the Creative Commons Attribution 4.0 License.
the Creative Commons Attribution 4.0 License.
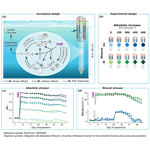
Early life stages of fish under ocean alkalinity enhancement in coastal plankton communities
Silvan Urs Goldenberg
Ulf Riebesell
Daniel Brüggemann
Gregor Börner
Michael Sswat
Arild Folkvord
Maria Couret
Synne Spjelkavik
Nicolás Sánchez
Cornelia Jaspers
Marta Moyano
Ocean alkalinity enhancement (OAE) stands as a promising carbon dioxide removal technology. Yet, this solution to climate change entails shifts in environmental drivers with unknown consequences for marine fish that are critical to ecosystem health and food security. Fish and their supporting food webs may be stressed by the novel carbonate chemistry or the nutrients contained in the deployed minerals. With a mesocosm experiment on natural plankton communities, we studied early life stages of fish under alkalinity (+600 µmol kg−1) and silicate (+75 µmol L−1) addition. Larvae and young juveniles of temperate coastal species, including Atlantic herring (Clupea harengus) and cod (Gadus morhua), were exposed to direct physiological and indirect food-web-mediated effects of OAE for 49 d. Neither in the shorter nor in the longer term did we find an impairment of fish growth and survival. Alkalization even led to an increase in fish biomass. This resistance to OAE was despite using non-CO2-equilibrated deployment that induces more severe perturbations in carbonate chemistry (ΔpH , pCO2=75 µatm) compared to alternative scenarios. Overall, our community-level study suggests that some fish populations, including key fisheries' species, may be resilient to the water chemistry changes under OAE. Whilst these results give cause for optimism regarding the large-scale application of OAE, other life history stages (embryos) and habitats (open ocean) may prove more vulnerable.
- Article
(2473 KB) - Full-text XML
-
Supplement
(909 KB) - BibTeX
- EndNote
Ocean alkalinity enhancement (OAE) is being proposed as a carbon dioxide removal technology to help limit global warming to 1.5 °C (Renforth and Henderson, 2017; Rogelj et al., 2018). This ocean-based solution can be implemented through the acceleration of a natural process – rock weathering – where alkaline minerals are dissolved in seawater. As alkalinity increases, so does the capacity of seawater to take up CO2 from the atmosphere. The sequestration may be scalable, safe and cost-effective (Lackner, 2002). Besides removing carbon to combat climate change, this approach would also counter ocean acidification, which is widely recognized as major threat to marine life (Doney et al., 2020). While OAE indeed seems promising from a technological and economic perspective, an understanding of the potential environmental risks and side effects is lacking (Gattuso et al., 2021; Nawaz et al., 2023; Bach et al., 2019). Research is needed to evaluate the ecosystem safety of OAE and guide its potential large-scale implementation.
Biological processes are not affected by alkalinity itself but through the associated changes in various ions and molecules (Bach et al., 2019). Added alkalinity reduces CO2 as a carbon source for primary producers (Hansen, 2002), increases calcium carbonate saturation which facilitates calcification (Renforth and Henderson, 2017) and increases pH concerning the acid–base balance of organisms (Tresguerres et al., 2020; Pörtner, 2008). Applied at stationary facilities or on ships, the perturbation would initially be localized. A pH of up to 9 may be reached (Bach et al., 2019; Hartmann et al., 2023). Over time, this “OAE plume” would mix with surrounding water masses and absorb CO2 from the atmosphere (equilibration), alleviating the perturbation (He and Tyka, 2023). Therefore, whilst at a global scale, sequestering gigatons of CO2 involves minor changes in water chemistry (Renforth and Henderson, 2017), species communities at deployment sites could experience OAE as a stressor. In this sense, studying coastal regions is particularly relevant. They are not only most attractive economically for OAE deployment given the proximity to mineral and energy sources (He and Tyka, 2023) but also hotspots of biodiversity and ecosystem services.
