater carbon dioxide concentrations, Biogeosciences, 7, 177–186, https://doi.org/10.5194/bg-7-177-2010, 2010.
Barcelos e Ramos, J., Ribeiro, S. C., Schulz, K. G., Coelho, F. J. R. D. C., Oliveira, V., Cunha, A., Gomes, N. C. M., Brownlee, C., Passow, U., and de Azevedo, E. B.: Emiliania huxleyi-Bacteria interactions under increasing CO2 concentrations, Microorganisms, 10, 2461, https://doi.org/10.3390/microorganisms10122461, 2022.
Bates, N. R., Astor, Y. M., Church, M. J., Currie, K., Dore, J. E., González-Dávila, M., Lorenzoni, L., Muller-Karger, F., Olafsson, J., and Santana-Casiano, J. M.: A Time-Series View of Changing Surface Ocean Chemistry Due to Ocean Uptake of Anthropogenic CO2 and Ocean Acidification, Oceanography, 27, 126–141, http://www.jstor.org/stable/24862128 (last access: 30 September 2024), 2014.
Bautista-Chamizo, E., Sendra, M., De Orte, M. R., and Riba, I.: Comparative effects of seawater acidification on microalgae: Single and multispecies toxicity tests, STOTEN, 649, 224–232, https://doi.org/10.1016/j.scitotenv.2018.08.225, 2019.
Berge, T., Daugbjerg, N., Andersen, B., and Hansen, P. J.: Effect of lowered pH on marine phytoplankton growth rates, Mar. Ecol.-Prog. Ser., 416, 79–91, https://doi.org/10.3354/meps08780, 2010.
Borchard, C. and Engel, A.: Size-fractionated dissolved primary production and carbohydrate composition of the coccolithophore Emiliania huxleyi, Biogeosciences, 12, 1271–1284, https://doi.org/10.5194/bg-12-1271-2015, 2015.
Branisa, J., Jenisova, Z., Porubska, M., Jomova, K., and Valko, M.: Spectrophotometric determination of chlorophylls and carotenoids. An effect of sonication and sample processing, J. Microbiol. Biotechnol. Food Sci., 3, 61–64, 2014.
Chvátalová, K., Slaninová, I., Březinová, L., and Slanina, J.: Influence of dietary phenolic acids on redox status of iron: ferrous iron autoxidation and ferric iron reduction, Food Chem., 106, 650–660, https://doi.org/10.1016/j.foodchem.2007.06.028, 2008.
Crawfurd, K. J., Raven, J. A., Wheeler, G. L., Baxter, E. J., and Joint, I.: The response of Thalassiosira pseudonana to long-term exposure to increased CO2 and decreased pH, PLoS One, 6, e26695, https://doi.org/10.1371/journal.pone.0026695, 2011.
Dedman, C. J., Barton, S., Fournier, M., and Rickaby, R. E. M.: The cellular response to ocean warming in Emiliania huxleyi, Front. Microbiol., 14, 1177349, https://doi.org/10.3389/fmicb.2023.1177349, 2023.
Dubois, K. M., Gilles, K. A., Hamilton, G. J. K., Rebers, P. A., and Smith, F.: Colorimetric method for determination of sugars and related substances, Anal. Chem., 28, 350–356, https://doi.org/10.1021/ac60111a017, 1956.
Dupont, S., Hall, E., Calosi, P., and Lundve, B.: First evidence of altered sensory quality in a shellfish exposed to decreased pH relevant to Ocean Acidification, J. Shellfish Res., 33, 857–861, https://doi.org/10.2983/035.033.0320, 2014.
Eltanahy, E. and Torky, A.: Microalgae as Cell Factories: Food and Feed-grade High-value Metabolites in Microalgal Biotechnology: Recent Advances, Market Potential, and Sustainability, 1–35, edited by: Shekh, A., Schenk, P., and Sarada, R., The Royal Society of Chemistry, https://doi.org/10.1039/9781839162473-00001, 2021.
Fernandes P. A. R. and Coimbra, M. A.: The antioxidant activity of polysaccharides: A structure-function relationship overview, Carbohyd. Polym., 314, 120965, https://doi.org/10.1016/j.carbpol.2023.120965, 2023.
Fukuda, S.-Y., Suzuki, Y., and Shiraiwa, Y.: Difference in physiological responses of growth, photosynthesis and calcification of the coccolithophore Emiliania huxleyi to acidification by acid and CO2 enrichment, Photosynth. Res., 121, 299–309, https://doi.org/10.1007/s11120-014-9976-9, 2014.
