the Creative Commons Attribution 4.0 License.
the Creative Commons Attribution 4.0 License.
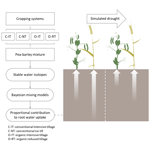
Water uptake patterns of pea and barley responded to drought but not to cropping systems
Valentin H. Klaus
Raphaël Wittwer
Yujie Liu
Marcel G. A. van der Heijden
Anna K. Gilgen
Nina Buchmann
Agricultural production is under threat of water scarcity due to increasingly frequent and severe drought events under climate change. Whether a change in cropping systems can be used as an effective adaptation strategy against drought is still unclear. We investigated how plant water uptake patterns of a field-grown pea–barley (Pisum sativum L. and Hordeum vulgare L.) mixture, an important fodder intercrop, responded to experimental drought under four cropping systems, i.e. organic intensive tillage, conventional intensive tillage, conventional no tillage, and organic reduced tillage. Drought was simulated after crop establishment using rain shelters. Proportional contributions to plant water uptake from different soil layers were estimated based on stable water isotopes using Bayesian mixing models. Pea plants always took up proportionally more water from shallower depths than barley plants. Water uptake patterns of neither species were affected by cropping systems. Both species showed similar responses to the drought simulation and increased their proportional water uptake from the shallow soil layer (0–20 cm) in all cropping systems. Our results highlight the impact of drought on plant water uptake patterns for two important crop species and suggest that cropping systems might not be as successful as adaptation strategies against drought as previously thought.
- Article
(1221 KB) - Full-text XML
-
Supplement
(511 KB) - BibTeX
- EndNote
-
Pea and barley shifted to shallower water uptake depths in response to drought.
-
No niche differentiation was found between pea and barley in a mixture under drought.
-
No differences on changes in uptake depths by drought were found among cropping systems.
-
Thus, cropping systems did not compensate drought effects on water uptake patterns.
Due to climate change, drought events may occur more frequently and become more severe than at present, and hence water scarcity is worsening in many regions of the world (Schewe et al., 2014; IPCC, 2019). Thus, agriculture is facing increasing pressure to ensure food security under aggravating drought conditions (FAO, 2019, 2018). Although crop breeding has a large potential to enhance agricultural productivity, it should certainly not be seen as the only option. Adaptive crop management to a changing climate is discussed as an additional solution to mitigate yield loss under drought, potentially by sustaining plant growth, enhancing soil water availability, or promoting mycorrhizal symbiosis (Cochard, 2002; Bot and Benites, 2005; Kundel et al., 2020; Wahdan et al., 2021). Therefore, there is a growing interest in organic farming and conservation tillage (i.e. no tillage or reduced tillage), as these management practices have been shown to be beneficial to soil health and water holding capacity, ecosystem stability, and environmental sustainability (e.g. Seitz et al., 2019; Teasdale et al., 2007; Hobbs et al., 2008; Wittwer et al., 2021). However, an evaluation of different cropping systems as a means to support arable crops under drought is still urgently needed (IPCC, 2019).
Understanding plant–water relations under drought plays an increasingly important role in promoting sustainable agriculture to secure food production (Penna et al., 2020). Plant water uptake and water use, particularly during critical growing stages, greatly determine physiological processes, survival, and ultimately crop productivity (Boyer and Rao, 1984; Wang et al., 2015). Although many studies reported plant water uptake patterns in response to drought over a broad range of species and environments (e.g. Ding et al., 2021; Grossiord et al., 2019; Prechsl et al., 2015; Rasmussen et al., 2020), only very few focused on arable agriculture (e.g. Borrell et al., 2014; Wu et al., 2018; Zegada-Lizarazu et al., 2006), and none compared arable cropping systems. Moreover, these studies found contrasting responses of crop species to changing environments, illustrating the current gap of knowledge on plant–water relations in cropping systems.
Plant water uptake mainly depends on soil water availability, root properties and distributions, and soil–plant interactions (von Freyberg et al., 2020). Soil water availability depends on soil physical characteristics and local climatic conditions. Root systems, including root distribution and functionality, are affected by soil physical and nutritional conditions as well as plant growth stages and species genetics. Soil–root interactions include hydrotropism, root damage caused by drying soil, and soil water redistribution (Whitmore and Whalley, 2009; Dietrich et al., 2017; Caldwell et al., 1998). Furthermore, plant water uptake patterns are highly dynamic and difficult to track. Since the 1960s, stable water isotopes, i.e. oxygen and hydrogen isotopes, have been used in ecohydrology studies (Gonfiantini et al., 1965; Zimmermann et al., 1967), e.g. to assess root water uptake patterns (Rothfuss and Javaux, 2017), to detect foliar water uptake (Berry et al., 2019), and to partition evapotranspiration fluxes (Wang et al., 2010). Stable water isotopes have since become a helpful tool to identify plant water uptake sources and quantify source contributions (Dawson and Ehleringer, 1991; Penna et al., 2018). However, studies in agroecosystems have often focused on grassland species (e.g. Prechsl et al., 2015; Bachmann et al., 2015) and much less on crop species as reviewed by Penna et al. (2020).
Hence, our experimental field study investigated how different cropping systems, namely organic vs. conventional farming with intensive vs. conservation tillage, affect plant water uptake patterns under drought using stable water isotopes. We focused on a pea–barley (Pisum sativum L. and Hordeum vulgare L.) mixture, an increasingly popular intercrop for fodder production (Gilliland and Johnston, 1992). We aimed at understanding (1) if pea and barley differ in their water uptake patterns when grown in mixture, (2) how drought affects plant water uptake depths, and (3) if cropping systems affect water uptake depths differently.
2.1 Research site and experimental setup
The research site is in Rümlang near Zurich (47.26∘ N, 8.31∘ E; 489 m a.s.l.) and belongs to the Swiss federal agricultural research station Agroscope. Long-term average annual precipitation at the site is 994 mm, and mean annual air temperature is 9.7 ∘C (1988 to 2017; MeteoSwiss, 2020). The soil at the research site is a calcareous Cambisol with 23 % clay, 34 % silt, and 43 % sand, and the total soil carbon content is 1.6 % to 1.8 % (Loaiza Puerta et al., 2018). The plant available soil depth is 50–70 cm, and no groundwater is accessible for plants (Kanton Zürich, 2020). Our study used a subset of plots in the Farming Systems and Tillage Experiment which began in 2009 with a 6-year crop rotation that is typical for Swiss cropping systems (for details see Wittwer et al., 2017). It combines conventional (C) and organic (O) farming with intensive or soil conservation tillage practices. The conventional systems are managed according to the “Proof of Ecological Performance” (PEP) guidelines of the Swiss Federal Office for Agriculture (Swiss Federal Council, 2021), which allows for synthetic fertiliser and pesticide applications. The organic systems were managed following the Bio Suisse guidelines, prohibiting the use of mineral fertilisers and synthetic plant protection products. Intensive tillage (IT) with a mouldboard plough to 20 cm depth followed by seedbed preparation with a rotary harrow to 5 cm depth was applied in both conventional (C-IT) and organic (O-IT) systems. For conservation tillage, direct sowing and no soil management were implemented in the no tillage conventional plots (C-NT), but glyphosate was sprayed before the sowing of the main crops for weed control. A disc or rotary harrow, which superficially disturbed the soil for weed control, was used for reduced tillage in organically managed plots (O-RT) to a maximum depth of 10 cm. These four cropping systems were repeated in four blocks following a Latin square design. Cropping system plots had an area of 6 m × 30 m.
In 2018, the same pea (Pisum sativum L. cv. Alvesta) and barley (Hordeum vulgare L. cv. Eunova) mixture was sown in all plots on 26 March and harvested on 12 July (108 d). The mixture was composed of 20 % and 80 % of the recommended sowing densities of pea (90 seeds m−2) and barley (350 grains m−2), respectively. The seeds were sown in a mixture with a standard drill-sowing machine. No fertilisation was applied in any of the treatments because the pea plants were expected to fix dinitrogen from the atmosphere.
In order to simulate a future drought scenario (CH2018), portable rain shelters were installed from 22 May to 28 June 2018 (37 d) during the 108 d growing season. This resulted in a 34 % reduction in precipitation from the drought subplots during the growing season in 2018 (from sowing to harvest; Table 1). No irrigation was applied to the control plots during the (unexpected) naturally dry period in June for logistical and rational reasons; i.e. irrigation is unusual for the region and this crop, and the dry period happened during the ripening phase of the crop. The portable, tunnel-shaped rain shelters (metal frames of 3 m × 5 m base area and 2.1 m height at the highest point) were covered with transparent and ultraviolet-light-transmissible plastic foil (Gewächshausfolie UV5, 200 µm, folitec Agrarfolien-Vertrieb, Germany) and were open at both ends as well as at both sides and had an opening at the top along the full length. This allowed for extensive ventilation and prevented temperature build-up (for technical details, see Hofer et al., 2016). Rain running down the foil was collected in PVC (polyvinyl chloride) half pipes and directed away from the plots (about 2 m). These drought subplots were established in each cropping system (which were in place since 2009) and located directly next to control subplots which received natural precipitation inputs, resulting in a split-plot layout. A total of 16 experimental plots (4 cropping systems × 4 replicates) with 32 subplots (16 plots × 2 water availability treatments) were used in this study. Our experimental design thus compared replicated drought and control subplots in parallel (i.e. at the same time), not after each other (i.e. a temporal replication over multiple years), since in crop rotations, the identical crop cannot be grown on the same field for several years due to soil health issues.