Fishes are essential for the stability of coastal ecosystems and global food security (FAO, 2022). Physiological processes in fish are highly sensitive to the concentrations of bicarbonate, CO2 and H+ (Tresguerres et al., 2020), which are all altered under OAE. To maintain homeostasis of body fluids and proper functioning of cells and tissues in variable environments, fish have evolved a sophisticated acid–base regulation (Perry and Gilmour, 2006). They are hence thought to cope well with mild changes in pH (i.e., H+ concentration) and to be generally less sensitive than marine invertebrates (Melzner et al., 2009). Whether fishes can still compensate under scenarios of OAE that far exceed natural pH variability (Bach et al., 2019; Carstensen and Duarte, 2019; Hofmann et al., 2011) is, however, uncertain. There could be additional energetic costs for acid–base regulation that channel resources away from growth and reproduction or pH thresholds beyond which physiological functions fail and threaten population viability.
The related field of ocean acidification has taught us several lessons on the pH sensitivity of fishes. While they were mostly found to be robust to acidification, some ecosystems, species, life stages and traits were surprisingly vulnerable (Cattano et al., 2018; Esbaugh, 2018; Nagelkerken and Connell, 2015). Physiological and behavioral impacts were observed related to metabolic rate, otolith calcification, sensory functioning, lateralization, swimming, foraging, predator avoidance and habitat selection. An OAE plume could entail a similarly large change in H+ concentration, albeit in the opposite direction, to that which may impair fishes under end-of-century acidification (Bach et al., 2019; Hartmann et al., 2023). Unlike for global acidification, however, the exposure of fishes to OAE would be abrupt and spatially inconsistent, lowering the chances for phenotypic buffering and genetic adaptation (Sunday et al., 2014; Wong and Candolin, 2015; Blewett et al., 2022). An investigation into the shorter-term responses of fishes to OAE is thus needed.
OAE may prove particularly challenging for early life stages, a bottleneck for most fish populations. At the organism level, larvae are highly sensitive to their abiotic environment (Melzner et al., 2009; Cattano et al., 2018) but lack the locomotory capacity to avoid unfavorable water masses. This may facilitate direct physiological effects of OAE (Brownell, 1980; Parra and Yufera, 2002). At the community level, larvae are tightly controlled by resources, competition and predation (Houde, 2008). OAE could change these food web interactions, for example via species-specific pH sensitivities, expanding calcifiers or CO2 limited primary production, giving rise to indirect effects (Ockendon et al., 2014; Goldenberg et al., 2018). Only by combining these different ecological scales will we achieve a mechanistic understanding of impacts on larvae growth and survival and ultimately recruitment success (Fig. 1a).
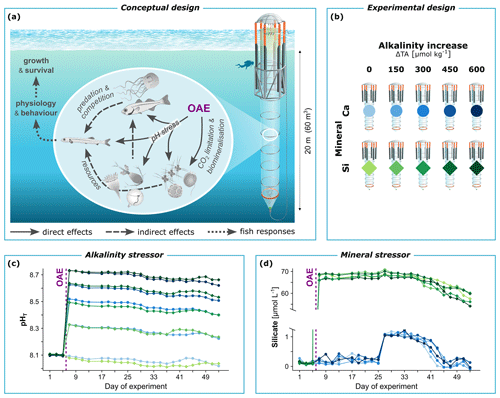
Figure 1Conceptual and methodological framework of our mesocosm study on non-CO2-equilibrated OAE. (a) Potential pathways of change in fish and natural plankton communities. (b) Water chemistry manipulations to simulate different scenarios of OAE. Using 10 mesocosm units, we tested increases in total alkalinity (ΔTA) under calcium-based (Ca) or silicate-based (Si) mineral addition. (c) OAE-induced shifts in pH and (d) silicate availability, as measured in each mesocosm throughout the experimental period. Mesocosm symbol from Rita Erven, GEOMAR, and organism symbols partly from Integration and Application Network, University of Maryland Center for Environmental Science (https://ian.umces.edu/symbols/, last access: 10 December 2023).
