the Creative Commons Attribution 4.0 License.
the Creative Commons Attribution 4.0 License.
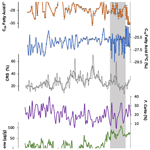
Exploring the use of compound-specific carbon isotopes as a palaeoproductivity proxy off the coast of Adélie Land, East Antarctica
Kate E. Ashley
Xavier Crosta
Johan Etourneau
Philippine Campagne
Harry Gilchrist
Uthmaan Ibraheem
Sarah E. Greene
Sabine Schmidt
Yvette Eley
Guillaume Massé
The Antarctic coastal zone is an area of high primary productivity, particularly within coastal polynyas, where large phytoplankton blooms and drawdown of CO2 occur. Reconstruction of historical primary productivity changes and the associated driving factors could provide baseline insights on the role of these areas as sinks for atmospheric CO2, especially in the context of projected changes in coastal Antarctic sea ice. Here we investigate the potential for using carbon isotopes (δ13C) of fatty acids in marine sediments as a proxy for primary productivity. We use a highly resolved sediment core from off the coast of Adélie Land spanning the last ∼ 400 years and monitor changes in the concentrations and δ13C of fatty acids along with other proxy data from the same core. We discuss the different possible drivers of their variability and argue that C24 fatty acid δ13C predominantly reflects phytoplankton productivity in open-water environments, while C18 fatty acid δ13C reflects productivity in the marginal ice zone. These new proxies have implications for better understanding carbon cycle dynamics in the Antarctica coastal zone in future palaeoclimate studies.
- Article
(1477 KB) - Full-text XML
-
Supplement
(442 KB) - BibTeX
- EndNote
Antarctic coastal zones are important players in the global carbon cycle. The deep ocean is ventilated in these regions as part of the Southern Ocean overturning circulation, allowing waters rich in nutrients and CO2 to be upwelled to the surface. In the absence of biological activity, most of the CO2 would be leaked to the atmosphere. However, coastal polynyas within the Antarctic margin are areas of very high primary productivity during the spring and summer months (e.g. Arrigo et al., 2008) that rapidly reduce CO2 to low levels through photosynthesis (Arrigo and van Dijken, 2003; Arrigo et al., 2008), resulting in surface water CO2 undersaturation with respect to atmospheric CO2 (Tortell et al., 2011). The subsequent export and burial of the organic carbon produced during these intense phytoplankton blooms can significantly lower atmospheric CO2 concentrations (Sigman and Boyle, 2000). Therefore, any change in the consumption of these nutrients by phytoplankton or any change in phytoplankton community structure may affect the air–sea CO2 exchange in this region.
Records of past phytoplankton productivity offer an opportunity to document the drivers of primary productivity at different timescales, from pluri-decadal to millennial. In the Antarctic coastal zone past work has focused on records of organic carbon, biogenic silica and diatom abundances (Ceccaroni et al., 1998; Frignani et al., 1998; Denis et al., 2009; Peck et al., 2015). These proxies however may provide a biased view of phytoplankton productivity as they only record a signal of siliceous productivity and may suffer from alteration during settling and burial (Beucher et al., 2004; Tréguer et al., 2017). As such, there is no robust understanding of how such records respond to surface water CO2, which is of major importance in the context of Antarctic coastal sea-ice changes.
Here we investigate the use of compound specific carbon isotope analysis (δ13C) of free (solvent extractable using ultrasonication), saturated algal fatty acids (FAs) in marine sediments as a potential integrative proxy for reconstructing primary productivity in a polynya environment. Fatty acids have the potential to be a useful palaeoproductivity tool in this region due to their ubiquitous presence within marine sediments, while other commonly used compounds, such as alkenones, are absent. Fatty acids are also able to persist within the sediments for several thousand years, meaning they have the potential to be applied over long time spans in contrast to more labile compounds such as highly branched isoprenoid alkenes (HBIs). Furthermore, fatty acids are amenable to isotope analysis allowing them to yield more detailed information about the environment.
Previous studies in the highly productive regions of the Southern Ocean have highlighted the potential for using compound-specific isotopes from algal biomarkers in sediments to track primary productivity changes both spatially and temporally. Villinski et al. (2008) found that the spatial variation in pCO2 in the Ross Sea was associated with a variation in the δ13C of sedimentary organic carbon and sterol biomarkers, most likely due to a change in isotopic fractionation associated with the photosynthetic drawdown of CO2. Their results demonstrate that the spatial variation in surface water CO2 is recorded in sedimentary organic matter and algal biomarkers. We explore this further as well as look into other potential drivers of compound-specific carbon isotopes.
We use samples from core DTGC2011, a 4.69 m sediment core recovered from offshore Adélie Land, East Antarctica, spanning the last ∼ 400 years. The core chronology is based on radiocarbon dates and confirmed by 210Pb excess activity measurements, which indicate that DTGC2011 spans the 1580–2000 CE period with a mean sedimentation rate of ∼ 1 cm yr−1 (Sect. S1 in the Supplement). In order to understand the signal recorded by the FAs, we estimate the most likely biological source of these compounds and the habitat and season of production. Moreover, we compare downcore changes in FA concentrations and δ13C with other proxy data from the same core.
Environmental setting
The Adélie drift is located in the Dumont D'Urville Trough in the Adélie Basin, ca. 35 km offshore from Adélie Land (Fig. 1). This is a 1000 m deep, glacially scoured depression on the East Antarctic continental shelf, bounded to the east by the Adélie Bank. Sea ice plays a key role in the dynamics of the region, with both fast ice and pack ice present off the coast of Adélie Land. A large bank of fast ice forms annually between 135 and 142∘ E and extends up to 120 km away from the coast (Massom et al., 2009). On the north edge of this fast-ice buttress is an inlet of open water forming a polynya, an area of open water surrounded by sea ice (Bindoff et al., 2000).
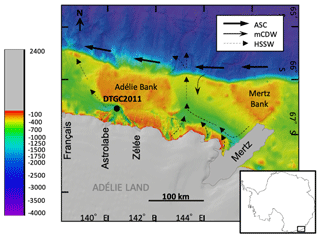
Figure 1Location of Site DTGC2011 on bathymetric map of the Adélie Land region (modified from Beaman et al., 2011), indicating positions of the main glaciers (prior to Mertz Glacier Tongue collapse in 2010) and pathways of the main water masses affecting the region: Antarctic Slope Current (ASC), Modified Circumpolar Deep Water (mCDW) and High-Salinity Shelf Water (HSSW) (Williams and Bindoff, 2003).
The Adélie Coast is characterised by extremely high primary productivity, with phytoplankton assemblages dominated by diatoms (Beans et al., 2008). The site itself is located close to the Dumont D'Urville polynya (DDUP), with an annual net primary productivity (NPP) of 30.3 g C m−2 yr−1, but is also directly downwind and downcurrent of the much larger and highly productive Mertz Glacier polynya (MGP) to the east, with an annual NPP of 39.9 g C m−2 yr−1 (Arrigo et al., 2015). Various factors are known to drive productivity trends in the Southern Ocean, including open-water area, glacial melt and mixed layer depth (Arrigo et al., 2015). In the MGP, Arrigo (2007) found light and nutrient availability to be the most important factors, which will in turn be modulated by changes in mixed layer depth, ice cover and glacial ice melt. Physiological differences in Phaeocystis antarctica compared to diatoms mean it can thrive in lower-nutrient conditions and with lower CO2 levels.
The region is affected by various water masses. High-Salinity Shelf Water (HSSW) is formed on the shelf in coastal polynyas as a result of sea-ice production and the associated brine rejection. HSSW flows out of the shelf through the Adélie sill at 143∘ E (Fig. 1). Modified Circumpolar Deep Water (mCDW) is a warm, macronutrient-rich and salty water mass which upwells onto the continental shelf through channels in the shelf break; mCDW has been observed to upwell across the shelf break near the Mertz Glacier at 144∘ E (Williams et al., 2008) (Fig. 1). The Antarctic Coastal Current, also known as the East Wind Drift, flows westward, often adjacent to ice shelves (Thompson et al., 2018). The Antarctic Surface Water (AASW) is a widespread water mass which extends across the continental shelf and has a surface mixed layer varying from a shallow (ca. 10 m), warmer and fresher layer in summer to a deeper (ca. 100 m), colder layer in winter. This is also transported westward along with the Antarctic Coastal Current (Martin et al., 2017). Surface waters along the Adélie coast have relatively high concentrations of nitrate, silica and phosphorus, with spatially variable levels of Fe, which may be due to re-suspension of sediments and calving of ice (Vaillancourt et al., 2003; Sambrotto et al., 2003).
2.1 Fatty acids
A total of 135 sediment samples were taken for organic geochemical analyses, sampled at 1 cm intervals in the top 50 cm, 2 cm intervals between 50 and 100 cm, and 5 cm intervals until 458 cm. Lipid extractions were completed at the University of Birmingham; 2 to 5 g of dried and homogenised sediment was extracted using 20 mL of dichloromethane : methanol (3 : 1 ) and ultrasonication. Specifically, samples were ultrasonicated for 20 min and then heated at ∼ 50 ∘C for 1 h, centrifuged and the supernatant pipetted off. The ultrasonication step and recovery of supernatant was repeated a further two times. The acid and neutral fractions were separated using an aminopropyl silica gel column and the FAs eluted using diethyl ether with 4 % acetic acid. The acid fraction was derivatised using boron trifluoride (14 % in methanol ()) and subsequently cleaned up using a silica gel column and the fatty acid methyl esters (FAMEs) eluted with dichloromethane.
FAs were identified using an Agilent 7890B gas chromatograph (GC) coupled to an Agilent 5977A mass selective detector (MSD) with a BP5-MS (SGE) column (60 m, 320 µm internal diameter, 0.25 µm film thickness). Helium was used as the carrier gas set at a constant flow rate of 2 mL min−1. The MSD was run in scan mode with a scan width of 50 to 800 mass units. Concentrations were quantified using an Agilent 7890B GC flame ionisation detector, using Hydrogen as the carrier gas with a constant flow rate of 2 mL min−1. An Rtx™-200 column (105 m, 250 µm internal diameter, 0.25 µm film thickness) which has a poly(trifluoropropylmethylsiloxane) stationary phase was used for FAME analyses to enable the best separation possible. The oven programme was 70 ∘C, held for 1 min, increased to 150 ∘C at a rate of 30 ∘C min−1, increased to 320 ∘C at a rate of 3 ∘C min−1, then held for 10 min. FAME concentrations were quantified by addition of a C19 alkane as an internal standard, prepared in-house to the concentration of 10 ng µL−1. The peak areas of FAMEs and the internal standard were used to calculate the concentration of each compound.