2.2 Climatic data and soil water content
Precipitation and air temperature data (Table 1; Fig. 1) were obtained from a nearby weather station, Zürich/Kloten (KLO; 47.48∘ N, 8.54∘ E; 4.6 km north of the research site; MeteoSwiss, 2020). Soil water content (SWC) was continuously measured and recorded at 10 and 40 cm depths with two replicates per cropping system (EC-5, Decagon Devices Inc., Pullman, WA, USA; factory-calibrated). Data were averaged at 10 min intervals by data loggers (CR1000 and CR216, Campbell Scientific Ltd., Loughborough, UK), then averaged for daily values.
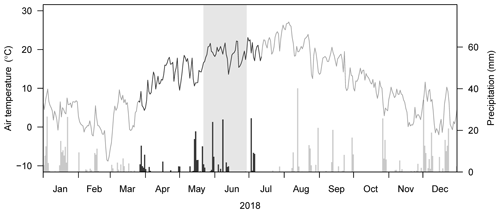
Figure 1Daily air temperature and precipitation in 2018. Dark line segments and bars depict the crop growing season from 26 March to 12 July 2018. The shaded area indicates the drought treatment from 22 May to 28 June 2018. Data from the MeteoSwiss station Zürich/Kloten (KLO; 47.48∘ N, 8.54∘ E; 4.6 km north of the research site; MeteoSwiss, 2020) are given.
2.3 Plant and soil water samples for stable isotope analysis
Plant and soil samples were collected on 7 May, 25 June, and 11 July 2018, i.e. before the drought treatment (BT), at the end of the treatment (ET), and after the treatment (AT), respectively. Pea was not sampled AT due to progressed senescence. Root crowns were collected for stable isotope analysis of plant xylem water, as this part best reflects the mixture of water sources taken up from the soil in herbaceous plants (Barnard et al., 2006; von Freyberg et al., 2020). Four to six individuals were collected and pooled into one sample per species and subplot. Root crowns were cleaned quickly to remove remaining soil and then immediately sealed in air-tight glass tubes (12 mL Exetainer, Labco Ltd., Ceredigion, UK). In parallel to the plant sampling, soil samples were collected close to the sampled plants with a soil auger (1 cm diameter). The soil cores were separated into six depth layers – 0–5, 5–10, 10–20, 20–30, 30–40, and 40–60 cm – and then immediately sealed in glass tubes (18 mL, SCHOTT AG, Mitterteich, Germany). All plant and soil samples for stable water isotope analysis were kept in a cool box in the field and then stored at −18 ∘C before extraction with cryogenic vacuum distillation (Ehleringer and Osmond, 1989). During the extraction, the samples were kept in an 80 ∘C water bath and extracted under 10−2 MPa for 2 h, and the extracted water was collected in glass tubes immersed in liquid nitrogen.
2.4 Stable water isotope analyses
The oxygen and hydrogen stable isotope ratios (δ18O and δ2H) of extracted water samples were analysed by coupling a high-temperature conversion elemental analyser (TC/EA, Finnigan MAT, Bremen, Germany) with an isotope ratio mass spectrometer (IRMS, DeltaplusXP, Finnigan MAT, Bremen, Germany) via a ConFlo III interface (Finnigan MAT; see Werner et al., 1999) using the high-temperature carbon reduction method described by Gehre et al. (2004). All δ18O and δ2H values are expressed relative to the Vienna Standard Mean Ocean Water–Standard Light Antarctic Precipitation (VSMOW-SLAP, Craig and Gordon, 1965; Gat, 2010) in parts per thousand (or “per mil”, ‰; Eq. 1):
where R is the isotope ratio of the rare isotope to the abundant isotope ( or ). The long-term precision of the quality-control standard IsoLab 1 (Stable Isotope Laboratory, Grassland Sciences Group, ETH Zurich) over the last 4 years was 0.22 ‰ for δ18O and 0.59 ‰ for δ2H.
The isotopic composition of precipitation at the global scale shows a linear relationship between the δ18O and δ2H of meteoric waters (global meteoric water line, GMWL; Craig, 1961), described by the regression line in a “dual-isotope” δ18O–δ2H plot (Eq. 2):
Similarly, the local meteoric water line (LMWL) describes the isotopic composition in rainfall for a specific location (Dansgaard, 1964). We fitted the long-term LMWL (1994 to 2017) with monthly mean data from the closest GNIP station (Global Network of Isotopes in Precipitation; Buchs Suhr; 47.37∘ N, 8.08∘ E; 34 km from the research site; IAEA, 2020; Eq. 3), while the LMWL of 2018 was fitted with data of precipitation samples collected at the research site (after Prechsl et al., 2014; Eq. 4) during the growing season and data of 2018 from GNIP Buchs (Fig. S1 in the Supplement):
2.5 Bayesian mixing model for plant water uptake
Proportional contributions of soil water to plant water uptake (PC) from
different depths were estimated using mixing models from the R package
simmr
(Parnell, 2020) within a Bayesian framework based
on code by Parnell et al. (2013). The δ18O or
δ2H signatures of soil water from the six soil layers were used
as sources, and plant xylem water was considered the mixture for modelling
in each subplot at different sampling times, i.e. BT, ET, and AT. Missing
replicates of soil samples due to sampling difficulties (n=5 in total)
were filled with mean values of the other replicates from the same cropping
system and treatment to have balanced model inputs. The model outputs
consisted of 10 000 possible combinations of PC from different soil depths
from four Markov chain Monte Carlo Bayesian models with at least 300 000
iterations, 50 000 burns, and 100 times of thinning for each chain. The
median of the model outputs on PC (MPC) from each soil depth was calculated
for each subplot and used for statistical analysis on plant water uptake
depths. Compared to the most frequent value of the model outputs, MPCs of
all the sources usually sum up closer to 1. To increase the clarity of
presentation, PC was grouped into three layers, namely shallow (0–20 cm),
middle (20–40 cm), and deep (40–60 cm) soil layers for further analyses. The
PC values from the shallow and middle layers are the sum of PC from soil depths
of 0–5, 5–10, and 10–20 cm and the sum of PC from soil depths of 20–30 and
30–40 cm, respectively. As δ18O and δ2H yielded
similar results, only the model outputs of δ18O are described
in detail in this paper.
2.6 Data analyses
For data analyses, the whole growing season was divided into three periods
based on the drought treatment, namely before the drought treatment (BT; 26 March to 21 May); the drought treatment period itself (22 May to 28 June),
which was sampled directly before the removal of shelters on 28 June (termed
ET, end of the treatment); and after the drought treatment (AT, 29 June to 12 July). All statistical analyses were carried out using R (v3.6.2; R Core Team, 2020). The effects of the cropping system, drought treatment,
and species were tested with linear mixed models using the function lmer()
from
the R package lmerTest
(Kuznetsova et al., 2017).
“Cropping system (CS)”, “drought treatment (D)”, and “blocks” were three
fixed factors (Dixon, 2016); interactive effects between
CS and D with “plots” (accounting for the split-plot design) were
considered random factors. For variables measured on both pea and barley
(i.e. stable isotopes of xylem water and MPC for BT and ET), “plant
species”, CS, D, and blocks were tested as fixed factors considering
interactive effects among plant species, CS, and D with plots and
“subplots” as random factors. Diagnostic plots were checked for the normality
and homoscedasticity of residuals for model assumptions. Differences among
cropping systems and between treatments or species were tested by Tukey's
HSD (honestly significant difference) test using the function glht()
, from the R
package multcomp
(Hothorn et al., 2008).
3.1 Environmental conditions in drought and control subplots
Air temperatures in 2018 were very high compared to the long-term mean, in particular in May and June, with a daily average air temperature of 15.8 and 18.8 ∘C, respectively, while the long-term (1988 to 2017) mean air temperatures in these 2 months were 13.9 and 17.2 ∘C, respectively (Table 1; Fig. 1). Annual precipitation was relatively low (Table 1). While the precipitation in May 2018 (102 mm) was comparable to the long-term mean (1988 to 2017: 105 mm), no precipitation fell between 14 June and 2 July 2018 (naturally dry period), resulting in a below-average precipitation in June (40 mm; long-term mean of 102 mm, Table 1), followed by an even more pronounced drought period in July (Fig. 1). Average daily soil water content (SWC) in the control subplots ranged from 16 % to 29 % at 10 cm depth and slightly higher, from 22 % to 29 %, at 40 cm depth, prior to the rain event on 3 July 2018. After this rain event, SWC increased in all cropping systems at both depths (Fig. 2a, b). Variations in SWC among cropping systems were small, particularly during the naturally dry period in June. SWC in drought subplots of all cropping systems decreased continuously during the 37 d drought treatment (22 May to 28 June 2018), averaging to 13 % at 10 cm and to 19 % at 40 cm soil depth (Fig. 2c, d). SWC at 10 cm did not show any pronounced differences among cropping systems, while SWC at 40 cm tended to be slightly higher in cropping systems with conservation tillage (O-RT and C-NT) compared to systems with intensive tillage (O-IT and C-IT; Fig. 2b, d).