Gafar, N. A., Eyre, B. D., and Schulz, K. G.: A comparison of species specific sensitivities to changing light and carbonate chemistry in calcifying marine phytoplankton, Sci. Rep., 9, 2486, https://doi.org/10.1038/s41598-019-38661-0, 2019.
Gao, G., Clare, A. S., Rose, C., and Caldwell, G. C.: Eutrophication and warming-driven green tides (Ulva rigida) are predicted to increase under future climate change scenarios, Mar. Pollut. Bull., 114, 439–447, https://doi.org/10.1016/j.marpolbul.2016.10.003, 2017.
Gattuso, J. P., Magnan, A., Billé, R., Cheung, W., Howes, E., Joos, F., Allemand, D., Bopp, L., Cooley, S. R., Eakin, C. M., Hoegh-Guldberg, O., Kelly, R. P., Pörtner, H.-O., Rogers, A. D., Baxter, J. M., Laffoley, D., Osborn, D., Rankovic, A., Rochette, J., Sumalia, U. R., Treyer, S., and Turley, C.: Contrasting futures for ocean and society from different anthropogenic CO2 emissions scenarios, Science, 349, 6243, https://doi.org/10.1126/science.aac4722, 2015.
Gattuso, J.-P., Epitalon, J.-M., Lavigne, H., and Orr, J.: seacarb: seawater carbonate chemistry, R package version 3.3.0, http://cran.r-project.org/package=seacarb (last access: 7 October 2024), 2021.
Gauthier, M. R., Senhorinho, G. N. A., and Scott, J. A.: Microalgae under environmental stress as a source of antioxidants, Algal Res., 52, 102104, https://doi.org/10.1016/j.algal.2020.102104, 2020.
Giljan, G., Brown, S., and Lloyd, C. C., Ghobrial, S., Amann, R., Arnosti, C.: Selfish bacteria are active throughout the water column of the ocean, ISME Commun., 3, 11, https://doi.org/10.1038/s43705-023-00219-7, 2023.
González, A. G, Cadena-Aizaga, M. I., Sarthou, G., González-Dávila, M., and Santana-Casiano, J. M.: Iron complexation by phenolic ligands in seawater, Chem. Geol., 511, 380–388, https://doi.org/10.1016/j.chemgeo.2018.10.017, 2019.
González-Dávila, M., Santana-Casiano, J. M., Fine, R. A., Happell, J., Delille, B., and Speich, S.: Carbonate system in the water masses of the Southeast Atlantic sector of the Southern Ocean during February and March 2008, Biogeosciences, 8, 1401–1413, https://doi.org/10.5194/bg-8-1401-2011, 2011.
Grosse, J., Endres, S., and Engel, A.: Ocean acidification modifies biomolecule composition in organic matter through complex interactions, Sci. Rep., 10, 20599, https://doi.org/10.1038/s41598-020-77645-3, 2020.
IPCC: Climate Change 2014: Synthesis Report, Contribution of Working Groups I, II and III to the Fifth Assessment Report of the Intergovernmental Panel on Climate Change, AR5 Synthesis Report: Climate Change 2014 – IPCC, edited by: Core Writing Team, Pachauri, R. K., and Meyer, L. A., IPCC, Geneva, Switzerland, 151 pp., https://www.ipcc.ch/report/ar5/syr/ (last access: 7 October 2024), 2014.
Jamovi: jamovi (Version 2.3) [software], https://www.jamovi.org (last access: 7 October 2024), 2022.
Harmesa, H., Wahyudi, A. J., Lestari, L., and Taufiqurrahman, E.: Variability of trace metals in coastal and estuary: Distribution, profile, and drivers, Mar. Pollut. Bull., 174, 113173, https://doi.org/10.1016/j.marpolbul.2021.113173, 2022.
Hassler, C. S., Schoemann, V., Nichols, C. M., Butler, E. C. V., and Boyd, P. W.: Saccharides enhance iron bioavailability to Southern Ocean phytoplankton, Pr. Natl. Acad. Sci. USA, 108, 1076–1081, https://doi.org/10.1073/pnas.1010963108, 2011.
Heidenreich, E., Wördenweber, R., Kirschhöfer, F., Nusser, M., Friedrich, F., Fahl, K., Kruse, O., Rost, B., Franzreb, M., Brenner-Weiss, G., and Rokitta, S.: Ocean acidification has little effect on the biochemical composition of the coccolithophore Emiliania huxleyi, PLoS One, 14, e0218564, https://doi.org/10.1371/journal.pone.0218564, 2019.