The δ13C composition of fatty acids are described in delta notation:
whereby the standard is Vienna Pee Dee Belemnite. Carbon isotopes were measured using an Agilent 7890A GC coupled to an Isoprime GC5 furnace and an Isoprime 100 isotope ratio mass spectrometer (IRMS). The Isoprime GC5 furnace contained a CuO furnace tube kept at 850 ∘C. Helium was used as the carrier gas set at a constant flow of 1.7 mL min−1, and CO2 was used as the reference gas. The GC had a VF-200ms column (60 m, 250 µm internal diameter, 0.25 µm film thickness), which also has a poly(trifluoropropylmethylsiloxane) stationary phase. The oven programme was 70 ∘C, held for 1 min, increased to 150 ∘C at a rate of 30 ∘C min−1, increased to 320 ∘C at a rate of 3 ∘C min−1, then held for 5 min. Most samples were run using an Agilent 7693 autosampler from dilutions of 10–100 µL. Where concentrations were very low, samples were dissolved in < 10 µL and were manually injected. Most samples were run in duplicate except for a few cases where the sample concentration was so low that the entire sample had to be injected in one run.
Machine performance was routinely checked using a FAME ester mix (F8; Indiana University) containing eight FAME compounds. This was run before the start of analysis and after every five duplicate samples. Errors are based on the difference between duplicate measures and are all within 0.26 ‰.
To correct for the additional carbon added during MeOH derivatisation, three FA standards were analysed for their bulk carbon isotope value using an Elementar PYRO cube at the University of Birmingham. Samples were combusted at 920 ∘C before being passed through a reduction column, and the isotopic composition of sample gases was determined on an isoprime continuous-flow mass spectrometer. These samples were then derivatised and then analysed on the GC–IRMS for the δ13C value of the FAME. The δ13C of the FA (δ13CFA) and FAME (δ13CFAME) was used to calculate the δ13C of the MeOH (δ13CMeOH) as follows:
whereby nFAME is the number of carbons in the FAME, and nFA is the number of carbons in the FA. δ13CMeOH was calculated to be ca. −40.8 ‰, and the δ13CFAME values were corrected using
2.2 HBIs
A total of 234 samples were taken every 2 cm over the whole core for highly branched isoprenoid (HBI) alkene analysis. HBIs were extracted at the Laboratoire d'Océanographie et du Climat: Experimentations et Approches Numériques (LOCEAN) separately from the fatty acids using a mixture of 9 mL CH2Cl2 MeOH (2 : 1, v:v); 7 hexyl nonadecane ( 266) was added as an internal standard during the first extraction steps, following the Belt et al. (2007) and Massé et al. (2011) protocols. Several sonication and centrifugation steps were applied in order to fully extract the selected compounds (Etourneau et al., 2013). After drying with N2 at 35 ∘C, the total lipid extract was fractionated over a silica column into an apolar and a polar fraction using 3 mL hexane and 6 mL CH2Cl2 MeOH (1 : 1, v:v), respectively. HBIs were obtained from the apolar fraction following the procedures reported by Belt et al. (2007) and Massé et al. (2011). After removing the solvent with N2 at 35 ∘C, elemental sulfur was removed using the TBA (tetrabutylammonium) sulfite method (Jensen et al., 1977; Riis and Babel, 1999). The obtained hydrocarbon fraction was analysed within an Agilent 7890A gas chromatograph (GC) fitted with 30 m fused silica Agilent J&C GC column (0.25 mm internal diameter, 0.25 µm film thickness), coupled to an Agilent 5975C Series mass selective detector (MSD). Spectra were collected using the Agilent MSD ChemStation software. Individual HBIs were identified on the basis of comparison between their GC retention times and mass spectra with those of previously authenticated HBIs (Johns et al., 1999) using the MassHunter software. Values are expressed as concentration relative to the internal standard.
2.3 Diatoms
A total of 118 samples were taken every 4 cm over the whole core for diatom analyses. Sediment processing and slide preparation followed the method described in Crosta et al. (2020). Diatom counting followed the rules described in Crosta and Koç (2007). Around 350 diatom valves were counted in each sample at a 1000× magnification on a Nikon Eclipse 80i phase contrast microscope. Diatoms were identified at the species or species group level. Absolute abundances of diatoms were calculated following the equation detailed in Crosta et al. (2008). The relative abundance of each species was determined as the fraction of diatom species against total diatom abundance in the sample.
Analysis by gas chromatography–mass spectrometry (GC–MS) identified seven dominant saturated FAs within the DTGC2011 samples (Fig. S2 in the Supplement). These have carbon chain lengths of C16 to C26, and only the saturated forms (i.e. no double bonds) were identified. These are predominantly even-chain-length FAs, with only minor amounts of the C17 compound measured (Gilchrist, 2018).
3.1 Fatty acid concentrations
The C19 alkane was used as an internal standard to aid quantification of fatty acid concentrations. However, it should be noted that since this standard was added to samples post-extraction, our concentration estimates are semi-quantitative but can be used to compare concentration changes in different FA compounds.
Downcore analysis of FA concentrations reveals clear groupings in concentration changes. In the upper part of the core (ca. 3–90 cm depth), spanning the last ∼ 78 years, all FA compounds show a similar pattern, with elevated concentrations broadly decreasing downcore (Fig. 2). Below this, however, two groups clearly diverge. These can be broadly divided into short-chained fatty acids (C16 to C20; SCFAs) and long-chained fatty acids (C22 to C26; LCFAs). Within these groups, the concentrations of different compounds show similar trends, but the two groups (SCFAs vs. LCFAs) show different trends to each other (Gilchrist, 2018). This is confirmed by R2 values calculated for the linear regression of concentrations of each FA against each other throughout the core (Fig. 3; n=135, p<0.001). Correlations between the SCFAs have R2 values between 0.97 and 0.99, while R2 values of LCFAs range between 0.88 and 0.95. Between the two groups, however, R2 values are all lower, ranging between 0.50 and 0.77.
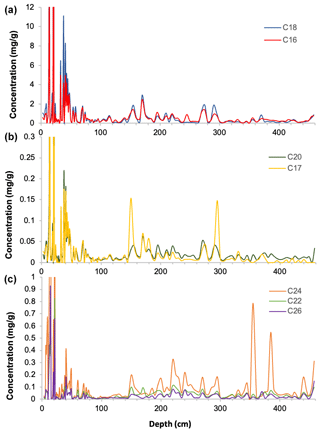
Figure 2Fatty acid concentrations (µg g−1 of dry sediment) with depth from core DTGC2011 (a) C16 and C18 fatty acids; (b) C17 and C20 fatty acids; and (c) C22, C24 and C26 fatty acids.
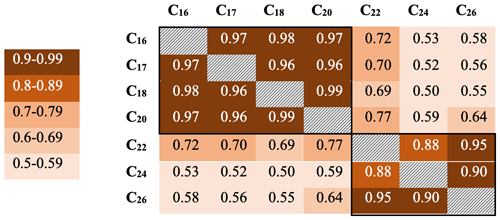
Figure 3R2 values for fatty acid concentrations throughout core DTGC2011. Values are colour-coded according to the key on the left. The black border denotes correlations within each group.
These distinct groupings suggest that compounds within each group (SCFAs and LCFAs) likely have a common precursor organism or group of organisms, but the two groups themselves have different producers from each other. These producers may in turn thrive during different seasons or within different habitats, and thus the isotopic composition of compounds from these different groups may record different environmental signals.
R2 values were also calculated for samples below 25 cm only (ca. 1587–1978 CE) to remove correlations associated with preservation changes in the top part of the core (discussed below). Although the R2 values are not quite as high, they broadly confirm these groupings, with the R2 values generally being greater within the two groups (n=73). R2 values range from 0.93 for the C18 with C20, down to 0.07 for the C18 and C24 (Fig. 4).
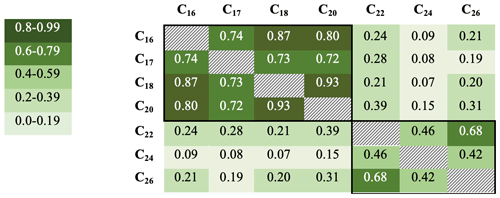
Figure 4R2 values for fatty acid concentrations in core DTGC2011 below 25 cm only. Values are colour-coded according to the key on the left. The black border denotes correlations within each group.
The C18 and C24 FAs are the most abundant compounds within the SCFA and LCFA groups, respectively, and also the least correlated with each other both in the whole core (R2=0.5) and below 25 cm (R2=0.07), which suggests that they are the most likely to be produced by different organisms. Furthermore, these two compounds yielded the highest-quality isotope measurements due to their greater concentrations, clean baseline and minimal coeluting peaks (Fig. S2). Thus, these two compounds (C18 and C24) are the focus of analysis and discussion.
3.2 Potential sources of the C18 fatty acid
Potential sources for the C18 FA in core U1357 (recovered from the same site as DTGC2011) are discussed in Ashley et al. (2021a), who suggest the prymnesiophyte Phaeocystis antarctica to be the most likely main producer. This is based on (a) previous studies of FAs produced by microalgae (Dalsgaard et al., 2003), (b) the high observed abundance of P. antarctica within modern Adélie surface waters (Riaux-Gobin et al., 2011; Sambrotto et al., 2003), and (c) comparison between the measured δ13C values and those reported in the literature for P. antarctica (Kopczynska et al., 1995; Wong and Sackett, 1978). Unfortunately, the absence of P. antarctica in sediments, as it does not biomineralise any test, precludes the direct comparison of downcore trends of this species with FAs. Phaeocystis antarctica has been found to live within and underneath sea ice before its break-up as well as in open ocean waters (Riaux-Gobin et al., 2013; Poulton et al., 2007) due to its ability to use a wide range of light intensities for energy production (Moisan and Mitchell, 1999).
Furthermore, Skerratt et al. (1998) compared the FAs produced by P. antarctica and two Antarctic diatoms, in culture samples, and showed that P. antarctica produced a much higher percentage of both saturated FAs (C14–C20) and C18 FAs than the diatoms. This supports the hypothesis of P. antarctica being a dominant and abundant source of the saturated C18 FA in the Adélie basin, though minor contributions of C18 from other phytoplankton species such as the diatoms and dinoflagellates or even bacteria cannot be excluded (Table S2).
3.3 Potential sources of the C24 fatty acid
Long-chain n-alkyl compounds, including FAs, are major components of vascular plant waxes, and their presence within sediments has commonly been used as a biomarker of terrestrial plants (Pancost and Boot, 2004). Although plants such as bryophytes (e.g. mosses), which are present in the Antarctic, do also produce LCFAs (Salminen et al., 2018), it is unlikely that FAs from terrestrial plants make a significant contribution to the water column due to their extremely limited extent on the continent and the significant distance of the site from other continental sources.
However, there is much evidence in the literature for various aquatic sources of LCFAs, a few of which are summarised in Table S2. Although not all of these sources are likely to be present within the coastal waters offshore Adélie Land, it highlights the wide range of organisms which can produce these compounds and thus suggests that an autochthonous marine source is likely, especially considering the highly productive nature of this region.
3.4 Microbial degradation and diagenetic effects on fatty acid concentration
Both the C18 and C24 FAs show an overall decrease in concentrations downcore, with significantly higher concentrations in the top 80 cm (representing ∼ 70 years) compared to the rest of the core. Below this point, FA concentration variations are attenuated (Fig. 5).