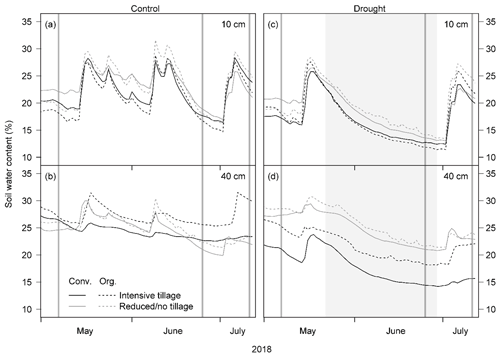
Figure 2Daily mean soil water content at 10 and 40 cm depth in (a, b) control and (c, d) drought subplots under different cropping systems (n=2 each; Conv. for conventional and Org. for organic). Vertical lines indicate sampling dates for stable water isotopes on 7 May, 25 June, and 11 July 2018. Shaded areas in (c) and (d) represent the drought treatment period from 22 May to 28 June 2018.
3.2 Stable isotopes in soil water and plant xylem water
In the dual-isotope space, stable oxygen and hydrogen isotope ratios of soil and plant xylem waters were strongly related to each other (R2=0.89 and 0.85, respectively; Fig. S1) and generally fell below the local meteoric water line (LMWL) of 2018, representing evaporation. Stable isotope signatures of xylem water were lower than the LMWL but higher than those of soil water, indicating that xylem water isotope signatures were mixtures of the original source precipitation and the pool of soil water, affected by different degrees of fractionation.
The stable water isotope profiles of soil water showed a characteristic pattern at all times, for all cropping systems and both treatments, with most enriched values in the uppermost soil and increasingly depleted values with increasing soil depth (Table S1 in the Supplement; Fig. 3 for δ18O; Fig. S2 for δ2H). The drought treatment showed significant effects before the treatment (BT) for neither δ18O nor δ2H (except for δ2H at 20–30 cm; Table 2). In contrast, at the end of the drought treatment (ET), soil water δ18O values from 20–60 cm (20–30, 30–40, and 40–60 cm) as well as δ2H values from all depths were strongly affected by the drought treatment (all values of p<0.05; Table 2), with more depleted signatures in the drought than in control subplots due to the exclusion of more enriched summer precipitation. Even after the shelters were removed and the treatment had been finished (AT), the drought treatment still significantly affected both δ18O and δ2H of soil water, albeit only in deeper soil depths (30–40 and 40–60 cm for δ18O and 40–60 cm for δ2H; all values of p<0.05; Table 2). Overall, cropping systems did not significantly affect the stable isotopic signatures in soil water at any time (Table 2).
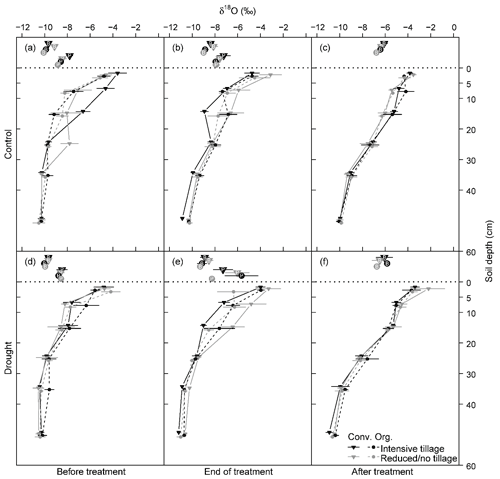
Figure 3δ18O values of soil water from different depths and plant xylem water in each cropping system (a, d) before the drought treatment on 7 May, (b, e) at the end of the drought treatment on 25 June, and (c, f) after the treatment on 11 July 2018 (Conv. for conventional and Org. for organic). Horizontal dotted lines separate isotopic composition of soil and plant samples (P for pea and B for barley). Pea plants were already senesced in early July; therefore no stable water isotope data are available after the drought treatment. Means and 1 SE (standard error; horizontal bars) are given for each cropping system (n = 3–4).
Table 2Effects of the cropping system (CS, df = 3; degrees of freedom), drought treatment (D, df = 1), and interaction (CS × D, df = 3) on stable water isotopes (δ18O and δ2H) in different soil depths before the drought treatment on 7 May, at the end of the treatment on 25 June, and after the treatment on 11 July (in 2018 tested by linear mixed models; p values are given).
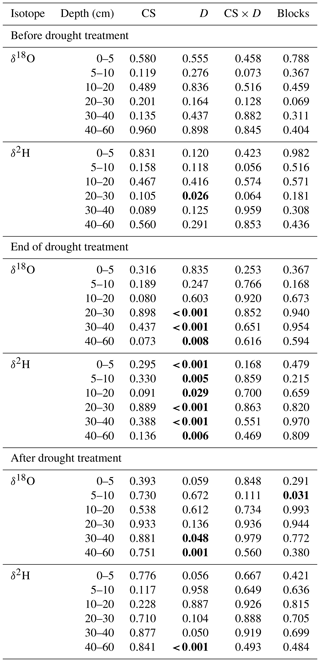
Significant differences are shown in bold (p<0.05).
Pea xylem water was always significantly more enriched in 18O and 2H compared to barley (all values of p<0.001; Table S2). The δ18O values in xylem water for pea ranged between −8.8 ‰ and −5.7 ‰ and were significantly lower between −10.1 ‰ and −5.8 ‰ for barley (averages per cropping system, treatment, and time; Tables 3 and S2). Similarly, the δ2H values in xylem water for pea ranged between −65.6 ‰ and −52.1 ‰ and were significantly lower between −74 ‰ and −47.1 ‰ for barley (Tables 3 and S2). Overall, isotopic signatures in xylem water became more enriched in 18O and 2H during the growing season for both pea and barley (Figs. 3 and S2, Table S2). On average, the xylem δ18O for pea was −8.5 ‰ before the treatment (BT) and −7.2 ‰ at the end of the treatment (ET), compared to −9.8 ‰ (BT), −8.8 ‰ (ET), and −6.3 ‰ after the treatment (AT) for barley. While average δ2H values for pea were −64.1 ‰ (BT) and −57.6 ‰ (ET), δ2H values averaged −72.2 ‰ (BT), −68.6 ‰ (ET), and −50.8 ‰ (AT) for barley (Figs. 3 and S2, Table S1). Since there was a strong relationship between δ18O and δ2H in xylem water (Fig. S1; R2=0.85), our analyses are mainly focused on δ18O in the text (but see Tables 3, S2, and Fig. S2 for analyses on δ2H).
Table 3Effects of the species (df = 1), cropping system (CS, df = 3), drought treatment (D, df = 1), and interaction (species × CS, df = 3; species × D, df = 1; CS × D, df = 3; species × CS × D, df = 3) on stable water isotopes (δ2H and δ18O) of pea and barley before the drought treatment on 7 May and at the end of the treatment on 25 June 2018 tested by linear mixed models (p values are given).
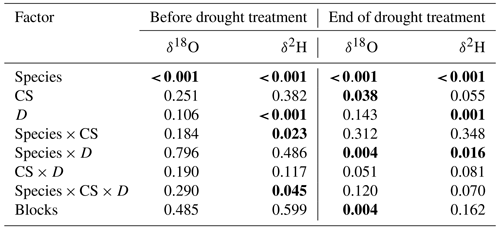
Significant differences are shown in bold (p<0.05).
For pea, cropping systems significantly affected neither δ18O nor δ2H in xylem water at either time (BT and ET; Table S2), while the drought treatment significantly affected the isotopic signatures of 18O only at the end of the treatment (ET: p=0.022; no interactions between cropping systems and drought treatment: p=0.085; Table S2). 18O in pea xylem water were significantly more enriched in the drought than in the control subplots (on average, δ18O of −6.9 ‰ and −7.7 ‰, respectively).
In contrast to pea, cropping systems significantly affected δ18O in barley xylem water (ET: p=0.035; Table S2). The drought treatment significantly affected the isotope signatures of both 18O and 2H at the end of the treatment (ET: both values of p<0.01; no interactions between cropping systems and drought treatment; Table S2). However, unlike pea, the xylem water of barley showed significantly lower δ18O values in drought than in control subplots for all cropping systems (on average, −9.0 ‰ and −8.6 ‰, respectively), although the difference was small (Table S2). A similar pattern was also observed for δ2H at the end of the treatment (ET), with significantly lower values on average in drought than in control subplots (ET: −71.8 ‰ and −65.4 ‰, respectively).
3.3 Modelled plant water uptake depths
The outputs of the Bayesian mixing model on the proportional contribution to total plant water uptake (PC) showed highly significantly different behaviours of pea and barley, mirroring some of the differences seen in the xylem water isotopic signatures of these two species (Figs. 4 and 5). Since frequency density distributions provide not just one estimate per soil depth but rather a full frequency distribution, the medians were calculated for each soil depth to assist in the analyses (Table S3 for results from δ18O; Table S4 for results from δ2H). As both stable isotope signatures showed similar results, we here focus on results derived from δ18O only. In addition, we grouped the uptake depths into shallow (0–20 cm as the sum of 0–5, 5–10, and 10–20 cm), middle (20–40 cm as the sum of 20–30 and 30–40 cm), and deep (the original 40–60 cm) soil layers (Tables 4 and 5). Overall, both species took up water from the entire soil profile studied (0 to 60 cm soil depth), albeit with different proportions depending on species, time (i.e. BT, ET, and AT), and treatment (i.e. control vs. drought; Tables 4 and 5).