Source minerals for OAE can introduce additional elements that may act as stimulators or stressors for fish. These include trace metals like iron and nickel (Morel and Price, 2003) but also macronutrients (Bach et al., 2019). The application of silicate minerals would, for instance, increase silicate concentrations (Si(OH)4) in seawater well above natural levels. This macronutrient favors silicifying diatoms over other primary producers (Sarthou et al., 2005) with consequences for food web functioning (Sommer et al., 2002). On the one hand, the larger size of diatoms can make primary productivity directly accessible to copepods (Hansen et al., 1994), a primary food source for many fish larvae (Turner, 1984). On the other hand, strong silica cell walls (Pancic et al., 2019), toxic secondary metabolites (Ianora and Miralto, 2010) and low nitrogen content (Goldenberg et al., 2024a) may reduce diatom palatability and nutritional value. Silicate minerals may hence cause changes at the bottom of the food web with either positive or negative knock-on effects for planktivorous fish.
Here, we study the sensitivity of early life stages of fish to OAE-induced increases in alkalinity and silicate. Direct physiological as well as indirect food-web-mediated effects were considered (Fig. 1a). For this, large-scale mesocosms were employed in coastal waters of temperate western Norway during the main spawning season in spring. They enclosed natural plankton communities including larvae and young juveniles of major fisheries' species such as Atlantic herring (Clupea harengus) and Atlantic cod (Gadus morhua) (FAO, 2022). Alkalinity was applied non-CO2-equilibrated with up to 600 µmol kg−1 of added alkalinity, entailing strong perturbations in pHT (increase by 0.7 units) and pCO2 (decrease by 325 µatm) (Fig. 1c). A calcium-based mineral treatment served as the baseline to establish the effects of extreme silicate availability (Fig. 1d). This intense yet realistic employment scenario (Bach et al., 2019; Hartmann et al., 2023; Renforth and Henderson, 2017) may reveal the maximum impact potential of OAE and facilitate the identification of response pathways in fish. Our findings on the resilience of fish help establish an environmentally safe operating space of OAE for carbon dioxide removal.
2.1 Study system
The experiment took place in Raunefjord near Bergen, Norway (60°15′55′′ N, 5°12′21′′ E). This temperate mesotrophic region is characterized by a seasonal plankton succession, with both calcifying and silicifying primary producers (Paulino et al., 2018). Here, spawning in many fish species coincides with the spring bloom. The subsequent survival of larvae and their growth into juveniles are vital for a balanced ecosystem and profitable fisheries. We studied the local assemblage of planktonic larvae, comprised of various demersal and pelagic fish species. A particular focus was put on Atlantic herring. This small pelagic fish supports the largest fishery in Norway and the fourth-largest in the world, with an annual yield of ∼ 1.7 Mt (Fao, 2022). As such, it holds a key role as a trophic intermediary in transferring energy from zooplankton to larger predators (Toresen et al., 2019). Herring larvae had served us as a model organism during past laboratory (Sswat et al., 2018a; Maneja et al., 2015) and mesocosm work on ocean acidification (Spisla et al., 2022; Sswat et al., 2018b). These studies suggested a certain tolerance of herring to pH decreases that already impacted larvae of other species such as cod (Stiasny et al., 2016).
On 7 May 2022, 10 floating KOSMOS mesocosms (Riebesell et al., 2013) were moored from RV ALKOR nearby Espegrend research station at a bottom depth of ∼ 60 m (map in Spisla et al. 2022). The units consisted of 20 m long and 2 m wide transparent polyurethane bags that terminated in a 2 m long sediment trap (Fig. 1a). The lower end of the bags was extended to depth, while open at the bottom, to enclose ∼ 60 m3 of seawater including any planktonic organisms present. Then, the bottom was closed, isolating the bags from the surrounding fjord water and marking the start of the experiment (day 0). Wall growth was removed regularly using brushes from the outside and a cleaning ring from the inside. Environmental conditions were assessed with CTD (conductivity, temperature and depth) casts in 2 d intervals. Average salinity was ∼ 33, and photosynthetically active radiation was reduced to ∼ 30 % of surface radiation. Oxygen saturation stayed consistently above 100 %. Temperature increased from 8.8 to 15.4 °C over the course of spring. These physicochemical conditions varied across the stratified water column from surface to depth.