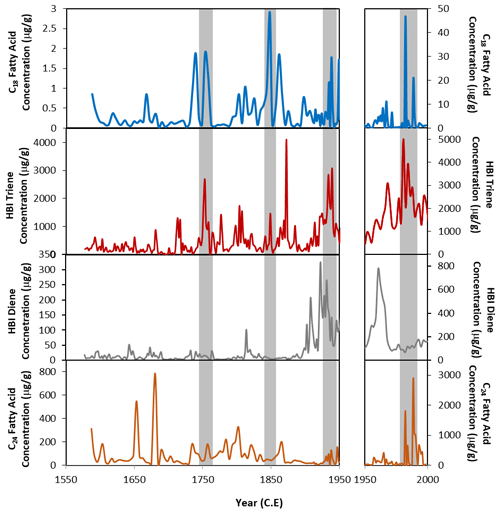
Figure 5Concentrations of the C18 fatty acid (blue), the HBI triene (red), HBI diene (grey) (Campagne, 2015) and C24 fatty acid (orange) from core DTGC2011. The left-hand panels show 1550 to 1950 CE, and the right-hand panels show 1950 to 2000 CE, plotted on different y axes due to the elevated concentrations in the top part of the core. Grey vertical bands highlight coincident peaks in C18 fatty acid and HBI triene records.
Many studies have shown that significant degradation of FAs occurs both within the water column and surface sediments as a result of microbial activity and that there is preferential break down of both short-chained and unsaturated FA compared to longer-chained and saturated FA (Haddad et al., 1992; Matsuda, 1978; Colombo et al., 1997). Haddad et al. (1992) studied the fate of FAs within rapidly accumulating (10.3 cm yr−1) coastal marine sediments (off the coast of North Carolina, USA) and showed that the vast majority (ca. 90 %) of saturated FAs were lost due to degradation within the top 100 cm (representing ∼ 10 years). Similarly, Matsuda and Koyama (1977) found that FA concentrations decrease rapidly within the top 20 cm of sediment (accumulating at 4 mm yr−1) from Lake Suwa, Japan. Assuming similar processes apply to the DTGC2011 sediments, this suggests that the declining concentrations within the upper part of the core are largely the result of diagenetic effects such as microbial activity occurring within the surface sediments and thus do not reflect a real change in production of these compounds in the surface waters.
The complete lack of both unsaturated and short-chained (fewer than 16 carbon atoms) FA compounds identified within DTGC2011 samples, even within the top layers, suggests that selective breakdown of compounds has already occurred within the water column and on the sea floor (before burial). Wakeham et al. (1984) assessed the loss of FAs with distance during their transport through the water column at a site in the equatorial Atlantic Ocean and estimated that only 0.4 % to 2 % of total FAs produced in the euphotic zone reached a depth of 389 m, with even less reaching more than 1000 m depth, the vast majority of material being recycled in the upper water column. Their results also show a significant preference for degradation of both unsaturated and short-chained compounds over saturated and longer chain length compounds. Although no studies on the fate of lipids within the water column exist for the Adélie region, the >1000 m water depth at the core site would provide a significant opportunity for these compounds to be broken down during transportation through the water column. It is likely, therefore, that the distribution of compounds preserved within the sediments will not be a direct reflection of production in the surface waters and explains the preference for saturated FAs with carbon chain lengths of 16 and more. It is also possible that some additional production and contribution of FAs by bacteria occurred during this process (Allen and Bartlett, 2002; Allen et al., 1999; Jónasdóttir, 2019).
Although FA concentrations in the top 80 cm of core DTGC2011 are much higher overall than the sediments below and show a broad decline over this section, there is a high level of variability. Concentrations do not decrease uniformly within the top part of the core, as may be expected if concentration change is a first-order response to declining microbial activity. The peak in total FAs instead occurs at a depth of 21–22 cm with a concentration more than an order of magnitude higher than in the top layer. This variability creates difficulty in directly determining the effects of diagenesis. However, by 25 cm (ca. 1978 CE) the concentrations drop to below 1000 ng g−1 and remain so until 32 cm before increasing again. This may suggest that diagenetic effects of FA concentrations are largely complete by 25 cm (representing ca. 25 years), consistent with results from Haddad et al. (1992) and Matsuda and Koyama (1977), and that subsequent downcore concentration variations predominantly represent real changes in export productivity, resulting from environmental factors. However, the fluctuating nature of concentrations, particularly in the youngest sediments, means it is difficult to clearly unpick the effects of diagenesis from actual changes in production of these compounds, and a clear cut-off point for diagenetic effects cannot be determined.
3.5 Comparison of fatty acid concentrations with highly branched isoprenoid alkenes
We compare FA concentrations with other organic compounds (whose source is better constrained) in DTGC2011 to better understand FA sources. Direct comparison between different organic compound classes can be made since both are susceptible to similar processes of diagenesis, in contrast to other proxies such as diatoms. In core DTGC2011, concentrations of di- and tri-unsaturated highly branched isoprenoid (HBI) alkenes (referred to as HBI diene and HBI triene, respectively, hereafter) were available.
In Antarctic marine sediments HBIs have been used as a tool for reconstructing sea ice (Belt et al., 2016, 2017). Smik et al. (2016) compared the concentrations of HBIs in sediment samples offshore East Antarctica from the permanently open-ocean zone (POOZ), the marginal ice zone (MIZ) and the summer sea-ice zone (SIZ). They found that the HBI diene reached the highest concentrations in the SIZ and was absent from the POOZ. In contrast, the HBI triene was most abundant in the MIZ, i.e. at the retreating sea-ice edge, with much lower concentrations in the SIZ and POOZ. This suggests that the two compounds are produced in contrasting environments but remain sensitive to changes in sea ice.
The HBI diene biomarker (or IPSO25 ice proxy for the Southern Ocean with 25 carbons) is mainly biosynthesised by Berkeleya adeliensis (Belt et al., 2016), a diatom which resides and blooms within the sea-ice matrix, and thus can be used as a proxy for fast ice attached to the coast. In contrast, the presence of the HBI triene mostly in the MIZ is suggestive of a predominantly pelagic phytoplankton source (e.g. Rhizosolenia spp.; Massé et al., 2011; Smik et al., 2016; Belt et al., 2017) rather than sea-ice dwelling diatoms (Smik et al., 2016). The fact that the HBI triene reached its greatest abundance within the MIZ suggests that its precursor organism may thrive in the stratified, nutrient-rich surface waters of the sea-ice edge.
One key similarity between both the HBI diene and triene and the FA concentrations is that the highest concentrations are found in the youngest sediments. These compounds all show broad increases in concentration from 110 cm depth (ca. 1900 CE) until the top of the core (Fig. 5). Concentrations of HBIs are also susceptible to degradation through the water column through visible-light-induced photo-degradation (Belt and Müller, 2013) and diagenetic effects within the sediments, including sulfurisation (Sinninghe Damsté et al., 2007), isomerisation and cyclisation (Belt et al., 2000). Thus, it is likely that the elevated concentrations and thus the similarity between FA and HBI concentrations are due to the material being fresher and thus less affected by diagenesis, with diagenetic effects having an increasing and progressive impact down to ca. 25 cm depth.
However, despite an overall increase in HBI and FA concentrations above 110 cm depth, there are clear deviations from this trend. Concentrations of the HBI triene show some broad similarities with FA concentrations. In particular, both the HBI triene and the C18 FA have coeval concentration peaks around 1980–1988, 1967, 1938, 1961–1972, 1848 and 1752 CE (Fig. 5). These peaks are offset from the HBI diene concentrations, suggesting that they result from increased production in the surface waters rather than simply changes in preservation. The HBI triene is more susceptible to degradation than the diene (Cabedo Sanz et al., 2016), so while this could explain some of the differences between the diene and triene records, where the triene increases independently of the diene, this is likely to be a genuine reflection of increased production of these compounds at the surface rather than an artefact of preservation processes.
This close similarity between the C18 FA and HBI triene concentrations (Fig. 5) suggests that the C18 may also be produced by an organism associated with the retreating ice edge. Phaeocystis antarctica has been proposed as a potential producer of the C18 in core U1357B (Ashley et al., 2021a). In the Ross Sea, P. antarctica has been observed to dominate the phytoplankton bloom during the spring, blooming in deep mixed layers as the sea ice begins to melt, after which diatoms tend to dominate during the summer (Arrigo et al., 1999; Tortell et al., 2011; DiTullio et al., 2000). However, a few studies in the Adélie region suggest that this is not the case there. Offshore Adélie Land, P. antarctica has been found to only appear late in the spring/early summer, later than many diatom species. During this time, it occurs preferentially within the platelet ice and under-ice water (Riaux-Gobin et al., 2013). Furthermore, Sambrotto et al. (2003) observed a surface bloom of P. antarctica near the Mertz Glacier (Fig. 1) during the summer months, in very stable waters along the margin of fast ice, and Riaux-Gobin et al. (2011) found P. antarctica to be abundant in the coastal surface waters 8 d after ice break-up. This indicates an ecological niche relationship with cold waters and ice melting conditions. This might explain the close similarity between the C18 and HBI triene concentrations, both produced by organisms occupying a similar habitat at the ice edge.
The C24 FA record also shows some similarity with the HBI triene record. This appears to be mostly in the top part of the core, where the highest concentrations are found. The reason for this resemblance is unclear, especially considering the lack of correlation between the C24 and C18 FA concentrations. However, it may relate to the progressive effect of diagenesis through the core. There is less similarity between the C24 and both the HBI triene and the HBI diene (compared to the coherence between C18 FA and the HBI triene), which suggests that the C24 FA is predominantly produced by an organism which is not associated with sea ice and thus instead with more open waters. A total of 73 diatom species were encountered in core DTGC2011 (Campagne, 2015), with Fragilariopsis curta and Chaetoceros resting spores being the most abundant. However, trends in diatom abundances do not show any clear correlations with the C18 or C24 FA concentrations. While this would lend support to the hypothesis that diatoms are not the main producers of these compounds, the differing effects of diagenesis on the preservation of diatoms and lipids could also explain some of the differences in observed concentrations, particularly in the upper part of the core. The known producer of the HBI diene, Berkeleya adeliensis, for example, was not recorded within the core, likely due to their lightly silicified frustules, which are more susceptible to dissolution (Belt et al., 2016). Therefore, despite the lack of a correlation between diatom abundances and FA concentrations, we cannot entirely rule out the possibility of a minor contribution of FAs by diatoms.
Downcore changes in δ13C for the C18 and C24 FAs (δ13C18FA and δ13C24FA, respectively) (Fig. 7) clearly show different trends, with very little similarity between them (R2=0.016). This further supports the idea that these compounds are being produced by different organisms and thus are recording different information.
The mean carbon isotope value of δ13C18FA of −29.8 ‰ in core U1357 from the same site (Ashley et al., 2021a) is suggestive of a pelagic phytoplankton source (Budge et al., 2008). In core DTGC2011 the mean values of δ13C18FA and δ13C24FA are −26.2 ‰ and −27.6 ‰, respectively. Though more positive, these values are still within the range of a phytoplankton source. Additionally, we tentatively suggest that the 0.5 ‰ more positive δ13C18FA mean value over the δ13C24FA may indicate the contribution of sea-ice-dwelling algae producers since carbon fixation occurring within the semi-closed system of the sea ice will lead to a higher degree of CO2 utilisation than in surrounding open waters (Henley et al., 2012). Although no studies on FA δ13C of different organisms are available for the Southern Ocean, Budge et al. (2008) measured the mean δ13C value of C16 FA from Arctic sea-ice algae (−24.0 ‰) to be 6.7 ‰ higher than pelagic phytoplankton (−30.7 ‰) from the same region.