Hogle, S. L., Barbeau, K. A., and Gledhill, M.: Heme in the marine environment: from cells to the iron cycle, Metallomics, 6, 1107–1120, https://doi.org/10.1039/c4mt00031e, 2014.
Hoppe, C. J. M., Langer, G., and Rost, B.: Emiliania huxleyi shows identical responses to elevated pCO2 in TA and DIC manipulations, J. Exp. Mar. Biol. Ecol., 406, 54–62, https://doi.org/10.1016/j.jembe.2011.06.008, 2011.
Howes, E. L., Kaczmarek, K., Raitzsch, M., Mewes, A., Bijma, N., Horn, I., Misra, S., Gattuso, J.-P., and Bijma, J.: Decoupled carbonate chemistry controls on the incorporation of boron into Orbulina universa, Biogeosciences, 14, 415–430, https://doi.org/10.5194/bg-14-415-2017, 2017.
Hunter, K. A. and Boyd, P. W.: Iron-binding ligands and their role in the ocean biogeochemistry of iron, Environ. Chem., 4, 221–232, https://doi.org/10.1071/EN07012, 2007.
Jacobson, M. Z.: Studying ocean acidification with conservative, stable numerical schemes for nonequilibrium air-ocean exchange and ocean equilibrium chemistry, J. Geophys. Res., 110, D07302, https://doi.org/10.1029/2004JD005220, 2005.
Jia, R., Yin, M., Feng, X., Chen, C., Qu, C., Liu, L., Li, P., and Li, Z.-H.: Ocean acidification alters shellfish-algae nutritional value and delivery, STOTEN, 918, 170841, https://doi.org/10.1016/j.scitotenv.2024.170841, 2024.
Jiang, J., Cao, L., Jin, X., Yu, Z., Zhang, H., Fu, J., and Jiang, G.: Response of ocean acidification to atmospheric carbon dioxide removal, J. Environ. Sci., 140, 79–90, https://doi.org/10.1016/j.jes.2023.04.029, 2023.
Jin, P., Wang, T., Liu, N., Dupont, S., Beardall, J., Boyd, P. W., Riebesell, U., and Gao, K.: Ocean acidification increases the accumulation of toxic phenolic compounds across trophic levels, Nat. Commun., 6, 8714, https://doi.org/10.1038/ncomms9714, 2015.
Jin, Q. and Kirk, M. F.: pH as a primary control in environmental microbiology: 1. Thermodynamic Perspective, Front. Environ. Sci., 6, 21, https://doi.org/10.3389/fenvs.2018.00021, 2018.
Jones, B. M., Iglesias-Rodriguez, M. D., Skipp, P. J., Edwards, R. J., Greaves, M. J., Young, J. R., Elderfield, H., and O'Connor, C. D.: Responses of the Emiliania huxleyi Proteome to Ocean Acidification, PLoS One, 8, e61868, https://doi.org/10.1371/journal.pone.0061868, 2013.
Kaur, S., Samota, M. K.: Choudhary, M. Choudhary, M., Pandey, A. K., Sharma, A., and Thakur, J.: How do plants defend themselves against pathogens-Biochemical mechanisms and genetic interventions, Physiol. Mol. Biol. Plants, 28, 485–504, https://doi.org/10.1007/s12298-022-01146-y, 2022.
Kholssi, R., Lougraimzi, H., and Moreno-Garrido, I.: Effects of global environmental change on microalgal photosynthesis, growth and their distribution, Mar. Environ. Res., 184, 105877, https://doi.org/10.1016/j.marenvres.2023.105877, 2023.
Langer, G., Nehrke, G., Probert, I., Ly, J., and Ziveri, P.: Strain-specific responses of Emiliania huxleyi to changing seawater carbonate chemistry, Biogeosciences, 6, 2637–2646, https://doi.org/10.5194/bg-6-2637-2009, 2009.
Lida, Y., Takatani, Y., Kojima, A., and Ishij, M.: Global trends of ocean CO2 sink and ocean acidification: an observation-based reconstruction of surface ocean inorganic carbon variables, J. Oceanogr., 77, 323–358, https://doi.org/10.1007/s10872-020-00571-5, 2021.
Lohbeck, K. T., Riebesell, U., and Reusch, T. B. H.: Adaptive evolution of a key phytoplankton species to ocean acidification, Nat. Geosci., 5, 346–351, https://doi.org/10.1038/ngeo1441, 2012.