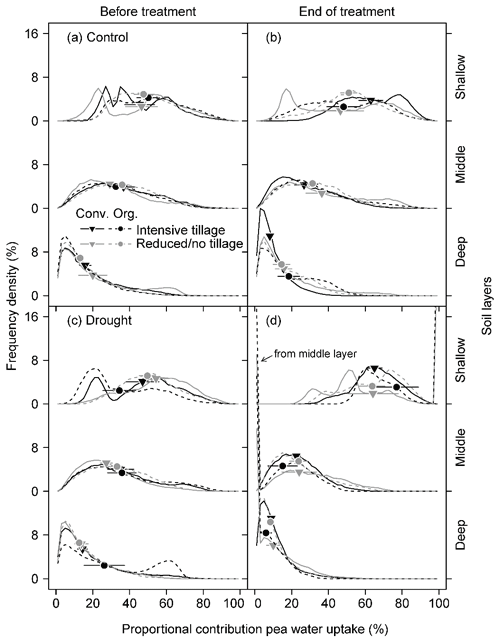
Figure 4Frequency density distribution of model outputs on the proportional contribution of soil water to pea water uptake from the shallow (0–20 cm; sum of 0–5, 5–10, and 10–20 cm), middle (20–40 cm; sum of 20–30 and 30–40 cm), and deep (40–60 cm) soil layers under different cropping systems (a, b) before the drought treatment on 7 May and (c, d) at the end of the treatment on 25 June 2018. Frequency density was derived from 10 000 simulations at a 2 % increment of mixing models using δ18O for each subplot (Conv. for conventional and Org. for organic). Data were pooled for all subplots in each cropping system. Symbols on the curves indicate the median of the model outputs for each soil layer. Means and 1 SE (horizontal bars) of each cropping system are given (n = 3–4).
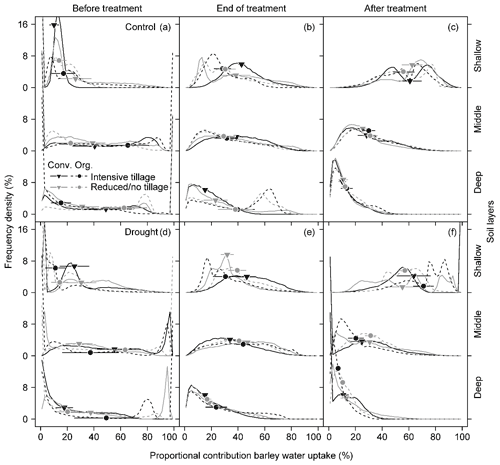
Figure 5Frequency density distribution of model outputs on the proportional contribution of soil water to barley water uptake from the shallow (0–20 cm; sum of 0–5, 5–10, and 10–20 cm), middle (20–40 cm; sum of 20–30 and 30–40 cm), and deep (40–60 cm) soil layers under different cropping systems (a, b) before the drought treatment on 7 May, (c, d) at the end of the treatment on 25 June, and (e, f) after the treatment on 11 July 2018. Frequency density was derived from 10 000 simulations at a 2 % increment of mixing models using δ18O for each subplot (Conv. for conventional and Org. for organic). Data were pooled for all subplots in each cropping system. Symbols on the curves indicate the median of the model outputs for each soil layer. Means and 1 SE (horizontal bars) of each cropping system are given (n = 3–4).
Table 4Effects of the species (df = 1), cropping system (CS, df = 3), drought treatment (D, df = 1), and interaction (species × CS, df = 3; species × D, df = 1; CS × D, df = 3; species × CS × D, df = 3) on the median proportional contribution from different soil depths to water uptake (MPC) of pea and barley before the drought treatment on 7 May and at the end of the treatment on 25 June 2018 tested by linear mixed models (p values are given).
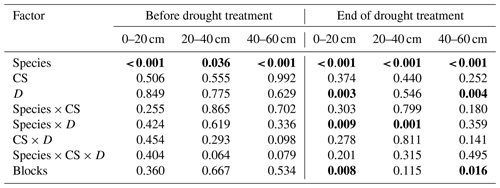
MPC was derived from 10 000 simulations by mixing models using δ18O data. Proportional contribution from 0–20 cm is the sum from 0–5, 5–10, and 10–20 cm, and that from 20–40 cm is the sum from 20–30 and 30–40 cm. Significant differences are shown in bold (p<0.05).
Table 5Median proportional contribution (MPC) from different soil depths to water uptake of pea and barley before the drought treatment on 7 May, at the end of the treatment on 25 June, and after the drought treatment on 11 July 2018 (left) as well as effects of the cropping system (CS, df = 3), drought treatment (D, df = 1), and interaction (CS × D, df = 3) on MPC tested by linear mixed models. Means and 1 SE (MPC) and p values are given.
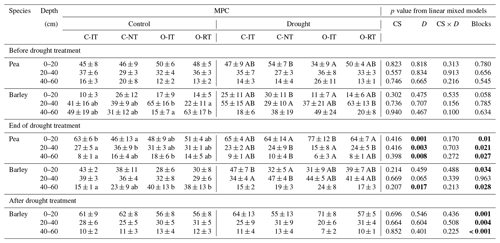
MPC was derived from 10 000 simulations by mixing models using δ18O data. Pea plants were already senesced in early July; therefore no stable water isotope data are available after the treatment. Proportional contribution from 0–20 cm is the sum from 0–5, 5–10, and 10–20 cm, and that from 20–40 cm is the sum from 20–30 and 30–40 cm. Means and 1 SE for MPC (%) are given for different cropping systems (C-IT for conventional intensive tillage, C-NT for conventional no tillage, O-IT for organic intensive tillage, and O-RT for organic reduced tillage). Different lowercase and capital letters indicate significant differences among cropping systems in control and drought subplots, respectively, tested with Tukey's HSD (honestly significant difference, p<0.05). Significant effects tested with linear mixed models are shown in bold (p<0.05).
For pea, soil water contributions to total plant water uptake decreased with increasing soil depth in both control and drought subplots before (BT) and at the end of the treatment (ET) for all cropping systems (Fig. 4). The median of PC values (MPC) differed significantly among shallow (0–20 cm), middle (20–40 cm), and deep (40–60 cm) layers, averaging 47 %, 33 %, and 16 %, respectively, for both treatments and all cropping systems (BT; Table 5; Fig. 4a, c). At ET, pea plants subjected to drought significantly shifted their water uptake to even higher contributions from the shallow layer (67 %) and less uptake from the middle (22 %) and deep (8 %) soil layers compared to BT (Tables 5 and S5; Fig. 4d). Pea plants in control subplots did not display such a significant shift but remained with average MPC from the shallow, middle, and deep soil layers of 52 %, 31 %, and 14 %, respectively (Tables 5 and S5; Fig. 4b). Cropping systems did not significantly affect MPC before (BT) or at the end of the treatment (ET; also no interactions between cropping systems and drought, Table 5; Fig. 4d).
In contrast to pea, barley plants showed very different water uptake patterns before the treatment (BT), with significantly lower PC from the shallow soil layer compared to the middle and deep layers. For barley, MPC values averaged 19 %, 44 %, and 35 % for shallow, middle, and deep soil layers, respectively, for both treatments and all cropping systems (Fig. 5a, d). However, at the end of the treatment (ET), barley plants significantly increased the contributions from the shallow layer in drought subplots, similar to pea (Tables 5 and S5; Fig. 5e), resulting in MPC values of 38 %, 41 %, and 18 % from the shallow, middle, and deep soil layers, respectively. The MPC further shifted after the treatment (AT) to values of 62 %, 27 %, and 10 % from the shallow, middle, and deep layers, respectively (Fig. 5f). Also in control subplots, barley plants showed the same significant shift from BT to ET, with MPC values at ET of 35 %, 34 %, and 29 % from the shallow, middle, and deep layers, respectively (Tables 5 and S5; Fig. 5b), and from ET to AT with MPC values AT of 59 %, 29 %, and 12 % from the shallow, middle, and deep layers, respectively (Tables 5 and S5; Fig. 5c). Similar to pea, barley water uptake patterns were not significantly affected by cropping systems (Table 5).
Overall, MPC values from the shallow and deep layers for pea and barley were positively correlated (r = 0.64 and 0.55, respectively; Fig. S3). This means when barley took up more water from the shallow layer, so did pea.