The higher δ13C of the C18 FA could therefore be indicative of P. antarctica living partly within the sea ice, e.g. during early spring before ice break-up. The more negative δ13C24FA suggests that it is more likely to be produced by phytoplankton predominantly within open water.
4.1 Controls on δ13CFA
The δ13C18FA record shows a broadly increasing trend towards more positive values from ca. 1587 until ca. 1920 CE, with short-term fluctuations of up to ∼ 4 ‰ superimposed on this long-term trend (Fig. 7). This is followed by a period of higher variability with a full range of 5.6 ‰ until the most recent material (ca. 1999 CE), with more negative δ13C values between 1921 and 1977 CE and a rapid shift toward more positive values thereafter. In contrast, the δ13C24FA record overall shows a weak, negative trend, with large decadal fluctuations of up to 4.6 ‰, with a more pronounced negative trend after ca. 1880 CE (Fig. 7).
Below we consider the various factors which may control the carbon isotope value of algal biomarkers produced in the surface waters. Downcore changes in FA δ13C are likely to be a function of either the δ13C of the dissolved inorganic carbon (DIC) source, changes in the species producing the biomarkers, diagenesis or changing photosynthetic fractionation (εp). The next section outlines the potential influence these factors may have in order to assess the mostly likely dominant driver of FA δ13C.
4.1.1 Isotopic composition of DIC
The δ13C of the DIC source can be affected by upwelling or advection of different water masses or the δ13C of atmospheric CO2. Around the Antarctic, distinct water masses have unique carbon, hydrogen and oxygen isotope signatures, and thus isotopes can be used as water mass tracers (e.g. Mackensen, 2001; Archambeau et al., 1998). In the Weddell Sea for example, Mackensen (2001) determined the δ13C value of eight water masses, which ranged from 0.41 ‰ for Weddell Deep Water, sourced from Circumpolar Deep Water (CDW), to 1.63 ‰ for AASW. A similar range of ∼ 1.5 ‰ was identified in water masses between the surface and ∼ 5500 m depth along a transect from South Africa to the Antarctic coast (Archambeau et al., 1998). Assuming similar values apply to these water masses offshore Adélie Land, this range in values would be insufficient to explain the ∼ 5 ‰ variation in δ13C recorded by both C18 and C24 FA, even in the situation of a complete change in water mass over the core site. Furthermore, site DTGC2011, located within a 1000 m deep depression and bounded by the Adélie Bank to the north, is relatively sheltered from direct upwelling of deep water (Fig. 1). Though inflow of mCDW has been shown to occur within the Adélie Depression to the east of the bank (Williams and Bindoff, 2003) and possibly within the Dumont d'Urville Trough, only very small amplitude changes in δ13C of benthic foraminifera, tracking upper CDW, have been observed over the Holocene in Palmer Deep, West Antarctica (Shevenell and Kennett, 2002). Although from a different location, this argues against large changes in the isotopic composition of the source of mCDW.
Changes in the δ13C of atmospheric CO2, which is in exchange with the surface waters, could also have the potential to drive changes in the δ13C of algal biomarkers. Over the last ca. 200 years, the anthropogenic burning of fossil fuels has released a large amount of CO2 depleted in 13C, meaning that the δ13C of CO2 has decreased by ca. 1.5 ‰, as recorded in the Law Dome ice core. Prior to this, however, the δ13C of CO2 in the atmosphere remained relatively stable, at least for the last thousand years (Francey et al., 1999). Therefore, this could potentially drive the δ13C of algal biomarkers towards more negative values within the last 200 years, but this could not explain the full variation of ∼ 5 ‰–6 ‰ in FA δ13C measured throughout the core. Although the C24 δ13C shows a slight decrease over the last ca. 100 years, this is preceded by increasing δ13C, while the C18 δ13C displays no clear trend over the last 200 years. If atmospheric CO2 was a key driver of fatty acid δ13C, we would expect both compounds to respond together, showing a trend towards more negative values over the last 200 years, which neither of them do. This suggests that the effect of changing δ13C of atmospheric CO2 is insignificant compared to local and regional inter-annual variations as a result of other environmental drivers (discussed below).
4.1.2 Changing species
A shift in the organisms producing the FA could also affect δ13C where species have different fractionation factors. For example, changing diatom species have been shown to have an effect on bulk organic matter δ13C in core MD03-2601, offshore Adélie Land, over the last 5 kyr (Crosta et al., 2005). However, the bulk organic matter might have contained other phytoplankton groups than diatoms with drastically different δ13C values and fractionation factors. Here we measured δ13C of individual biomarkers, produced by a more restricted group of phytoplankton groups (possibly restricted to a few dominant species) compared to bulk δ13C. As discussed above, the C18 appears to be produced predominantly by P. antarctica, whereas diatoms do not tend to produce high proportions of this compound (Dalsgaard et al., 2003).
4.1.3 Effect of diagenesis on lipid δ13C
Sun et al. (2004) studied the carbon isotope composition of FAs during 100 d of incubation in both oxic and anoxic seawater. They observed a shift towards more positive values in FA δ13C, ranging between 2.6 ‰ for the C14 : 0 and as much as 6.9 ‰ in the C18 : 1, under anoxic conditions. This suggests that diagenesis could affect FA δ13C in core DTGC2011. However, these observed changes are rapid (days to months), occurring on timescales which are unresolvable in the FA δ13C record (annual to decadal), and thus may have no effect on the trends observed in our record. Based on concentration data discussed above, it seems that diagenetic overprint is largely complete by ∼ 25 cm (Fig. 5). In the top 25 cm of the core (ca. 1978–1998 CE), the δ13C24FA values increase by ∼ 2.5 ‰ downcore (R2=0.63, n=11), while the δ13C18FA values display a large variation with no overall trend (R2=0.12, n=20). If diagenesis was driving the changes in δ13C, it is likely that this trend would be observed in all FA compounds.
Taken together, it appears that neither changes in the δ13C of the DIC, changing phytoplankton groups nor diagenesis can fully explain the variation in FA δ13C recorded within DTGC2011. Therefore, we hypothesise that changes in εp are the main driver of FA δ13C.
4.2 Controls on photosynthetic fractionation (εp)
There is a positive relationship between εp in marine algae and dissolved surface water CO2(aq) concentration (Rau et al., 1989). As a result, higher δ13C values are hypothesised to reflect lower surface water CO2(aq) and vice versa. Popp et al. (1999) showed a strong negative correlation between CO2(aq) and δ13C of suspended particulate organic matter across a latitudinal transect in the Southern Ocean, suggesting that changes in surface water CO2(aq) can explain a large amount of the variation in δ13C. Changes in surface water CO2(aq) concentration in turn may be driven by various factors, including changing atmospheric CO2 (Fischer et al., 1997); wind-driven upwelling of deep, carbon-rich water masses (Sigman and Boyle, 2000; Takahashi et al., 2009); sea-ice cover (Henley et al., 2012); and/or primary productivity (Villinski et al., 2008). Thus, determining the main driver(s) of surface water CO2 changes offshore Adélie Land should enable interpretation of the DTGC2011 FA δ13C records.
4.2.1 Sea ice
Brine channels within sea ice have very low CO2 concentrations and a limited inflow of seawater. Carbon isotopic fractionation of algae living within these channels has been shown to be greatly reduced compared to organisms living in the surrounding open waters (Gibson et al., 1999), leading to elevated δ13C values. It is thus possible that, under conditions of high sea-ice cover, enhanced FA contribution from sea-ice algae leads to elevated sedimentary δ13C values. HBI diene concentrations within DTGC2011 show a much greater presence of fast ice at the core site in ca. 1960 CE (Fig. 5). However, during this time there is no clear elevation in δ13C concentrations in either δ13C18FA or δ13C24FA, both instead showing generally lower δ13C values. In fact, δ13C18FA shows the lowest values of the whole record between 1925 and 1974 CE, during which sea ice, as recorded by the HBI diene, is at its highest level. This suggests that inputs in sea-ice algae at this time are not driving changes in FA δ13C.
The DTGC2011 core site sits proximal to the Dumont D'Urville polynya, which has a summer area of 13.02×103 km2 and a winter area of 0.96×103 km2 (Arrigo and van Dijken, 2003). Changes in the size of the polynya both on seasonal and inter-annual timescales will affect air–sea CO2 exchange and thus also surface water CO2 concentration. A reduced polynya may lead to greater supersaturation of CO2 in the surface waters due to reduced outgassing, allowing CO2 to build up below the ice, leading to lower δ13C values of algal biomarkers produced in that habitat (Massé et al., 2011). Thus changes in the extent of sea ice may also affect FA δ13C.
4.2.2 Observed trends in surface water CO2(aq)
If the trend in surface water CO2(aq) paralleled atmospheric CO2, with an increase of over 100 ppm over the last 200 years (MacFarling Meure et al., 2006), we might expect phytoplankton to exert a greater fractionation during photosynthesis in response to elevated surface water CO2(aq) concentration, resulting in more negative δ13C values. Taking into account the decline in atmospheric δ13CO2 over the same period would further enhance the reduction in phytoplankton δ13C. Fischer et al. (1997) looked at the δ13C of both sinking matter and surface sediments in the South Atlantic and suggested that, since the preindustrial, surface water CO2(aq) has increased much more in the Southern Ocean than in the tropics. They estimated that a 70 ppm increase in CO2(aq) in surface waters of 1 ∘C would decrease phytoplankton δ13Corg by ca. 2.7 ‰, and changes of up to 3.3 ‰ δ13CO2 are included between preindustrial and 1977–1990. However, sea-ice cover and summer primary productivity are likely to be much higher off Adélie Land than in the South Atlantic, both of which will affect air–sea gas exchange.
Shadwick et al. (2014) suggest that surface water CO2 should track the atmosphere in the Mertz polynya region, despite the seasonal ice cover limiting the time for establishing equilibrium with the atmosphere. They calculated wintertime CO2 in the shelf waters of the Mertz polynya region, offshore Adélie Land (Fig. 1), measuring ca. 360 ppm in 1996, ca. 396 ppm in 1999 and ca. 385 ppm in 2007, while atmospheric CO2 at the South Pole was 360, 366 and 380 ppm, respectively (Keeling et al., 2005). Based on the 1996 and 2007 data only, an increase in CO2 of ca. 25 ppm is observed over these 11 years, coincident with the 20 ppm atmospheric CO2 increase over this time period. However, high inter-annual variability (± ca. 30 ppm) is evident (e.g. 396 ppm in 1999), suggesting that other factors, particularly upwelling, may override this trend. The latter was also suggested by Roden et al. (2013) based on winter surface water measurements in Prydz Bay, indicating that decadal-scale carbon cycle variability is nearly twice as large as the anthropogenic CO2 trend alone.