López, A., Rico, M., Santana-Casiano, J. M., and González, A. G.: Phenolic profile of Dunaliella tertiolecta growing under high levels of copper and iron, Environ, Sci. Pollut. Res., 22, 14820–14828, https://doi.org/10.1007/s11356-015-4717-y, 2015.
Lund, P. A., De Biase, D., Liran, O., Scheler, O., Mira, N. P., Cetecioglu, Z., Fernández, E. N., Bover-Cid, S., Hall, R., Sauer, M., and O'Byrne, C.: Understanding How Microorganisms Respond to Acid pH Is Central to Their Control and Successful Exploitation, Front. Microbiol., 11, 556140, https://doi.org/10.3389/fmicb.2020.556140, 2020.
Lwalaba, J. L. W., Zvobgo, G., Mwamba, T. M., Louis, L. T., Fu, L., Kirika, B. A., Tshibangu, A. K., Adil, M. F., Sehar, S., Mukobo, R. P., and Zhang, G.: High accumulation of phenolics and amino acids confers tolerance to the combined stress of cobalt and copper in barley (Hordeum vulagare), Plant Physiol. Biochem., 155, 927–937, https://doi.org/10.1016/j.plaphy.2020.08.038, 2020.
Lu, X., Tian, C., Pei, H., Hu, W., and Xie, J.: Environmental factors influencing cyanobacteria community structure in Dongping Lake, China, J. Environ. Sci., 25, 2196–2206, https://doi.org/10.1016/S1001-0742(12)60297-6, 2013.
Mackey, K. R. M., Morris, J. J., Morel, F. M. M., and Kranz, S. A.: Response of photosynthesis to Ocean Acidification, Oceanography, 28, 74–91, http://www.jstor.org/stable/24861872 (last access: 1 October 2024), 2015.
Marinov, I., Doney, S. C., and Lima, I. D.: Response of ocean phytoplankton community structure to climate change over the 21st century: partitioning the effects of nutrients, temperature and light, Biogeosciences, 7, 3941–3959, https://doi.org/10.5194/bg-7-3941-2010, 2010.
Meier, K. J. S., Beaufort, L., Heussner, S., and Ziveri, P.: The role of ocean acidification in Emiliania huxleyi coccolith thinning in the Mediterranean Sea, Biogeosciences, 11, 2857–2869, https://doi.org/10.5194/bg-11-2857-2014, 2014.
Miller, C. A. and Kelley, A. L.: Alkalinity cycling and carbonate chemistry decoupling in seagrass mystify processes of acidification mitigation, Sci. Rep., 11, 13500, https://doi.org/10.1038/s41598-021-92771-2, 2021.
Millero, F. J., Graham, T. B., Huang, F., Bustos-Serrano, H., and Pierrot, D.: Dissociation constants of carbonic acid in seawater as a function of salinity and temperature, Mar. Chem., 100, 80–94, https://doi.org/10.1016/j.marchem.2005.12.001, 2006.
Millero, F. J., Woosley, R., Ditrolio, B., and Waters, J.: Effect of ocean acidification on the speciation of metals in seawater, Oceanography, 22, 72–85, https://doi.org/10.5670/oceanog.2009.98, 2009.
Mintrop, L., Perez, F. F., González Dávila, M., Körtzinger, A., and Santana-Casiano, J. M.: Alkalinity determination by potentiometry: intercalibration using three different methods, Cien. Mar., 26, 23–37, http://hdl.handle.net/10261/25136, 2000.
Morel, F. M. M. and Price, N. M.: The biogeochemical cycles of trace metals in the oceans, Science, 300, 944–947, https://www.science.org/doi/10.1126/science.1083545 (last access: 1 October 2024), 2003.
Nelson, K. S., Baltar, F., Lamare, M. D., and Morales, S. E.: Ocean acidification affects microbial community and invertebrate settlement on biofilms, Sci. Rep., 10, 3274, https://doi.org/10.1038/s41598-020-60023-4, 2020.
Parvin, K., Nahar, K., Mohsin, S. M., Al Mahmud, J., Fujita, M., and Hasanuzzaman, M.: Plant phenolic compounds for abiotic stress tolerance, in: Managing plant production under changing environment, edited by: Hasanuzzaman, M., Ahammed, G. J., and Nahar, K., Springer, Singapore, https://doi.org/10.1007/978-981-16-5059-8_8, 2022.