A diverse, multi-trophic plankton food web was enclosed in the mesocosms. Larger organisms were removed at the start using a net with a mesh size of 3 mm that spanned the width of the mesocosms. The resulting community comprised of primary producers (e.g., diatoms and coccolithophores), grazers (e.g., copepods) and predators (e.g., jellyfish) as in previous experiments at this locality (Schulz et al., 2017; Spisla et al., 2022). Larvae of various fish species were also present including codfishes (mostly Atlantic cod), gobies (mostly Pomatoschistus spp.) and flatfishes (mostly Limanda spp. and Microstomus spp.). On day 6, these naturally occurring larvae were supplemented with 95 laboratory-reared herring larvae per mesocosm (25 d post-hatch, 15 ± 1 mm). Prior to their introduction, herring had either been absent or at low abundance according to our monitoring of deceased fish via the sediment trap. By the end of the experiment, the fish assemblage consisted of a mixture of larvae and young juveniles.
Primary production in the mesocosms was initially low as the experiment began shortly after the spring bloom with little inorganic nutrients remaining (Ferderer et al., 2024). To also consider bloom conditions in our study of OAE, nutrients were added on day 25 to all mesocosms, leading to an increase in nitrate, phosphate and silicate of 3.8 ± 0.2, 0.20 ± 0.01 and 0.98 ± 0.06 µmol L−1, respectively. During past experiments, this level of fertilization induced phytoplankton blooms in mesocosms (e.g., Schulz et al., 2017) that corresponded in intensity to those observed in the natural environment (Paulino et al., 2018).
2.2 Herring larvae rearing
The herring larvae had been reared under ambient conditions (i.e., without OAE) in the laboratories at the nearby University of Bergen. Following Folkvord et al. (2020), two wild-caught females (∼ 356 g in size) and males (∼ 297 g) were strip-spawned onto plastic plates in two crosses. Fertilization success was 92 %. Eggs were incubated at 7.1 ± 0.1 °C and a salinity of 33.4 ± 0.1 (mean ± SD). After hatching 17 d later (24 April), a total of 2400 larvae were distributed amongst two ∼ 500 L rearing tanks. These were filled with water pumped from the fjord and aerated with ambient air. Temperature was continuously adjusted to match that of the outside fjord and averaged 6.5 ± 0.8 °C. Oxygen levels were 87.8 ± 4.7 %, salinity 33.5 ± 0.3, total alkalinity 2523 ± 6 µmol kg−1 and pHNBS 7.94 ± 0.09. Artificial light mimicked the local diurnal light cycle. The larvae were fed ad libitum with a diverse assemblage of zooplankton including nauplii and copepodites, regularly collected from the nearby fjord (Folkvord et al., 2015). Prey densities were closely monitored in the tanks and restocked daily to maintain high levels (2000 prey L−1). Algae cultures were also added daily (Rhodomonas sp. and Isochrysis sp.). Over the rearing period, larval survival was estimated at 99.8 %.
2.3 OAE application
On day 6, we established a five-step gradient in alkalinity increase (ΔTA) from 0 to 600 µmol kg−1, with half of the mesocosms simulating calcium-based (Ca) and the other half silicate-based (Si) mineral addition (Fig. 1b). The Ca-based treatment only introduced elements that are already abundant in seawater and were thus unlikely to impact marine life beyond the alkalinity effect. It hence served as the baseline to establish the effect of excess silicate. ΔTA was applied non-CO2-equilibrated (Bach et al., 2019). This economically more feasible approach only adds alkalinity, and CO2 sequestration occurs afterwards through natural equilibration with the atmosphere. In the alternative pre-CO2-equilibrated approach, the to-be-sequestered CO2 would instead be added together with the alkalinity, leading to milder changes in seawater chemistry. We restricted ΔTA to 600 µmol kg−1 to avoid abiotic precipitation of calcium carbonate, which would signify a loss in alkalinity and thus a nonsensical OAE scenario (Hartmann et al., 2023). The gradient design with non-replicated treatment levels (Riebesell et al., 2023) was preferred for ΔTA to allow for a more informative study of biogeochemical processes that were also part of the multidisciplinary mesocosm project (e.g., Ferderer et al., 2024). For analysis, ΔTA could then be tested as a continuous explanatory variable in the sense of linear regression.