During the austral winter, upwelling of deep water masses causes CO2 to build up in the surface waters, and sea-ice cover limits gas exchange with the atmosphere (Arrigo et al., 2008; Shadwick et al., 2014). Although based on limited data, the measurements by Shadwick et al. (2014) suggest that slight supersaturation of up to 30 ppm occurs in the winter due to mixing with carbon-rich subsurface water but with high inter-annual variability. This is compared to undersaturation of 15 to 40 ppm during the summer as a result of biological drawdown of CO2. Roden et al. (2013) also observed varying levels of winter supersaturation in Prydz Bay, East Antarctica, with late winter CO2 values of 433 ppm in 2011 (45 µatm higher than atmospheric CO2), and suggested that intrusions of C-rich mCDW onto the shelf may play a part in this. Similarly, winter surface water CO2 of 425 ppm has been measured by Sweeney (2003) in the Ross Sea, before being drawn down to below 150 ppm in the summer as phytoplankton blooms develop.
Enhanced upwelling of deep carbon-rich waters in the Southern Ocean are thought to have played a key role in the deglacial rise in atmospheric CO2, increasing CO2 concentrations by ∼ 80 ppm (Anderson et al., 2009; Burke and Robinson, 2012). Changes in upwelling offshore Adélie Land could therefore drive some inter-annual variability in surface water CO2 and hence FA δ13C in DTGC2011. However, upwelling tends to be stronger during the winter months, when sea-ice formation and subsequent brine rejection drive mixing with deeper C-rich waters. At this time, heavy sea-ice cover limits air–sea gas exchange and enhances CO2 supersaturation in regional surface waters (Shadwick et al., 2014). In contrast, the phytoplankton producing FA thrive during the spring and summer months, during which CO2 is rapidly drawn down, and the surface waters become undersaturated. However, upwelling cannot be discarded as a possible contributor to surface water CO2 change. However, the core site is in a relatively sheltered area and is probably not affected by significant upwelling.
Based on these studies, changes in atmospheric CO2 concentration and δ13C of the source appear to be unlikely to be a dominant driver of the FA δ13C record, with inter-annual variations driven by other factors overriding any longer-term trend. There is also no clear anthropogenic decline in the FA δ13C record over the last 200 years, which supports this hypothesis.
4.2.3 Productivity
Given that changes in atmospheric CO2, source signal, sea-ice algae or diagenesis seem unable to explain the full range of variability seen in the FA δ13C record, the most plausible driver appears to be changes in surface water primary productivity. Coastal polynya environments in the Antarctic are areas of very high primary productivity (Arrigo and van Dijken, 2003). The DTGC2011 core site sits near the Dumont D'Urville polynya and is just downstream of the larger and more productive MGP (Arrigo and van Dijken, 2003). In large polynyas such as the Ross Sea, primary productivity leads to intense drawdown of CO2 in the surface waters, resulting in reduced fractionation by the phytoplankton during photosynthesis (Villinski et al., 2008). In the Ross Sea, surface water CO2 has been observed to drop to below 100 ppm during times of large phytoplankton blooms (Tortell et al., 2011), demonstrating that primary productivity can play a key role in controlling surface water CO2 concentrations in a productive polynya environment. Arrigo et al. (2015) found the MGP to be the eighth most productive polynya in the Antarctic (out of 46) based on total net primary productivity during their sampling period, and Shadwick et al. (2014) observed CO2 drawdown in the MGP during the summer months.
Therefore, we suggest that FA δ13C signals recorded in DTGC2011 are predominantly a signal of surface water CO2 driven by primary productivity. Indeed, the potential for the δ13C of sedimentary lipids to track surface water primary productivity has been recognised in the highly productive Ross Sea polynya. High variability in surface water CO2 values has been measured across the polynya during the summer months (December–January), ranging from less than 150 ppm in the western Ross Sea near the coast to > 400 ppm on the northern edge of the polynya. This pattern was closely correlated with diatom abundances, indicating intense drawdown of CO2 in the western region, where diatom abundances were highest (Tortell et al., 2011). This spatial variation in productivity is recorded in particulate organic carbon (POC) δ13C and is also tracked in the surface sediments by total organic carbon (TOC) δ13C and algal sterol δ13C, all of which show significantly higher values in the western Ross Sea. This spatial pattern in sterol δ13C was concluded to be directly related to CO2 drawdown at the surface, resulting in average sterol δ13C values varying from −27.9 ‰ in the west, where productivity is greatest, down to −33.5 ‰ farther offshore (Villinski et al., 2008).
A similar relationship is evident in Prydz Bay, where POC δ13C was found to be positively correlated with POC concentration and negatively correlated with nutrient concentration, indicating greater drawdown of CO2 and nutrients under high productivity levels (Zhang et al., 2014).
This suggests that it is possible to apply FA δ13C as a palaeoproductivity indicator in the highly productive Adélie polynya environment. However, it is important to constrain the most likely season and habitat being represented since phytoplankton assemblages vary both spatially (e.g. ice edge or open water) and temporally (e.g. spring or summer). The incredibly high sedimentation rate (1–2 cm yr−1) within the Adélie Basin is thought to result, on top of regional high productivity, from syndepositional focusing processes bringing biogenic debris from the shallower Adélie and Mertz banks to the ca. 1000 m deep basin (Escutia et al., 2011). Thus, it is likely that core DTGC2011 contains material from a wide area, including both the Mertz and Dumont d'Urville polynyas and areas both near the coast and farther offshore, meaning it is quite possible that the C18 and C24 FAs are integrating palaeoproductivity changes weighted towards different regional environments, which would explain their different trends. Furthermore, surface water CO2 can vary spatially, such as in the Ross Sea polynya, where Tortell et al. (2011) measured surface water CO2 values ranging between 100 and 400 ppm. Thus, it is likely that these two areas offshore Adélie Land, where the C18 and C24 FAs are being produced, will also have differing surface water CO2 concentrations and trends.
4.3 Comparison of fatty acid δ13C with other proxy data
Comparison of downcore variations in FA δ13C with other proxy data can also be used to decipher the main signal recorded. Comparison between δ13C24FA and the major diatom species abundances within the core (Fragilariopsis kerguelensis, Fragilariopsis curta, Fragilariopsis rhombica, Fragilariopsis cylindrus, Chaetoceros resting spores) shows a reasonably close coherence with Fragilariopsis kerguelensis, particularly since ∼ 1800 CE (Fig. 6). Fragilariopsis kerguelensis is an open-water diatom species and one of the most dominant phytoplankton species offshore Adélie Land (Chiba et al., 2000), reaching its peak abundance in the summer (Crosta et al., 2007). This suggests that the C24 FA is being produced during the summer months and, as such, is reflecting productivity in more open waters. The δ13C24FA record does not show any similarity to the sea-ice records, as inferred by HBI diene concentrations and abundances of Fragilariopsis curta (Figs. 6 and 7), here again suggesting that these compounds are being produced in open water during the summer months after sea ice has retreated.
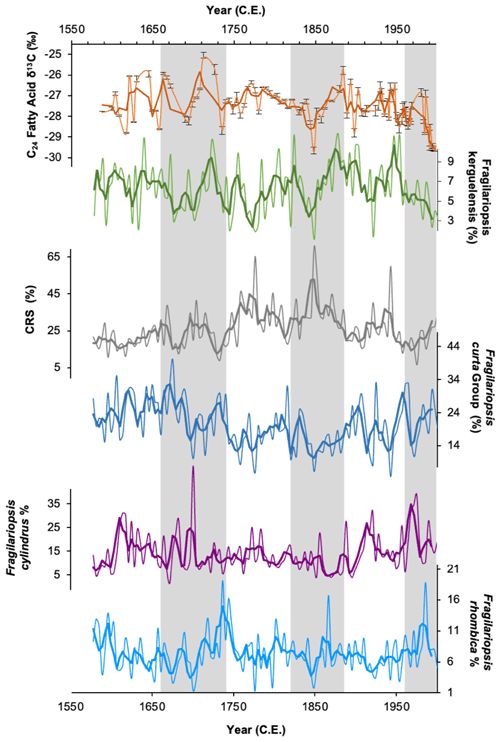
Figure 6δ13C values of the C24 fatty acid (orange) and relative abundances (%) of the open-water diatom Fragilariopsis kerguelensis (green). Also shown are relative abundances of the four most abundant diatom groups in DTGC2011. Chaetoceros resting spores (CRS; grey line), Fragilariopsis curta group (dark blue line), Fragilariopsis cylindrus (purple line) and Fragilariopsis rhombica (light-blue line). The thick line represents the three-point moving average for each. Grey vertical bands highlight periods where C24 fatty acid δ13C is in phase with F. kerguelensis.
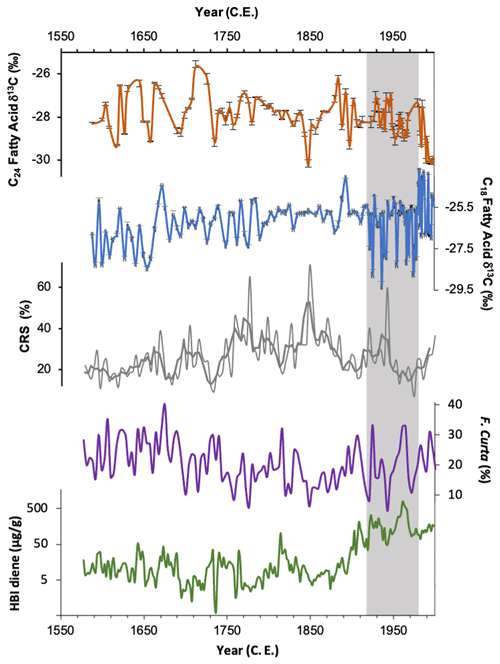
Figure 7δ13C of the C24 (orange) and C18 (blue) fatty acid, HBI diene concentrations (green; plotted on a log scale) and relative abundances of Fragilariopsis curta plus Fragilariopsis cylindrus (purple). The latter two records reflect sea-ice concentrations. The grey vertical band highlights the period where low C18 δ13C overlaps with elevated HBI diene concentrations.
As discussed above, P. antarctica is a likely producer for the C18 FA, a prymnesiophyte algae which has been observed in the Adélie region in summer months, residing predominantly along the margin of fast ice but also farther offshore (Riaux-Gobin et al., 2013, 2011; Vaillancourt et al., 2003). The aversion of F. kerguelensis to sea ice (and thus also the C24 FA producer) in contrast to P. antarctica may explain the clear lack of coherence in the downcore trends in δ13C18FA and δ13C24FA (Fig. 7). Thus, we hypothesise that δ13C18FA is recording surface water CO2 driven by productivity in the MIZ, whilst δ13C24FA is recording surface water CO2 in more open water, farther from the sea-ice edge.
HBI diene concentrations indicate elevated fast-ice cover between ∼ 1919 and 1970 CE, with a particular peak between 1942 and 1970 CE, after which concentrations rapidly decline and remain low until the top of the core (Fig. 7). Abundances of F. curta, used as a sea-ice proxy, similarly show peaks at this time, indicating increased sea-ice concentration (Campagne, 2015) (Fig. 7). δ13C18FA indicates a period of low productivity between ∼ 1922 and 1977 CE, broadly overlapping with this period of elevated fast-ice concentration (Fig. 7), with a mean value of −27.12 ‰. This is compared to the mean value of −26.23 ‰ in the subsequent period (∼ 1978 to 1998 CE), during which HBI diene concentration remains low (Fig. 7). This suggests that productivity in the coastal region was reduced, while sea-ice concentrations were high. This might be expected during a period of enhanced ice cover – perhaps representing a reduction in the amount of open water or a shorter open-water season – since the majority of productivity generally takes place within open water (Wilson et al., 1986).