Cropping system types organic and reduced/no tillage are discussed as adaptation strategies under climate change conditions to ensure arable crop production. Thus, we analysed plant water uptake depths in drought subplots at the end of the treatment (ET) more in detail, although cropping systems showed no significant effects on water uptake depths for either species, and no interactions occurred between cropping systems and drought treatment (Table 5). Pea plants in both intensive systems (C-IT and O-IT) showed significantly higher (O-IT: 77 %) or similar (C-IT: 65 %) contributions to total water uptake (such as MPC) from the shallow layer (0–20 cm) compared to conservation tillage systems (64 % in both C-NT and O-RT; Table 5; Fig. 4d). Conversely, contributions from the middle layer (20–40 cm) for pea at the end of the treatment (ET) were only 15 % in O-IT compared to 24 % in the other three cropping systems (O-RT, C-IT, and C-NT). Differences among cropping systems under drought were even smaller for barley than for pea (Table 5; Fig. 5e). MPC values of barley for uptake from the shallow layer were 47 % (C-IT), 39 % (O-RT), 31 % (O-IT), and 32 % (C-NT). Conversely, contributions from the middle layer were the largest in C-NT (47 %), followed by O-IT (44 %) and O-RT (41 %), and lowest in C-IT (34 %). The absolute changes in MPC values between before the treatment (BT) and the end of the treatment (ET) were not significantly affected by cropping systems for either species but were significantly affected by the drought treatment for pea (for the shallow and middle soil layers; Table S6).
Root water uptake patterns are often discussed for their important role in plant–water relations, but only few studies considered arable crop species (Penna et al., 2020). In addition, most studies on responses of crop root water uptake patterns to drought took place in pots or under controlled conditions (e.g. Araki and Iijima, 2005; Zegada-Lizarazu and Iijima, 2004) so that information on field conditions is particularly scarce, except maize (Ma and Song, 2016), wheat (Ma and Song, 2018), oilseed rape, and barley in monoculture (Wu et al., 2016). Furthermore, studies comparing the role of different cropping systems for crop water uptake are completely lacking. Here, we showed for the first time that root water uptake patterns of field-grown pea and barley in mixture responded to drought but not to different cropping systems. Subjected to a pronounced drought period (37 d without precipitation), both crop species shifted to relying more on the shallow soil layer (0–20 cm) for water uptake. This drought response was independent of the cropping system, i.e. organic vs. conventional farming or intensive vs. conservation tillage.
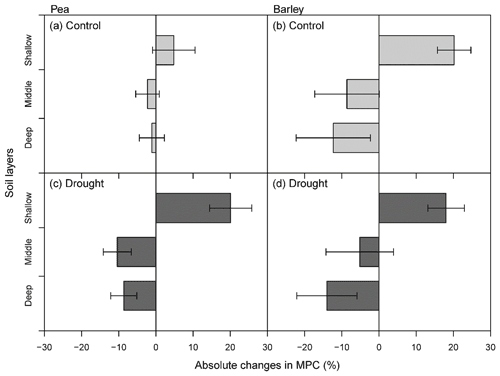
Figure 6Absolute changes in median proportional contribution (MPC) to plant water uptake of (a, c) pea and (b, d) barley, calculated as the difference of MPC at the end (25 June; ET) and before the drought treatment (7 May; BT), from three soil layers in (a, b) control and (c, d) drought subplots in all cropping systems. MPC was derived from 10 000 simulations of mixing models using stable water isotope data. Proportional contribution from the shallow layer is the sum of 0–5, 5–10, and 10–20 cm depths; the middle layer is the sum of 20–30 and 30–40 cm depths; and the deep layer represents 40–60 cm. Means and 1 SE (horizontal lines) are given (n = 14–16).
Previous research on root water uptake patterns in crop as well as grassland species showed ambiguous responses to drought. For some species, root water uptake depth was dependent on root distribution during wet periods but on soil water availability during dry periods (Sprenger et al., 2016). Therefore, utilising more water from the deep rather than from the shallow soil layer is typically the anticipated drought response, such as barley in monoculture (Wu et al., 2018), maize (Ma and Song, 2016), wheat, rice, soybean (Zegada-Lizarazu and Iijima, 2004), or chickpea (Purushothaman et al., 2017). However, other studies reported that crop and grassland species do not take up water from deeper depths under drought but rather absorb more water from the shallow soil layer (e.g. barley in monoculture; maize; pigeon pea; cowpea, Zegada-Lizarazu and Iijima, 2004; or grassland species, Hoekstra et al., 2014; Prechsl et al., 2015; Wu et al., 2016). This is in accordance with our results in which both pea and barley increased their proportional water uptake from the shallow layer (0–20 cm) at the end of the treatment (ET) in the drought subplots. Although soil water content (SWC) values were still higher at 40 cm than at 10 cm at the end of the treatment (ET; Fig. 2c, d), SWC values at 40 and 10 cm depths were both very low. Thus, the whole soil profile showed very low water availability at the end of the treatment (ET), and fine root distributions most likely dominated plant water uptake patterns.
Rooting profiles for legumes with increased proportions of deeper roots under drought, e.g. below 23–30 cm, have been reported (Benjamin and Nielsen, 2006; Purushothaman et al., 2017), although different responses in root growth to drought were found among different varieties (Kumar et al., 2012; Kashiwagi et al., 2006; Purushothaman et al., 2017). The architecture of legume root systems is strongly affected by rhizobia, which typically find better living conditions in terms of oxygen and nitrogen concentrations higher up in the soil profile than at greater depths (Concha and Doerner, 2020) and also in dry soils. Moreover, barley grown under drought conditions has been reported to develop proportionally more shallow roots (0–20 cm depth) relative to deeper soil depths (Carvalho et al., 2014). Also, studies on grassland plants (both legume and grass species) found increasing root biomass production in shallow soil depths (0–15 cm) in response to drought (e.g. Prechsl et al., 2015). Although we did not investigate root distributions for either crop species, they most likely followed evolutionary strategies such as those also during our rather strong, 37 d drought treatment, in addition to recent crop breeding efforts leading to less deep root systems in general (Canadell et al., 1996; Thorup-Kristensen et al., 2020). Moreover, shifting to shallower water uptake depths during drought might actually be beneficial for nutrient acquisition (Querejeta et al., 2021), since not only concentrations of soil water and atmospheric N2 but also litter inputs for N mineralisation are higher in the topsoil than in the deeper soil. Thus, besides the low soil moisture within the entire soil profile, acclimation of the root systems most likely also contributed to the shift towards shallower water uptake depths under drought for both pea and barley in this study.
The year 2018 was characterised by low precipitation during our experimental period, when a naturally dry period occurred at the end of our pronounced drought treatment in June (which excluded 34 % of the precipitation during the growing season; Table 1). Our treatment compared well with the climate scenarios available for Switzerland, with a 25 % reduction of precipitation in 2060 and up to 40 % by the end of the century, and an increase of the longest rain-free summer period (June, July, and August) from currently 11 to 20 d (CH2018). The dry period in June affected pea and barley plants in our control subplots differently (Fig. 6a, b). While pea did not shift its water uptake pattern (Fig. 6a; Table S5), barley grown in the control subplots reacted very similarly to the natural dry period (before the ET sampling, 14 to 25 June; Fig. 2) as barley subjected to our drought treatment, namely with a clear shift from the deep (40–60 cm) to the shallow (0–20 cm) soil layer (Fig. 6b, d; Table S5). However, barley still relied more on water uptake from the deep soil layer during this naturally dry period in the control subplots than under the experimental drought (p=0.017; Table 5). Hence, these different reactions of the two species to the dry period clearly indicated that barley was more susceptible than pea even to a mild water stress. This observation is fully in line with measurements of stem hydraulic traits (i.e. loss of xylem conductance) from the same experiment (Sun et al., 2021). Barley plants lost xylem conductance much earlier than pea plants when xylem water potentials decreased. In addition, legumes like pea can maintain low stomatal conductance to avoid water stress without compromising photosynthesis when growing under conditions with a limiting water supply, due to their high foliar N concentrations (Adams et al., 2018). This adds to the hydraulic trait benefits of pea and explains why pea was less affected by the natural dry period. Nevertheless, as shown in our study, if severities and frequencies of droughts increase in the future, one can expect negative consequences on the performance not only of barley but also of pea (Martin and Jamieson, 1996).
Moreover, the two species growing together in the pea–barley mixture showed distinct niches for root water uptake before drought, with pea relying more on water from the shallow (0–20 cm) and barley from the deep (40–60 cm) soil layers, in accordance with resource partitioning in the absence of water limitation as observed in intercrops, e.g. pearl millet and cowpea (Zegada-Lizarazu et al., 2006), and in mixed-species grasslands (e.g. Hoekstra et al., 2014). However, the niches became more similar under drought conditions, contradicting ecological theory which postulates more pronounced niche differentiation and less niche overlap under stressful conditions, such as during a drought (see Guderle et al., 2018; Silvertown et al., 2015; Nippert and Knapp, 2007). However, our results were in line with results from biodiversity studies in temperate grasslands (Barry et al., 2020; Bachmann et al., 2015; Hoekstra et al., 2014) which also did not show niche differentiation in response to increased competition or drought. Thus, further detailed knowledge on the dynamics of intercrop water uptake patterns is needed to solve this contradiction and to decrease the uncertainty for arable crop production now and under future climate conditions.