Pérez-Almeida, N., González, A. G., Santana-Casiano, J. M., and González-Dávila, M.: Iron and copper redox interactions in UV-seawater: A kinetic model approach, Chem. Geol., 506, 149–161, https://doi.org/10.1016/j.chemgeo.2018.12.041, 2019.
Pérez-Almeida, N., González, A. G., Santana-Casiano, J. M., and González-Dávila, M.: Ocean Acidification effect on the iron-gallic acid redox interaction in seawater, Front. Mar. Sci., 9, 837363, https://doi.org/10.3389/fmars.2022.837363, 2022.
Raven, J. A., Evans, M. C. W., and Korb, R. E.: The role of trace metals in photosynthetic electron transport in O2-evolving organisms, Photosyn. Res., 60, 111–149, https://doi.org/10.1023/A:1006282714942, 1999.
Rico, M., Santiago-Díaz, P., Rivero, A., and Santana-Casiano, J. M.: Characterization of polyphenols and carbohydrates exuded by Phaeodactylum tricornutum diatom grown under Cu stress, Sci. Rep., 14, 9367, https://doi.org/10.1038/s41598-024-60252-x, 2024.
Šamec, D., Karalija, E., Šola, I., Bok, V. V., and Salopek-Sond, B.: The role of polyphenols in abiotic stress response: the influence of molecular structure, Plants, 10, 118, https://doi.org/10.3390/plants10010118, 2021.
Samperio-Ramos, G., Santana-Casiano, J. M., González-Dávila, M., Ferreira, S., and Coimbra, M. A.: Variability in the organic ligands released by Emiliania huxleyi under simulated ocean acidification conditions, AIMS Environ. Sci., 4, 788–808, https://doi.org/10.3934/environsci.2017.6.788, 2017.
Santana-Casiano, J. M., González-Dávila, M., González, A. G., Rico, M., López, A., and Martel, A.: Characterization of phenolic exudates from Phaeodactylum tricornutum and their effects on the chemistry of Fe(II)–Fe(III), Mar. Chem., 158, 10–16, https://doi.org/10.1016/j.marchem.2013.11.001, 2014.
Santiago-Díaz, P., Rivero, A., Rico, M., González-González, A., González-Dávila, M., and Santana-Casiano, M.: Copper toxicity leads to accumulation of free amino acids and polyphenols in Phaeodactylum tricornutum diatoms, Environ. Sci. Pollut. R., 30, 51261–51270, https://doi.org/10.1007/s11356-023-25939-0, 2023.
Sethi, S., Joshi, A., Arora, B., Bhowmik, A., Sharma, R. R., and Kumar, P.: Significance of FRAP, DPPH, and CUPRAC assays for antioxidant activity determination in apple fruit extracts, Eur. Food Res. Technol., 246, 591–598, https://doi.org/10.1007/s00217-020-03432-z, 2020.
Shaked, Y., Buck, K. N., Mellett, T., and Maldonado, M. T.: Insights into the bioavailability of oceanic dissolved Fe from phytoplankton uptake kinetics, ISME J., 14, 1182–1193, https://doi.org/10.1038/s41396-020-0597-3, 2020.
Shay, J., Elbaz, H. A., Lee, I., Zielske, S. P., Malek, M. H., and Hüttemann, M.: Molecular mechanisms and therapeutic effects of (-)-Epicatechin and other polyphenols in cancer, inflammation, diabetes, and neurodegeneration, Oxid. Med. Cell. Longev., 2015, 181260, https://doi.org/10.1155/2015/181260, 2015.
Shi, D., Xu, Y., and Morel, F. M. M.: Effects of the pH/pCO2 control method on medium chemistry and phytoplankton growth, Biogeosciences, 6, 1199–1207, https://doi.org/10.5194/bg-6-1199-2009, 2009.
Singh, P. K. and Shrivastava, A. K.: Role of initial cell density of algal bioassay of toxic chemicals, J. Basic Microb., 56, 812–819, https://doi.org/10.1002/jobm.201500597, 2016.
Spisla, C., Taucher, J., Bach, L. T., Haunost, M., Boxhammer, T., King, A. L., Jenkins, B. D., Wallace, J. R., Ludwig, A., Meyer, J., Stange, P., Minutolo, F., Lohbeck, K. T., Nauendorf, A., Kalter, V., Lischka, S., Sswat, M., Dörner, I., Ismar-Rebitz, S. M. H., Aberle, N., Yong, J. C., Bouquet, J.-M., Lechtenbörger, A. K., Kohnert, P., Krudewig, M., and Riebesell, U.: Extreme levels of Ocean Acidification restructure the plankton community and biogeochemistry of a temperate coastal ecosystem: A mesocosm study, Front. Mar. Sci., 7, 611157, https://doi.org/10.3389/fmars.2020.611157, 2021.