Furthermore, δ13C18FA shows a broad similarity with Chaetoceros resting spores (CRSs) on a centennial scale, with lower productivity at the start of the record, ca. 1587 to 1662 CE, followed by an increase in both proxies in the middle part of the record, where δ13C18FA becomes relatively stable, and CRSs reaches their highest abundances of the record. This is then followed in the latter part of the record, after ca. 1900 CE, by both proxies displaying lower values overall. CRSs are associated with high nutrient levels and surface water stratification along the edge of receding sea ice, often following high productivity events (Crosta et al., 2008). The broad similarity to CRSs, with lower values recorded during periods of high sea-ice concentrations, suggests that δ13C18FA is similarly responding to productivity in stratified water at the ice edge. This supports the hypothesis that δ13C18FA is recording primary productivity in the MIZ. Little similarity is evident between the fatty acid isotope records and F. cylindrus and F. rhombica.
FAs identified within core DTGC2011, recovered from offshore Adélie Land, were analysed for their concentrations and carbon isotope compositions to assess their utility as a palaeoproductivity proxy in an Antarctic polynya environment. The C18 and C24 compounds yielded the best isotope measurements and show very different δ13C trends, suggesting that they are being produced by different species in different habitats and/or seasons. Although we have made parsimonious interpretations, there are clearly uncertainties in interpreting the FA δ13C, and as such various assumptions have been made. The primary producers of the C18 and especially the C24 FAs are a key source of uncertainty. Because these are general biomarkers, produced by many organisms, it is impossible to constrain entirely to one producer class. But with further work in the region, it could be possible to further elucidate the most likely contributors. The possibility of inputs of FAs from multiple sources, in particular from organisms farther up the food chain, has consequences for their interpretation since this could mean the δ13C FA is not fully reflecting just surface water conditions. Other key uncertainties are the magnitude of upwelling of CO2 at the site in comparison to drawdown by phytoplankton and the potential role of changes in air–sea CO2 exchange.
Despite this, we argue that FA δ13C has the potential to be used as a productivity proxy but would be best used in parallel with other environmental proxies such as diatom abundances or HBIs. Comparison with other proxy data and information from previous studies suggests that the C18 compound may be predominantly produced by P. antarctica, with δ13C18FA reflecting productivity changes in the marginal ice zone, where it is sensitive to changes in ice cover. In contrast, δ13C24FA, which compares well with abundances of the open-water diatom F. kerguelensis, may be reflecting summer productivity farther offshore, in open waters, where it is less sensitive to fast-ice changes. The use of δ13C analysis of multiple FA compounds as opposed to individual compounds or bulk isotope analysis allows a more detailed insight into the palaeoproductivity dynamics of the region, with the potential to separate productivity trends within different habitats.
Data are available in the Supplement and at the NOAA National Climatic Data Center (NCDC) database: https://www.ncei.noaa.gov/access/paleo-search/study/34232 (Ashley et al., 2021b).
The supplement related to this article is available online at: https://doi.org/10.5194/bg-18-5555-2021-supplement.
KEA wrote the paper with contributions and guidance from JB, XC, JE and SEG; KEA carried out the fatty acid molecular analyses (with support from UI and HG) and isotope analysis; PC and XC generated the diatom assemblage data; PC and GM generated the HBI analyses; SS performed the 210Pb analyses; XC and SS developed the age model. All authors contributed to the interpretations of data and finalisation of the manuscript.
The authors declare that they have no conflict of interest.
Publisher’s note: Copernicus Publications remains neutral with regard to jurisdictional claims in published maps and institutional affiliations.
We thank Sarah Feakins and four anonymous reviewers for comments and suggestions, which improved the manuscript. We thank Matt O'Callahan for technical support.
Core DTGC2011 was recovered during the expedition ALBION 2011 on board the R/V Astrolabe with the logistical support of the French Institut Paul Emile Victor (IPEV). The Natural Environment Research Council funded Kate E. Ashley (CENTA PhD NE/L002493/1). Sarah E. Greene was supported by NERC grant NE/L011050 while working on this paper. This research was also funded by the European Commission Seventh Framework Programme (ICEPROXY (ERC StG grant no. 203441)) and the ANR CLIMICE project. The CNRS (Centre National de la Recherche Scientifique) and the FRQNT (Fonds de recherche du Québec – Nature et technologies) provided Philippine Campagne with a fellowship.
This paper was edited by Markus Kienast and reviewed by Sarah Feakins and four anonymous referees.
Allen, E. E. and Bartlett, D. H.: Structure and regulation of the omega-3 polyunsaturated fatty acid synthase genes from the deep-sea bacterium Photobacterium profundum strain SS9The GenBank accession numbers for the sequences reported in this paper are AF409100 and AF467805, Microbiology, 148, 1903–1913, https://doi.org/10.1099/00221287-148-6-1903, 2002.
Allen, E. E., Facciotti, D., and Bartlett, D. H.: Monounsaturated but Not Polyunsaturated Fatty Acids Are Required for Growth of the Deep-Sea Bacterium Photobacterium profundum SS9 at High Pressure and Low Temperature, Appl. Environ. Microbiol., 65, 1710–1720, https://doi.org/10.1128/aem.65.4.1710-1720.1999, 1999.
Anderson, R. F., Ali, S., Bradtmiller, L. I., Nielsen, S. H. H., Fleisher, M. Q., Anderson, B. E., and Burckle, L. H.: Wind-Driven Upwelling in the Southern Ocean and the Deglacial Rise in Atmospheric CO2, Science, 323, 1443–1448, https://doi.org/10.1126/science.1167441, 2009.
Archambeau, A. S., Pierre, C., and Poisson, A.: Distributions of oxygen and carbon stable isotopes and CFC-12 in the water masses of the Southern Ocean at 30∘ E from South Africa to Antarctica: Results of the CIVA1 cruise, J. Marine Syst., 17, 25–38, https://doi.org/10.1016/S0924-7963(98)00027-X, 1998.
Arrigo, K. R.: Chapter 7 Physical Control of Primary Productivity in Arctic and Antarctic Polynyas, Elsevier Oceanography Series, 74, 223–238, https://doi.org/10.1016/S0422-9894(06)74007-7, 2007.
Arrigo, K. R. and van Dijken, G. L.: Phytoplankton dynamics within 37 Antarctic coastal polynya systems, J. Geophys. Res.-Oceans, 108, 3271, https://doi.org/10.1029/2002jc001739, 2003.
Arrigo, K. R., Dijken, G. L., and Strong, A. L.: Environmental controls of marine productivity hot spots around Antarctica, J. Geophys. Res.-Oceans, 118, 2121–2128, https://doi.org/10.1002/2015JC010888, 2015.
Arrigo, K. R., Robinson, D. H., Worthen, D. L., Dunbar, R. B., DiTullio, G. R., VanWoert, M., and Lizotte, M. P.: Phytoplankton community structure and the drawdown of nutrients and CO2 in the Southern Ocean, Science, 283, 365–367, https://doi.org/10.1126/science.283.5400.365, 1999.
Arrigo, K. R., van Dijken, G., and Long, M.: Coastal Southern Ocean: A strong anthropogenic CO2 sink, Geophys. Res. Lett., 35, 1–6, https://doi.org/10.1029/2008GL035624, 2008.
Ashley, K. E., McKay, R., Etourneau, J., Jimenez-Espejo, F. J., Condron, A., Albot, A., Crosta, X., Riesselman, C., Seki, O., Massé, G., Golledge, N. R., Gasson, E., Lowry, D. P., Barrand, N. E., Johnson, K., Bertler, N., Escutia, C., Dunbar, R., and Bendle, J. A.: Mid-Holocene Antarctic sea-ice increase driven by marine ice sheet retreat, Clim. Past, 17, 1–19, https://doi.org/10.5194/cp-17-1-2021, 2021a.
Ashley, K., Bendle, J. A., Crosta, X., Etourneau, J., Campagne, P., Gilchrist, H., Ibraheem, U., Greene, S., Schmidt, S., Eley, Y., and Massé, G.: Adélie Drift, East Antarctic Fatty Acid Carbon Isotope Data from 1587-1998 CE, NOAA [data set], available at: https://www.ncei.noaa.gov/access/paleo-search/study/34232, last access: 30 September 2021b.
Beaman, R. J., O’Brien, P. E., Post, A. L., and De Santis, L.: A new high-resolution bathymetry model for the Terre Adélie and George V continental margin, East Antarctica, Antarct. Sci., 23, 95–103, https://doi.org/10.1017/S095410201000074X, 2011.
Beans, C., Hecq, J. H., Koubbi, P., Vallet, C., Wright, S., and Goffart, A.: A study of the diatom-dominated microplankton summer assemblages in coastal waters from Terre Adelie to the Mertz Glacier, East Antarctica (139∘ E–145∘ E), Polar Biol., 31, 1101–1117, https://doi.org/10.1007/s00300-008-0452-x, 2008.
Belt, S. T. and Müller, J.: The Arctic sea ice biomarker IP25: a review of current understanding, recommendations for future research and applications in palaeo sea ice reconstructions, Quaternary Sci. Rev., 79, 9–25, https://doi.org/10.1016/j.quascirev.2012.12.001, 2013.
Belt, S. T., Allard, W. G., Rintatalo, J., Johns, L. A., van Duin, A. C. T., and Rowland, S. J.: Clay and acid catalysed isomerisation and cyclisation reactions of highly branched isoprenoid (HBI) alkenes: Implications for sedimentary reactions and distributions, Geochim. Cosmochim. Ac., 64, 3337–3345, https://doi.org/10.1016/s0016-7037(00)00444-0, 2000.
Belt, S. T., Massé, G., Rowland, S. J., Poulin, M., Michel, C., and LeBlanc, B.: A novel chemical fossil of palaeo sea ice: IP25, Org. Geochem., 38, 16–27, https://doi.org/10.1016/j.orggeochem.2006.09.013, 2007.
Belt, S. T., Smik, L., Brown, A., Kim, J. H., Rowland, S. J., Allen, C. S., Gal, J. K., Shin, K. H., Lee, J. I., and Taylor, K. W. R.: Source identification and distribution reveals the potential of the geochemical Antarctic sea ice proxy IPSO25, Nat. Commun., 7, 12655, https://doi.org/10.1038/ncomms12655, 2016.
Belt, S. T., Brown, T. A., Smik, L., Tatarek, A., Wiktor, J., Stowasser, G., Assmy, P., Allen, C. S., and Husum, K.: Identification of C-25 highly branched isoprenoid (HBI) alkenes in diatoms of the genus Rhizosolenia in polar and sub-polar marine phytoplankton, Org. Geochem., 110, 65–72, https://doi.org/10.1016/j.orggeochem.2017.05.007, 2017.