As global agriculture has already been considerably compromised by and become increasingly sensitive to climate change (Ortiz-Bobea et al., 2021), farming practices such as organic management and conservation tillage are being discussed widely. They have been shown to improve general soil conditions compared to conventional management and intensive tillage, particularly under drought (Bot and Benites, 2005; Gomiero et al., 2011; Choudhary et al., 2016). For instance, organic management and conservation tillage can increase soil water holding capacity, therefore providing higher water availability than conventional management and intensive tillage (e.g. Colombi et al., 2019; Kundel et al., 2020). In this study, the systems with conservation tillage (C-NT and O-RT) indeed showed slightly higher SWC than systems with intensive tillage (C-IT and O-IT) at 40 cm (Fig. 2d). However, this did not result in any benefit for root water uptake patterns of pea and barley against drought. Water uptake of both species shifted to the shallow layer (0–20 cm) in all cropping systems under drought, without cropping system effects or interactive effects between cropping systems and drought treatment. Thus, any further changes in soil physical characteristics due to the drought treatment among cropping systems did not affect the observed root water uptake patterns. The relatively short period that annual crop species are growing under these conditions might limit the potential benefits from improved soil conditions present in those systems (e.g. Dennert et al., 2018; Loaiza Puerta et al., 2018; Schluter et al., 2018). Although it remains to be seen if the observed behaviour of a pea–barley mixture also holds true for other crop species, our results clearly challenge the potential of cropping management under temperate climate as a tool to adapt arable agriculture to climate change.
Water uptake patterns of pea and barley both shifted under drought in all cropping systems, and both species relied more on water from the shallow soil layer (0–20 cm) than on water from deeper in the soil profile. This was also the case for cropping system types organic and reduced/no tillage, which are often discussed as beneficial for crop performance, particularly under water-limited conditions, and are thus suggested as adaptive cropping management practices under a future climate. However, in this study, we showed for the first time that cropping systems could not counteract the effects of severe drought on plant water uptake patterns for pea and barley grown in mixture. It remains to be seen if this observation also holds true for other major crops grown under water-limited conditions.
The data that support the findings of this study will be openly available in the ETH Zurich Research Collection at https://www.research-collection.ethz.ch/ (last access: 20 February 2022; https://doi.org/10.3929/ethz-b-000533193, Sun, 2022).
The supplement related to this article is available online at: https://doi.org/10.5194/bg-19-1853-2022-supplement.
NB, AKG, RW, and MGAvdH designed the study. QS, AKG, and VHK carried out the fieldwork. QS analysed the data. QS, AKG, and NB wrote the first drafts of the manuscript. All authors discussed the results and revised and agreed on the final version of the manuscript.
The contact author has declared that neither they nor their co-authors have any competing interests.
Publisher’s note: Copernicus Publications remains neutral with regard to jurisdictional claims in published maps and institutional affiliations.
The authors would like to thank the RELOAD team, in particular Emily Oliveira and Ivo Beck for their great technical and logistical support; Reto Zihlmann from ETH Zurich statistical consulting service for advice on statistical analyses; Yangyang Jia, Gicele Silva Duarte Sa, Xingyu Hu, and Ming Yi for their assistance with fieldwork; and Elham Rouholahnejad for constructive scientific discussion. Roland A. Werner and Annika Ackermann are greatly acknowledged for measurements of many water isotope samples.
This work was supported by the Mercator Research Program of the ETH Zurich World Food System Center and the ETH Zurich Foundation.
This paper was edited by Serita Frey and reviewed by two anonymous referees.
Adams, M. A., Buchmann, N., Sprent, J., Buckley, T. N., and Turnbull, T. L.: Crops, nitrogen, water: Are legumes friend, foe, or misunderstood ally?, Trends Plant Sci., 23, 539–550, https://doi.org/10.1016/j.tplants.2018.02.009, 2018.
Araki, H. and Iijima, M.: Stable isotope analysis of water extraction from subsoil in upland rice (Oryza sativa L.) as affected by drought and soil compaction, Plant Soil, 270, 147–157, https://doi.org/10.1007/s11104-004-1304-2, 2005.
Bachmann, D., Gockele, A., Ravenek, J. M., Roscher, C., Strecker, T., Weigelt, A., and Buchmann, N.: No evidence of complementary water use along a plant species richness gradient in temperate experimental grasslands, Plos One, 10, e0116367, https://doi.org/10.1371/journal.pone.0116367, 2015.
Barnard, R. L., de Bello, F., Gilgen, A. K., and Buchmann, N.: The δ18O of root crown water best reflects source water δ18O in different types of herbaceous species, Rapid Commun. Mass Sp., 20, 3799–3802, https://doi.org/10.1002/rcm.2778, 2006.
Barry, K. E., van Ruijven, J., Mommer, L., Bai, Y. F., Beierkuhnlein, C., Buchmann, N., de Kroon, H., Ebeling, A., Eisenhauer, N., Guimaraes-Steinicke, C., Hildebrandt, A., Isbell, F., Milcu, A., Nesshover, C., Reich, P. B., Roscher, C., Sauheitl, L., Scherer-Lorenzen, M., Schmid, B., Tilman, D., von Felten, S., and Weigelt, A.: Limited evidence for spatial resource partitioning across temperate grassland biodiversity experiments, Ecology, 101, e02905, https://doi.org/10.1002/ecy.2905, 2020.
Benjamin, J. G. and Nielsen, D. C.: Water deficit effects on root distribution of soybean, field pea and chickpea, Field Crops Res., 97, 248–253, https://doi.org/10.1016/j.fcr.2005.10.005, 2006.
Berry, Z. C., Emery, N. C., Gotsch, S. G., and Goldsmith, G. R.: Foliar water uptake: Processes, pathways, and integration into plant water budgets, Plant Cell Environ., 42, 410–423, 2019.
Borrell, A. K., van Oosterom, E. J., Mullet, J. E., George-Jaeggli, B., Jordan, D. R., Klein, P. E., and Hammer, G. L.: Stay-green alleles individually enhance grain yield in sorghum under drought by modifying canopy development and water uptake patterns, New Phytol., 203, 817–830, https://doi.org/10.1111/nph.12869, 2014.
Bot, A. and Benites, J.: The importance of soil organic matter: Key to drought-resistant soil and sustained food production, Food & Agriculture Organization, ISBN 92-5-105366-9, ISSN 0253-2050, 2005.
Boyer, J. S. and Rao, I. M.: Magnesium and the acclimation of photosynthesis to low leaf water potentials, Plant Physiol., 74, 161–166, 1984.
Caldwell, M. M., Dawson, T. E., and Richards, J. H.: Hydraulic lift: Consequences of water efflux from the roots of plants, Oecologia, 113, 151–161, https://doi.org/10.1007/s004420050363, 1998.
Canadell, J., Jackson, R. B., Ehleringer, J. R., Mooney, H. A., Sala, O. E., and Schulze, E. D.: Maximum rooting depth of vegetation types at the global scale, Oecologia, 108, 583–595, https://doi.org/10.1007/Bf00329030, 1996.
Carvalho, P., Azam-Ali, S., and Foulkes, M. J.: Quantifying relationships between rooting traits and water uptake under drought in Mediterranean barley and durum wheat, J. Integ. Plant Biol., 56, 455–469, 2014.
Choudhary, M., Ghasal, P. C., Kumar, S., Yadav, R., Singh, S., Meena, V. S., and Bisht, J. K.: Conservation agriculture and climate change: an overview, in: Conservation Agriculture, edited by: Bisht, J., Meena, V., Mishra, P., and Pattanayak, A., Springer, 1–37, https://doi.org/10.1007/978-981-10-2558-7_1, 2016.
Cochard, H.: Xylem embolism and drought-induced stomatal closure in maize, Planta, 215, 466–471, https://doi.org/10.1007/s00425-002-0766-9, 2002.
Colombi, T., Walder, F., Büchi, L., Sommer, M., Liu, K., Six, J., van der Heijden, M. G. A., Charles, R., and Keller, T.: On-farm study reveals positive relationship between gas transport capacity and organic carbon content in arable soil, SOIL, 5, 91–105, https://doi.org/10.5194/soil-5-91-2019, 2019.
Concha, C. and Doerner, P.: The impact of the rhizobia-legume symbiosis on host root system architecture, J. Exp. Bot., 71, 3902–3921, https://doi.org/10.1093/jxb/eraa198, 2020.
Craig, H.: Isotopic variations in meteoric waters, Science, 133, 1702–1703, https://doi.org/10.1126/science.133.3465.1702, 1961.
Craig, H. and Gordon, L. I.: Deuterium and oxygen 18 variation in the ocean and the marine atmosphere, in: Stable Isotopes in Oceanographic Studies and Paleotemperatures, edited by: Tongiorgi, E., Consiglio Nazionale delle Ricerche, Laboratorio di Geologia Nucleare, Pisa, Italy, 9–130, 1965.
Dansgaard, W.: Stable isotopes in precipitation, Tellus, 16, 436–468, 1964.
Dawson, T. E. and Ehleringer, J. R.: Streamside trees that do not use stream water, Nature, 350, 335–337, https://doi.org/10.1038/350335a0, 1991.
Dennert, F., Imperiali, N., Staub, C., Schneider, J., Laessle, T., Zhang, T., Wittwer, R., van der Heijden, M. G., Smits, T. H. M., Schlaeppi, K., Keel, C., and Maurhofer, M.: Conservation tillage and organic farming induce minor variations in Pseudomonas abundance, their antimicrobial function and soil disease resistance, FEMS Microbiol. Ecol., 94, fiy075, https://doi.org/10.21256/zhaw-3904, 2018.