Tagliabue, A., Bowie, A., Boyd, P., Buck, K. N., Johnson, K. S., and Saito, M. A.: The integral role of iron in ocean biogeochemistry, Nature, 543, 51–59, https://doi.org/10.1038/nature21058, 2017.
Tandon, P., Jin, Q., and Huang, L.: A promising approach to enhance microalgae productivity by exogenous supply of vitamins, Microb. Cell Fact., 16, 1–13, https://doi.org/10.1186/s12934-017-0834-2, 2017.
Thornton, D. C. O.: Effect of low pH on carbohydrate production by a marine planktonic diatom (Chaetoceros muelleri), Int. J. Ecol., 2009, 105901, https://doi.org/10.1155/2009/105901, 2009.
Trombetta, T., Vidussi, F., Mas, S., Parin, D., Simier, M., and Mostajir, B.: Water temperature drives phytoplankton blooms in coastal waters, PloS One, 14, 1–28, https://doi.org/10.1371/journal.pone.0214933, 2019.
Tong, S., Hutchins, D. A., and Gao, K.: Physiological and biochemical responses of Emiliania huxleyi to ocean acidification and warming are modulated by UV radiation, Biogeosciences, 16, 561–572, https://doi.org/10.5194/bg-16-561-2019, 2019.
Usher, P. K., Ross, A. B., Camargo-Valero, M. A., Tomlin, A. S., and Gale, W. F.: An overview of the potential environmental impacts of large-scale microalgae cultivation, Biofuels, 5, 331–349, https://doi.org/10.1080/17597269.2014.913925, 2014.
Vasconcelos, M. T. S. D., Leal, M. F. C., and van den Berg, C. M. G.: Influence of the nature of the exudates released by different marine algae on the growth, trace metal uptake and exudation of Emiliania huxleyi in natural seawater, Mar. Chem., 77, 187–210, https://doi.org/10.1016/S0304-4203(01)00087-1, 2002.
Vázquez, V., León, P., Gordillo, F. J. L., Jiménez, C., Concepción, I., Mackenzie, K., Bresnan, E., and Segovia, M.: HighCO2 levels rather than acidification restrict Emiliania huxleyi growth and performance, Microb. Ecol., 86, 127–143, https://doi.org/10.1007/s00248-022-02035-3, 2022.
Wang, D. L., Henrichs, S. M., and Guo, L. D.: Distributions of nutrients, dissolved organic carbon and carbohydrates in the western Arctic Ocean, Cont. Shelf Res., 26, 1654–1667, https://doi.org/10.1016/j.csr.2006.05.001, 2006.
Westbroek, P.: The coccolithophore Emiliania huxleyi and global climate, Paleontological Society Special Publications, 6, 309–309, https://doi.org/10.1017/S2475262200008698, 1992.
Wu, Y., Xiang, W., Fu, X., Yan, S., Su, J., Liu, J., and Bao, Z.: Geochemical interactions between iron and phenolics originated from peatland in Hani, China: implications for effective transport of iron from terrestrial systems to marine, Environ. Earth Sci., 75, 336, https://doi.org/10.1007/s12665-015-5189-6, 2016.
Xie, E., Xu, K., Li, Z., Li, W., Yi, X., Li, H., Han, Y., Zhang, H., and Zhang, Y.: Disentangling the effects of ocean carbonation and acidification on elemental contents and macromolecules of the Coccolithophore Emiliania Huxley, Front. Microbiol., 20, 12, https://doi.org/10.3389/fmicb.2021.737454, 2021.
Yu, J., Tian, J.-Y., Gao, G., Xu, R., Lai, J.-G., and Yang, G.-P.: Growth, DMS and DMSP production in Emiliania huxleyi under elevated CO2 and UV radiation, Environ. Pollut., 294, 118643, https://doi.org/10.1016/j.envpol.2021.118643, 2022.
Zhang, Y., Wu, H., Yuan, C., Li, T., and Li, A.: Growth, biochemical composition, and photosynthetic performance of Scenedesmus acuminatus during nitrogen starvation and resupply, J. Appl. Phycol., 31, 2797–2809, https://doi.org/10.1007/s10811-019-01783-z, 2019.