Beucher, C., Treguer, P., Hapette, A. M., Corvaisier, R., Metzl, N., and Pichon, J. J.: Intense summer Si-recycling in the surface Southern Ocean, Geophys. Res. Lett., 31, L09305, https://doi.org/10.1029/2003gl018998, 2004.
Bindoff, N., Rintoul, S., and Massom, R.: Bottom water formation and polynyas in Adelie Land, Antarctica, Papers and Proceedings of the Royal Society of Tasmania, 133, 51–56, https://doi.org/10.26749/rstpp.133.3.51, 2000.
Budge, S. M., Wooller, M. J., Springer, A. M., Iverson, S. J., McRoy, C. P., and Divoky, G. J.: Tracing carbon flow in an arctic marine food web using fatty acid-stable isotope analysis, Oecologia, 157, 117–129, https://doi.org/10.1007/s00442-008-1053-7, 2008.
Burke, A. and Robinson, L. F.: The Southern Ocean's Role in Carbon Exchange During the Last Deglaciation, Science, 335, 557–561, https://doi.org/10.1126/science.1208163, 2012.
Cabedo Sanz, P., Smik, L., and Belt, S. T.: On the stability of various highly branched isoprenoid (HBI) lipids in stored sediments and sediment extracts, Org. Geochem., 97, 74–77, https://doi.org/10.1016/j.orggeochem.2016.04.010, 2016.
Campagne, P.: Étude de la variabilité des conditions océanographiques et climatiques en Antarctique de l'Est (Terre Adélie-Georges V) au cours de l'Holocène tardif et de la période instrumentale, Université de Bordeaux, France, 2015.
Ceccaroni, L., Frank, M., Frignani, M., Langone, L., Ravaioli, M., and Mangini, A.: Late Quaternary fluctuations of biogenic component fluxes on the continental slope of the Ross Sea, Antarctica, J. Marine Syst., 17, 515–525, https://doi.org/10.1016/s0924-7963(98)00061-x, 1998.
Chiba, S., Hirawake, T., Ushio, S., Horimoto, N., Satoh, R., Nakajima, Y., Ishimaru, T., and Yamaguchi, Y.: An overview of the biological/oceanographic survey by the RTV Umitaka-Maru III off Adelie Land, Antarctica in January–February 1996, Deep-Sea Res. Pt. II, 47, 2589–2613, https://doi.org/10.1016/s0967-0645(00)00037-0, 2000.
Colombo, J. C., Silverberg, N., and Gearing, J. N.: Lipid biogeochemistry in the Laurentian Trough–ll. Changes in composition of fatty acids, sterols and aliphatic hydrocarbons during early diagenesis, Org. Geochem., 26, 257–274, 1997.
Crosta, X. and Koç, N.: Chapter Eight Diatoms: From Micropaleontology to Isotope Geochemistry, Dev. Mar. Geol., 1, 327–369, https://doi.org/10.1016/S1572-5480(07)01013-5, 2007.
Crosta, X., Crespin, J., Billy, I., and Ther, O.: Major factors controlling Holocene delta C-13(org) changes in a seasonal sea-ice environment, Adelie Land, East Antarctica, Global Biogeochem. Cy., 19, GB4029, https://doi.org/10.1029/2004gb002426, 2005.
Crosta, X., Debret, M., Denis, D., Courty, M. A., and Ther, O.: Holocene long- and short-term climate changes off Adelie Land, East Antarctica, Geochem. Geophy. Geosys., 8, Q11009, https://doi.org/10.1029/2007gc001718, 2007.
Crosta, X., Shukla, S. K., Ther, O., Ikehara, M., Yamane, M., and Yokoyama, Y.: Last Abundant Appearance Datum of Hemidiscus karstenii driven by climate change, Mar. Micropaleontol., 157, 101861, https://doi.org/10.1016/j.marmicro.2020.101861, 2020.
Dalsgaard, J., St John, M., Kattner, G., Muller-Navarra, D., and Hagen, W.: Fatty acid trophic markers in the pelagic marine environment, in: Advances in Marine Biology, Vol 46, edited by: Southwards, A. J., Tyler, P. A., Young, C. M., and Fuiman, L. A., Advances in Marine Biology, 225–340, https://doi.org/10.1016/s0065-2881(03)46005-7, 2003.
Denis, D., Crosta, X., Schmidt, S., Carson, D. S., Ganeshram, R. S., Renssen, H., Crespin, J., Ther, O., Billy, I., and Giraudeau, J.: Holocene productivity changes off Adélie land (East Antarctica), Paleoceanography, 24, 1–12, https://doi.org/10.1029/2008PA001689, 2009.
DiTullio, G. R., Grebmeier, J. M., Arrigo, K. R., Lizotte, M. P., Robinson, D. H., Leventer, A., Barry, J. B., VanWoert, M. L., and Dunbar, R. B.: Rapid and early export of Phaeocystis antarctica blooms in the Ross Sea, Antarctica, Nature, 404, 595–598, https://doi.org/10.1038/35007061, 2000.
Escutia, C., Brinkhuis, H., Klaus, A., and the Expedition 318 Scientists: Expedition 318 summary, Integrated Ocean Drilling Program Management International, Inc., for the Integrated Ocean Drilling Program, U.S. Implementing Organization Science Services, Texas A&M University, https://doi.org/10.2204/iodp.proc.318.101.2011, 2011.
Etourneau, J., Collins, L. G., Willmott, V., Kim, J.-H., Barbara, L., Leventer, A., Schouten, S., Sinninghe Damsté, J. S., Bianchini, A., Klein, V., Crosta, X., and Massé, G.: Holocene climate variations in the western Antarctic Peninsula: evidence for sea ice extent predominantly controlled by changes in insolation and ENSO variability, Clim. Past, 9, 1431–1446, https://doi.org/10.5194/cp-9-1431-2013, 2013.
Fischer, G., Schneider, R., Muller, P. J., and Wefer, G.: Anthropogenic CO2 in Southern Ocean surface waters: evidence from stable organic carbon isotopes, Terra Nova, 9, 153–157, https://doi.org/10.1046/j.1365-3121.1997.d01-29.x, 1997.
Francey, R. J., Allison, C. E., Etheridge, D. M., Trudinger, C. M., Enting, I. G., Leuenberger, M., Langenfelds, R. L., Michel, E., and Steele, L. P.: A 1000-year high precision record of delta C-13 in atmospheric CO2, Tellus B, 51, 170–193, https://doi.org/10.1034/j.1600-0889.1999.t01-1-00005.x, 1999.
Frignani, M., Giglio, F., Langone, L., Ravaioli, M., and Mangini, A.: Late Pleistocene-Holocene sedimentary fluxes of organic carbon and biogenic silica in the northwestern Ross Sea, Antarctica, Ann. Glaciol., 27, 697–703, 1998.
Gibson, J. A. E., Trull, T., Nichols, P. D., Summons, R. E., and McMinn, A.: Sedimentation of C-13-rich organic matter from Antarctic sea-ice algae: A potential indicator of past sea-ice extent, Geology, 27, 331–334, https://doi.org/10.1130/0091-7613(1999)027<0331:Socrom>2.3.Co;2, 1999.
Gilchrist, H.: A high-resolution record of sea ice, glacial and biological dynamics from an Antarctic coast environment, Masters Thesis, School of Geography, Earth and Environmental University of Birmingham, 2018.
Haddad, R. I., Martens, C. S., and Farrington, J. W.: Quantifying Early Diagenesis of Fatty-Acids in a Rapidly Accumulating Coastal Marine Sediment, Org. Geochem., 19, 205–216, https://doi.org/10.1016/0146-6380(92)90037-x, 1992.
Henley, S. F., Annett, A. L., Ganeshram, R. S., Carson, D. S., Weston, K., Crosta, X., Tait, A., Dougans, J., Fallick, A. E., and Clarke, A.: Factors influencing the stable carbon isotopic composition of suspended and sinking organic matter in the coastal Antarctic sea ice environment, Biogeosciences, 9, 1137–1157, https://doi.org/10.5194/bg-9-1137-2012, 2012.
Jensen, S., Renberg, L., and Reutergårdh, L.: Residue Analysis of Sediment and Sewage Sludge for Organochlorines in the Presence of Elemental Sulfur, Ana. Chem., 49, 316–318, https://doi.org/10.1021/ac50010a033, 1977.
Johns, L., Wraige, E. J., Belt, S. T., Lewis, C. A., Masse, G., Robert, J. M., and Rowland, S. J.: Identification of a C-25 highly branched isoprenoid (HBI) diene in Antarctic sediments, Antarctic sea-ice diatoms and cultured diatoms, Org. Geochem., 30, 1471–1475, https://doi.org/10.1016/s0146-6380(99)00112-6, 1999.
Jónasdóttir, S. H.: Fatty Acid Profiles and Production in Marine Phytoplankton, Mar. Drugs, 17, 151, https://doi.org/10.3390/md17030151, 2019.
Keeling, C. D., Piper, S. C., Bacastow, R. B., Wahlen, M., Whorf, T. P., Heimann, M., and Meijer, H. A., Ehleringer, J. R., Cerling, T., and Dearing, M. D. (Eds.): Atmospheric CO2 and 13CO2 Exchange with the Terrestrial Biosphere and Oceans from 1978 to 2000: Observations and Carbon Cycle Implications, A History of Atmospheric CO2 and Its Effects on Plants, Animals, and Ecosystems, Springer, https://doi.org/10.1007/0-387-27048-5_5, 2005.
Kopczynska, E. E., Goeyens, L., Semeneh, M., and Dehairs, F.: Phytoplankton Composition and Cell Carbon Distribution in Prydz Bay, Antarctica – Relation to Organic Particulate Matter and its Delta-C-13 Values, J. Plankton Res., 17, 685–707, https://doi.org/10.1093/plankt/17.4.685, 1995.
MacFarling Meure, C., Etheridge, D., Trudinger, C., Steele, P., Langenfelds, R., Van Ommen, T., Smith, A., and Elkins, J.: Law Dome CO2, CH4 and N2O ice core records extended to 2000 years BP, Geophys. Res. Lett., 33, 1–4, https://doi.org/10.1029/2006GL026152, 2006.
Mackensen, A.: Oxygen and carbon stable isotope tracers of Weddell Sea water masses: new data and some paleoceanographic implications, Deep-Sea Res. Pt. I, 48, 1401–1422, https://doi.org/10.1016/s0967-0637(00)00093-5, 2001.
Martin, A., Houssais, M., Le Geoff, H., Marec, C., and Dausse, D.: Circulation and water mass transports on the East Antarctic shelf in the Mertz Glacier region, Deep-Sea Res. Pt. I, 126, 1–20, https://doi.org/10.1016/j.dsr.2017.05.007, 2017.
Massé, G., Belt, S. T., Crosta, X., Schmidt, S., Snape, I., Thomas, D. N., and Rowland, S. J.: Highly branched isoprenoids as proxies for variable sea ice conditions in the Southern Ocean, Antarct. Sci., 23, 487–498, https://doi.org/10.1017/s0954102011000381, 2011.
Matsuda, H.: Early Diagenesis of Fatty-Acids in Lacustrine Sediments .3. Changes in Fatty-Acid Composition in Sediments from a Brackish Water Lake, Geochim. Cosmochim. Ac., 42, 1027–1034, https://doi.org/10.1016/0016-7037(78)90291-0, 1978.