Dietrich, D., Pang, L., Kobayashi, A., Fozard, J. A., Boudolf, V., Bhosale, R., Antoni, R., Nguyen, T., Hiratsuka, S., Fujii, N., Miyazawa, Y., Bae, T. W., Wells, D. M., Owen, M. R., Band, L. R., Dyson, R. J., Jensen, O. E., King, J. R., Tracy, S. R., Sturrock, C. J., Mooney, S. J., Roberts, J. A., Bhalerao, R. P., Dinneny, J. R., Rodriguez, P. L., Nagatani, A., Hosokawa, Y., Baskin, T. I., Pridmore, T. P., De Veylder, L., Takahashi, H., and Bennett, M. J.: Root hydrotropism is controlled via a cortex-specific growth mechanism, Nat. Plants, 3, 17057, https://doi.org/10.1038/nplants.2017.57, 2017.
Ding, Y. L., Nie, Y. P., Chen, H. S., Wang, K. L., and Querejeta, J. I.: Water uptake depth is coordinated with leaf water potential, water-use efficiency and drought vulnerability in karst vegetation, New Phytol., 229, 1339–1353, https://doi.org/10.1111/nph.16971, 2021.
Dixon, P. M.: Should blocks be fixed or random?, Statistics Conference Proceedings, Presentations and Posters, 6, https://doi.org/10.4148/2475-7772.1474, 2016.
Ehleringer, J. R. and Osmond, C. B.: Stable isotopes, in: Plant Physiological Ecology, edited by: Pearcy, R. W., Ehleringer, J. R., Mooney, H. A., and Rundel, P. W., Chapman & Hall, Heidelberg, Germany, 281–300, https://doi.org/10.1007/978-94-009-2221-1_13, 1989.
FAO: The impact of disasters and crises on agriculture and food security, Food and Agriculture Organization of the United Nations, Rome, https://doi.org/10.4060/cb3673en, 2018.
FAO. Proactive approaches to drought preparedness – Where are we now and where do we go from here? Rome, http://www.fao.org/3/ca5794en/ca5794en.pdf (last access: 20 March 2022), 2019.
Gat, J. R.: The isotopes of hydrogen and oxygen, in: Isotope Hydrology: A Study of the Water Cycle, edited by: Kwang Wei, T., Environmental Science and Management, Imperial College Press, London, UK, 9–21, https://doi.org/10.1146/annurev.earth.24.1.225, 2010.
Gehre, M., Geilmann, H., Richter, J., Werner, R. A., and Brand, W. A.: Continuous flow and analysis of water samples with dual inlet precision, Rapid Commun. Mass Sp., 18, 2650–2660, https://doi.org/10.1002/rcm.1672, 2004.
Gilliland, T. J. and Johnston, J.: Barley pea mixtures as cover crops for grass re-seeds, Grass Forage Sci., 47, 1–7, 1992.
Gomiero, T., Pimentel, D., and Paoletti, M. G.: Environmental impact of different agricultural management practices: Conventional vs. organic agriculture, Crit. Rev. Plant Sci., 30, 95–124, https://doi.org/10.1080/07352689.2011.554355, 2011.
Gonfiantini, R., Gratziu, S., and Tongiorgi, E.: Oxygen isotopic composition of water in leaves, in: Isotopes and Radiation in Soil-Plant Nutrition Studies, edited by: IAEA, Vienna, Austria, 405–410, 1965.
Grossiord, C., Sevanto, S., Bonal, D., Borrego, I., Dawson, T. E., Ryan, M., Wang, W. Z., and McDowell, N. G.: Prolonged warming and drought modify belowground interactions for water among coexisting plants, Tree Physiol., 39, 55–63, https://doi.org/10.1093/treephys/tpy080, 2019.
Guderle, M., Bachmann, D., Milcu, A., Gockele, A., Bechmann, M., Fischer, C., Roscher, C., Landais, D., Ravel, O., Devidal, S., Roy, J., Gessler, A., Buchmann, N., Weigelt, A., and Hildebrandt, A.: Dynamic niche partitioning in root water uptake facilitates efficient water use in more diverse grassland plant communities, Funct. Ecol., 32, 214–227, https://doi.org/10.1111/1365-2435.12948, 2018.
Hobbs, P. R., Sayre, K., and Gupta, R.: The role of conservation agriculture in sustainable agriculture, Philos. T. R. Soc. B, 363, 543–555, 2008.
Hoekstra, N. J., Finn, J. A., Hofer, D., and Lüscher, A.: The effect of drought and interspecific interactions on depth of water uptake in deep- and shallow-rooting grassland species as determined by δ18O natural abundance, Biogeosciences, 11, 4493–4506, https://doi.org/10.5194/bg-11-4493-2014, 2014.
Hofer, D., Suter, M., Haughey, E., Finn, J. A., Hoekstra, N. J., Buchmann, N., and Luscher, A.: Yield of temperate forage grassland species is either largely resistant or resilient to experimental summer drought, J. Appl. Ecol., 53, 1023–1034, https://doi.org/10.1111/1365-2664.12694, 2016.
Hothorn, T., Bretz, F., and Westfall, P.: Simultaneous inference in general parametric models, Biometrical J., 50, 346–363, 2008.
IAEA/WMO: Global Network of Isotopes in Precipitation, The GNIP Database, https://nucleus.iaea.org/wiser (last access: 10 January 2021), 2020.
IPCC: Climate change and land. An IPCC special report on climate change, desertification, land degradation, sustainable land management, food security, and greenhouse gas fluxes in terrestrial ecosystems, in press, 2019.
Kashiwagi, J., Krishnamurthy, L., Crouch, J. H., and Serraj, R.: Variability of root length density and its contributions to seed yield in chickpea (Cicer arietinum L.) under terminal drought stress, Field Crops Res., 95, 171–181, https://doi.org/10.1016/j.fcr.2005.02.012, 2006.
Kumar, J., Basu, P. S., Srivastava, E., Chaturvedi, S. K., Nadarajan, N., and Kumar, S.: Phenotyping of traits imparting drought tolerance in lentil, Crop Pasture Sci., 63, 547–554, 2012.
Kundel, D., Bodenhausen, N., Jorgensen, H. B., Truu, J., Birkhofer, K., Hedlund, K., Mader, P., and Fliessbach, A.: Effects of simulated drought on biological soil quality, microbial diversity and yields under long-term conventional and organic agriculture, FEMS Microbiol. Ecol., 96, fiaa205, https://doi.org/10.1093/femsec/fiaa205, 2020.
Kuznetsova, A., Brockhoff, P. B., and Christensen, R. H. B.: lmerTest package: Tests in linear mixed effects models, J. Stat. Softw., 82, 1–26, https://doi.org/10.18637/jss.v082.i13, 2017.
Loaiza Puerta, V., Pereira, E. I. P., Wittwer, R., Heijden, M. V. D., and Six, J.: Improvement of soil structure through organic crop management, conservation tillage and grass-clover ley, Soil Till. Res., 180, 1–9, 2018.
Ma, Y. and Song, X. F.: Using stable isotopes to determine seasonal variations in water uptake of summer maize under different fertilization treatments, Sci. Total Environ., 550, 471–483, https://doi.org/10.1016/j.scitotenv.2016.01.148, 2016.
Ma, Y. and Song, X. F.: Seasonal variations in water uptake patterns of winter wheat under different irrigation and fertilization treatments, Water, 10, 1633, https://doi.org/10.3390/w10111633, 2018.
Martin, R. J. and Jamieson, P. D.: Effect of timing and intensity of drought on the growth and yield of field peas (Pisum sativum L), New Zeal. J. Crop Hort., 24, 167–174, https://doi.org/10.1080/01140671.1996.9513949, 1996.
MeteoSwiss: the Swiss Federal Office of Meteorology and Climatology, IDAWEB, https://www.meteoswiss.admin.ch/home.html?tab=overview, last access: 15 March 2020.
Nippert, J. B. and Knapp, A. K.: Linking water uptake with rooting patterns in grassland species, Oecologia, 153, 261–272, https://doi.org/10.1007/s00442-007-0745-8, 2007.
Ortiz-Bobea, A., Ault, T. R., Carrillo, C. M., Chambers, R. G., and Lobell, D. B.: Anthropogenic climate change has slowed global agricultural productivity growth, Nat. Clim. Change, 11, 306–312, https://doi.org/10.1038/s41558-021-01000-1, 2021.
Parnell, A. C.: simmr: A stable isotope mixing model: https://CRAN.R-project.org/package=simmr (last access: 1 March 2021), 2020.
Parnell, A. C., Phillips, D. L., Bearhop, S., Semmens, B. X., Ward, E. J., Moore, J. W., Jackson, A. L., Grey, J., Kelly, D. J., and Inger, R.: Bayesian stable isotope mixing models, Environmetrics, 24, 387–399, 2013.
Penna, D., Hopp, L., Scandellari, F., Allen, S. T., Benettin, P., Beyer, M., Geris, J., Klaus, J., Marshall, J. D., Schwendenmann, L., Volkmann, T. H. M., von Freyberg, J., Amin, A., Ceperley, N., Engel, M., Frentress, J., Giambastiani, Y., McDonnell, J. J., Zuecco, G., Llorens, P., Siegwolf, R. T. W., Dawson, T. E., and Kirchner, J. W.: Ideas and perspectives: Tracing terrestrial ecosystem water fluxes using hydrogen and oxygen stable isotopes – challenges and opportunities from an interdisciplinary perspective, Biogeosciences, 15, 6399–6415, https://doi.org/10.5194/bg-15-6399-2018, 2018.