Matsuda, H. and Koyama, T.: Early Diagenesis of Fatty-Acids in Lacustrine Sediment .1. Identification and Distribution of Fatty-Acids in Recent Sediment from a Freshwater Lake, Geochim. Cosmochim. Ac., 41, 777–783, https://doi.org/10.1016/0016-7037(77)90048-5, 1977.
Moisan, T. A. and Mitchell, B. G.: Photophysiological acclimation of Phaeocystis antarctica Karsten under light limitation, Limnol. Oceanogr., 44, 247–258, https://doi.org/10.4319/lo.1999.44.2.0247, 1999.
Pancost, R. D. and Boot, C. S.: The palaeoclimatic utility of terrestrial biomarkers in marine sediments, Mar. Chem., 92, 239–261, https://doi.org/10.1016/j.marchem.2004.06.029, 2004.
Peck, V. L., Allen, C. S., Kender, S., McClymont, E. L., and Hodgson, D.: Oceanographic variability on the West Antarctic Peninsula during the Holocene and the influence of upper circumpolar deep water, Quaternary Sci. Rev., 119, 54–65, https://doi.org/10.1016/j.quascirev.2015.04.002, 2015.
Popp, B. N., Trull, T., Kenig, F., Wakeham, S. G., Rust, T. M., Tilbrook, B., Griffiths, F. B., Wright, S. W., Marchant, H. J., Bidigare, R. R., and Laws, E. A.: Controls on the carbon isotopic composition of Southern Ocean phytoplankton, Global Biogeochem. Cy., 13, 827–843, https://doi.org/10.1029/1999gb900041, 1999.
Poulton, A. J., Moore, C. M., Seeyave, S., Lucas, M. I., Fielding, S., and Ward, P.: Phytoplankton community composition around the Crozet Plateau, with emphasis on diatoms and Phaeocystis, Deep-Sea Res. Pt. II, 54, 2085–2105, https://doi.org/10.1016/j.dsr2.2007.06.005, 2007.
Rau, G. H., Takahashi, T., and Marais, D. J. D.: Latitudinal Variations in Plankton Delta-C-13 – Implications for CO2 and Productivity in Past Oceans, Nature, 341, 516–518, https://doi.org/10.1038/341516a0, 1989.
Riaux-Gobin, C., Poulin, M., Dieckmann, G., Labrune, C., and Vetion, G.: Spring phytoplankton onset after the ice break-up and sea-ice signature (Adelie Land, East Antarctica), Polar Res., 30, 5910, https://doi.org/10.3402/polar.v30i0.5910, 2011.
Riaux-Gobin, C., Dieckmann, G. S., Poulin, M., Neveux, J., Labrune, C., and Vetion, G.: Environmental conditions, particle flux and sympagic microalgal succession in spring before the sea-ice break-up in Adelie Land, East Antarctica, Polar Res., 32, 19675, https://doi.org/10.3402/polar.v32i0.19675, 2013.
Riis, V. and Babel, W.: Removal of sulfur interfering in the analysis of organochlorines by GC-ECD, Analyst, 124, 1771–1773, https://doi.org/10.1039/a907504f, 1999.
Roden, N. P., Shadwick, E. H., Tilbrook, B., and Trull, T. W.: Annual cycle of carbonate chemistry and decadal change in coastal Prydz Bay, East Antarctica, Mar. Chem., 155, 135–147, https://doi.org/10.1016/j.marchem.2013.06.006, 2013.
Salminen, T. A., Eklund, D. M., Joly, V., Blomqvist, K., Matton, D. P., and Edqvist, J.: Deciphering the Evolution and Development of the Cuticle by Studying Lipid Transfer Proteins in Mosses and Liverworts, Plants-Basel, 7, 6, https://doi.org/10.3390/plants7010006, 2018.
Sambrotto, R. N., Matsuda, A., Vaillancourt, R., Brown, M., Langdon, C., Jacobs, S. S., and Measures, C.: Summer plankton production and nutrient consumption patterns in the Mertz Glacier Region of East Antarctica, Deep-Sea Res. Pt. II, 50, 1393–1414, https://doi.org/10.1016/S0967-0645(03)00076-6, 2003.
Shadwick, E. H., Tilbrook, B., and Williams, G. D.: Carbonate chemistry in the Mertz Polynya (East Antarctica): Biological and physical modification of dense water outflows and the export of anthropogenic CO2, J. Geophys. Res.-Oceans, 119, 1–14, https://doi.org/10.1002/2013jc009286, 2014.
Shevenell, A. E. and Kennett, J. P.: Antarctic Holocene climate change: A benthic foraminiferal stable isotope record from Palmer Deep, Paleoceanography, 17, PAL 9-1–PAL 9-12, https://doi.org/10.1029/2000pa000596, 2002.
Sigman, D. M. and Boyle, E. A.: Glacial/interglacial variations in atmospheric carbon dioxide, Nature, 407, 859–869, https://doi.org/10.1038/35038000, 2000.
Sinninghe Damsté, J. S., Rijpstra, W. I. C., Coolen, M. J. L., Schouten, S., and Volkman, J. K.: Rapid sulfurisation of highly branched isoprenoid (HBI) alkenes in sulfidic Holocene sediments from Ellis Fjord, Antarctica, Org. Geochem., 38, 128–139, https://doi.org/10.1016/j.orggeochem.2006.08.003, 2007.
Skerratt, J. H., Davidson, A. D., Nichols, P. D., and McMeekin, T. A.: Effect of UV-B on lipid content of three Antarctic marine phytoplankton, Phytochemistry, 49, 999–1007, https://doi.org/10.1016/s0031-9422(97)01068-6, 1998.
Smik, L., Belt, S. T., Lieser, J. L., Armand, L. K., and Leventer, A.: Distributions of highly branched isoprenoid alkenes and other algal lipids in surface waters from East Antarctica: Further insights for biomarker-based paleo sea-ice reconstruction, Org. Geochem., 95, 71–80, https://doi.org/10.1016/j.orggeochem.2016.02.011, 2016.
Sun, M. Y., Zou, L., Dai, J. H., Ding, H. B., Culp, R. A., and Scranton, M. I.: Molecular carbon isotopic fractionation of algal lipids during decomposition in natural oxic and anoxic seawaters, Org. Geochem., 35, 895–908, https://doi.org/10.1016/j.orggeochem.2004.04.001, 2004.
Sweeney, C.: The annual cycle of surface water CO2 And O2 in the Ross Sea: A model for gas exchange on the continental shelves of Antarctica, Antarct. Res. Ser., 78, https://doi.org/10.1029/078ars19, 2003.
Takahashi, T., Sutherland, S. C., Wanninkhof, R., Sweeney, C., Feely, R. A., Chipman, D. W., Hales, B., Friederich, G., Chavez, F., Sabine, C., Watson, A., Bakker, D. C. E., Schuster, U., Metzl, N., Yoshikawa-Inoue, H., Ishii, M., Midorikawa, T., Nojiri, Y., Kortzinger, A., Steinhoff, T., Hoppema, M., Olafsson, J., Arnarson, T. S., Tilbrook, B., Johannessen, T., Olsen, A., Bellerby, R., Wong, C. S., Delille, B., Bates, N. R., and de Baar, H. J. W.: Climatological mean and decadal change in surface ocean pCO(2), and net sea-air CO2 flux over the global oceans (vol 56, pg 554, 2009), Deep-Sea Res. Pt. I, 56, 2075–2076, https://doi.org/10.1016/j.dsr.2009.07.007, 2009.
Thompson, A. F., Stewart, A. L., Spence, P., and Heywood, K. J.: The Antarctic Slope Current in a Changing Climate, Rev. Geophys., 56, 741–770, https://doi.org/10.1029/2018RG000624, 2018.
Tortell, P. D., Gueguen, C., Long, M. C., Payne, C. D., Lee, P., and DiTullio, G. R.: Spatial variability and temporal dynamics of surface water pCO(2), Delta O-2/Ar and dimethylsulfide in the Ross Sea, Antarctica, Deep-Sea Res. Pt. I, 58, 241–259, https://doi.org/10.1016/j.dsr.2010.12.006, 2011.
Tréguer, P., Bowler, C., Moriceau, B., Dutkiewicz, S., Gehlen, M., Aumont, O., Bittner, L., Dugdale, R., Finkel, Z., Iudicone, D., Jahn, O., Guidi, L., Lasbleiz, M., Leblanc, K., Levy, M., and Pondaven, P.: Influence of diatom diversity on the ocean biological carbon pump, Nat. Geosci., 11, 27–37, https://doi.org/10.1038/s41561-017-0028-x, 2017.
Vaillancourt, R. D., Sambrotto, R. N., Green, S., and Matsuda, A.: Phytoplankton biomass and photosynthetic competency in the summertime Mertz Glacier Region of East Antarctica, Deep-Sea Res. Pt. II, 50, 1415–1440, https://doi.org/10.1016/s0967-0645(03)00077-8, 2003.
Villinski, J. C., Hayes, J. M., Brassell, S. C., Riggert, V. L., and Dunbar, R. B.: Sedimentary sterols as biogeochemical indicators in the Southern Ocean, Org. Geochem., 39, 567–588, https://doi.org/10.1016/j.orggeochem.2008.01.009, 2008.
Wakeham, S. G., Lee, C., Farrington, J. W., and Gagosian, R. B.: Biogeochemistry of Particulate Organic-Matter in the Oceans – Results from Sediment Trap Experiments, Deep-Sea Res. Pt. A, 31, 509–528, https://doi.org/10.1016/0198-0149(84)90099-2, 1984.
Williams, G. D. and Bindoff, N. L.: Wintertime oceanography of the Adelie Depression, Deep-Sea Res. Pt. II, 50, 1373–1392, https://doi.org/10.1016/s0967-0645(03)00074-2, 2003.
Williams, G. D., Bindoff, N. L., Marsland, S. J., and Rintoul, S. R.: Formation and export of dense shelf water from the Adélie depression, East Antarctica, J. Geophys. Res.-Oceans, 113, 1–12, https://doi.org/10.1029/2007JC004346, 2008.
Wilson, D. L., Smith, W. O., and Nelson, D. M.: Phytoplankton Bloom Dynamics of the Western Ross Sea Ice Edge .1. Primary Productivity and Species-Specific Production, Deep-Sea Res. Pt. A, 33, 1375–1387, https://doi.org/10.1016/0198-0149(86)90041-5, 1986.
Wong, W. W. and Sackett, W. M.: Fractionation of Stable Carbon Isotopes by Marine-Phytoplankton, Geochim. Cosmochim. Ac., 42, 1809–1815, https://doi.org/10.1016/0016-7037(78)90236-3, 1978.
Zhang, R., Zheng, M. F., Chen, M., Ma, Q., Cao, J. P., and Qiu, Y. S.: An isotopic perspective on the correlation of surface ocean carbon dynamics and sea ice melting in Prydz Bay (Antarctica) during austral summer, Deep-Sea Res. Pt. I, 83, 24–33, https://doi.org/10.1016/j.dsr.2013.08.006, 2014.