Penna, D., Geris, J., Hopp, L., and Scandellari, F.: Water sources for root water uptake: Using stable isotopes of hydrogen and oxygen as a research tool in agricultural and agroforestry systems, Agr. Ecosyst. Environ., 291, 106790, https://doi.org/10.1016/j.agee.2019.106790, 2020.
Prechsl, U. E., Gilgen, A. K., Kahmen, A., and Buchmann, N.: Reliability and quality of water isotope data collected with a lowbudget rain collector, Rapid Commun. Mass Sp., 28, 879–885, https://doi.org/10.1002/rcm.6852, 2014.
Prechsl, U. E., Burri, S., Gilgen, A. K., Kahmen, A., and Buchmann, N.: No shift to a deeper water uptake depth in response to summer drought of two lowland and sub-alpine C3-grasslands in Switzerland, Oecologia, 177, 97–111, https://doi.org/10.1007/s00442-014-3092-6, 2015.
Purushothaman, R., Krishnamurthy, L., Upadhyaya, H. D., Vadez, V., and Varshney, R. K.: Genotypic variation in soil water use and root distribution and their implications for drought tolerance in chickpea, Funct. Plant Biol., 44, 235–252, https://doi.org/10.1071/Fp16154, 2017.
Querejeta, J. I., Ren, W., and Prieto, I.: Vertical decoupling of soil nutrients and water under climate warming reduces plant cumulative nutrient uptake, water-use efficiency and productivity, New Phytol., 230, 1378–1393, https://doi.org/10.1111/nph.17258, 2021.
Rasmussen, C. R., Thorup-Kristensen, K., and Dresboll, D. B.: Uptake of subsoil water below 2 m fails to alleviate drought response in deep-rooted Chicory (Cichorium intybus L.), Plant Soil, 446, 275–290, 2020.
R Core Team: R: A language and environment for statistical computing, R Foundation for Statistical Computing, Vienna, Austria, https://www.R-project.org/ (last access: 20 March 2022), 2020.
Rothfuss, Y. and Javaux, M.: Reviews and syntheses: Isotopic approaches to quantify root water uptake: a review and comparison of methods, Biogeosciences, 14, 2199–2224, https://doi.org/10.5194/bg-14-2199-2017, 2017.
Schewe, J., Heinke, J., Gerten, D., Haddeland, I., Arnell, N. W., Clark, D. B., Dankers, R., Eisner, S., Fekete, B. M., Colon-Gonzalez, F. J., Gosling, S. N., Kim, H., Liu, X. C., Masaki, Y., Portmann, F. T., Satoh, Y., Stacke, T., Tang, Q. H., Wada, Y., Wisser, D., Albrecht, T., Frieler, K., Piontek, F., Warszawski, L., and Kabat, P.: Multimodel assessment of water scarcity under climate change, P. Natl. Acad. Sci. USA, 111, 3245–3250, 2014.
Schluter, S., Grossmann, C., Diel, J., Wu, G. M., Tischer, S., Deubel, A., and Rucknagel, J.: Long-term effects of conventional and reduced tillage on soil structure, soil ecological and soil hydraulic properties, Geoderma, 332, 10–19, https://doi.org/10.1016/j.geoderma.2018.07.001, 2018.
Seitz, S., Goebes, P., Puerta, V. L., Pereira, E. I. P., Wittwer, R., Six, J., van der Heijden, M. G. A., and Scholten, T.: Conservation tillage and organic farming reduce soil erosion, Agron. Sustain. Dev., 39, 4, https://doi.org/10.1007/s13593-018-0545-z, 2019.
Silvertown, J., Araya, Y., and Gowing, D.: Hydrological niches in terrestrial plant communities: A review, J. Ecol., 103, 93–108, https://doi.org/10.1111/1365-2745.12332, 2015.
Kanton Zürich: Soil map of the agricultural areas: http://maps.zh.ch/?topic=BoKaZH, last access: 25 November 2020.
Sprenger, M., Leistert, H., Gimbel, K., and Weiler, M.: Illuminating hydrological processes at the soil-vegetation-atmosphere interface with water stable isotopes, Rev. Geophys., 54, 674–704, https://doi.org/10.1002/2015rg000515, 2016.
Sun, Q: Water uptake patterns of pea and barley responded to drought but not to cropping systems, ETH Zurich Research Collection [data set], https://doi.org/10.3929/ethz-b-000533193, 2022.
Sun, Q., Gilgen, A. K., Signarbieux, C., Klaus, V. H., and Buchmann, N.: Cropping systems alter hydraulic traits of barley but not pea grown in mixture, Plant Cell Environ., 44, 2912–2924, https://doi.org/10.1111/pce.14054, 2021.
Swiss Federal Council: Verordnung über die Direktzahlungen an die Landwirtschaft (Direktzahlungsverordnung, DZV) vom 23. Oktober 2013 (Stand am 1 Januar 2021) [Swiss council regulation no. 910.13: Ordinance on Direct Payments of 23 October 2013 (as of 1 January 2021)], https://www.fedlex.admin.ch/eli/cc/2013/765/de, last access: 19 February 2021.
Teasdale, J. R., Coffman, C. B., and Mangum, R. W.: Potential long-term benefits of no-tillage and organic cropping systems for grain production and soil improvement, Agron. J., 99, 1297–1305, 2007.
Thorup-Kristensen, K., Halberg, N., Nicolaisen, M., Olesen, J. E., Crews, T. E., Hinsinger, P., Kirkegaard, J., Pierret, A., and Dresboll, D. B.: Digging deeper for agricultural resources, the value of deep rooting, Trends Plant Sci., 25, 406–417, https://doi.org/10.1016/j.tplants.2019.12.007, 2020.
von Freyberg, J., Allen, S. T., Grossiord, C., Dawson, T. E., and Royles, J.: Plant and root-zone water isotopes are difficult to measure, explain, and predict: Some practical recommendations for determining plant water sources, Methods Ecol. Evol., 11, 1352–1367, https://doi.org/10.1111/2041-210x.13461, 2020.
Wahdan, S. F. M., Reitz, T., Heintz-Buschart, A., Schadler, M., Roscher, C., Breitkreuz, C., Schnabel, B., Purahong, W., and Buscot, F.: Organic agricultural practice enhances arbuscular mycorrhizal symbiosis in correspondence to soil warming and altered precipitation patterns, Environ. Microbiol., 23, 6163–6176, 2021.
Wang, L. X., Caylor, K. K., Villegas, J. C., Barron-Gafford, G. A., Breshears, D. D., and Huxman, T. E.: Partitioning evapotranspiration across gradients of woody plant cover: Assessment of a stable isotope technique, Geophys. Res. Lett., 37, L09401, https://doi.org/10.1029/2010gl043228, 2010.
Wang, L. X., Manzoni, S., Ravi, S., Riveros-Iregui, D., and Caylor, K.: Dynamic interactions of ecohydrological and biogeochemical processes in water-limited systems, Ecosphere, 6, 133, https://doi.org/10.1890/Es15-00122.1, 2015.
Werner, R. A., Bruch, B. A., and Brand, W. A.: ConFlo III – An interface for high precision δ13C and δ15N analysis with an extended dynamic range, Rapid Commun. Mass Sp., 13, 1237–1241, 1999.
Whitmore, A. P. and Whalley, W. R.: Physical effects of soil drying on roots and crop growth, J. Exp. Bot., 60, 2845–2857, 2009.
Wittwer, R. A., Dorn, B., Jossi, W., and van der Heijden, M. G. A.: Cover crops support ecological intensification of arable cropping systems, Sci. Rep.-UK, 7, 41911, https://doi.org/10.1038/srep41911, 2017.
Wu, H. W., Li, X. Y., Li, J., Jiang, Z. Y., Chen, H. Y., Ma, Y. J., and Huang, Y. M.: Differential soil moisture pulse uptake by coexisting plants in an alpine Achnatherum splendens grassland community, Environ. Earth Sci., 75, 914, https://doi.org/10.1007/s12665-016-5694-2, 2016.
Wu, H. W., Li, J., Zhang, C. C., He, B., Zhang, H. X., Wu, X. C., and Li, X. Y.: Determining root water uptake of two alpine crops in a rainfed cropland in the Qinghai Lake watershed: first assessment using stable isotopes analysis, Field Crops Res., 215, 113–121, https://doi.org/10.1016/j.fcr.2017.10.011, 2018.
Zegada-Lizarazu, W. and Iijima, M.: Hydrogen stable isotope analysis of water acquisition ability of deep roots and hydraulic lift in sixteen food crop species, Plant Prod. Sci., 7, 427–434, 2004.
Zegada-Lizarazu, W., Kanyomeka, L., Izumi, Y., and Iijima, M.: Pearl millet developed deep roots and changed water sources by competition with intercropped cowpea in the semiarid environment of northern Namibia, Plant Prod. Sci., 9, 355–363, 2006.
Zimmermann, U., Ehhalt, D., and Münnich, K. O.: Soil-water movement and evapotranspiration: Changes in the isotopic composition of the water, Isotopes in hydrology, Proceedings of a symposium, International Atomic Energy Agency (IAEA), 38061083, International Nuclear, Vol. 38, 1967.