The OAE application followed the principles in Riebesell et al. (2023). NaOH was used as an alkalizer with a proportional amount of CaCl2 to simulate quick or hydrated lime (Ca-based) and MgCl2 to simulate olivine (Si-based). Silicate was added in equal amounts of 75 µmol L−1 to all five Si-based mesocosms using Na2SiO3. This allowed us to separate the effects of alkalinity and silicate and prevented mineral precipitation (Ferderer et al., 2024). These salts were dissolved in 40 L Milli-Q water per mesocosm and then mixed into the mesocosms using a special distribution device referred to as the “spider” (Riebesell et al., 2013).
Carbonate chemistry and inorganic nutrients were monitored in 2 d intervals based on depth-integrated water samples. For this, samplers equipped with a pressure-controlled motor (5 L, Hydro-Bios, Kiel, Germany) were lowered from the surface to the bottom of the mesocosms to collect water evenly across the water column. The resulting samples represented mesocosm averages. In the laboratory, water was first sterile-filtered using a 0.2 µm pore size polyethersulfone membrane. Carbonate chemistry was assessed following Schulz et al. (2023). TA was measured via titration (Metrohm 862 Compact Titrosampler with Aquatrode Plus with PT1000) calibrated against certified reference material (CRM batch 193) supplied by Andrew Dickson's laboratory, and pH was measured spectrophotometrically (Dickson et al., 2007). With the temperature and salinity provided by the CTD casts, in situ pH and pCO2 could then be calculated using CO2SYS for Excel with constants from Lueker et al. (2000) (Pierrot et al., 2021). Silicate was determined spectrophotometrically following Hansen and Koroleff (1999).
Our OAE treatments produced the intended water chemistry changes. TA increased in a clean gradient (Fig. S1a), followed by the expected increases in pH (Fig. 1c) and ΩCa (from 3.2 ± 0.2 to 11.7 ± 0.2) and decreases in pCO2 (Fig. S1b). These OAE-induced shifts in carbonate chemistry were present across depth (as exemplified via pH, Fig. S1c). CO2 equilibration with the atmosphere was minimal throughout the experimental period given the large depth and stratified water column of the mesocosms. Silicate availability in the Si-based mineral treatments stayed in extreme excess between 55 and 70 µmol L−1, while the Ca-based treatments remained well below 1 µmol L−1 for most of the experiment, suggesting silicate limitation (Fig. 1d). In conclusion, carbonate chemistry and silicate perturbations were relatively stable over 49 d of OAE, optimal conditions for the emergence of food web effects and their propagation to fish.
2.4 Biological variables
We monitored the fish assemblage closely. Mortality through processes other than predation was tracked over the entire experiment. For this, the sediment trap was sampled in 2 d intervals via a tube connected to the surface (Fig. 1a) and immediately screened for dead fish. Carcasses were assigned to either herring, codfishes or flatfishes based on their distinct body shape. This method had proven successful in previous campaigns, especially in colder climates where fish carcasses disintegrate slowly (Spisla et al., 2022). At the end of the experiment, all live fish were caught with a net (1 mm mesh) spanning the width of the mesocosm. Fish were anesthetized (0.1 g L−1) followed by euthanizing (overdose) with MS222 and preserved at −80 °C. Finally, each individual fish was weighed for wet mass. Predation on herring was estimated as the difference between the individuals initially introduced and the ones found later in the sediment trap and final sampling.
The wider plankton community was also monitored to better understand potential indirect effects on fish. Primary producer biomass and photosynthetic capacity was approximated every 2 d via chlorophyll a. For this, depth-integrated water samples were filtered and analyzed on a fluorometer (Ferderer et al., 2024). Metazoan zooplankton was sampled every 4 d across the entire water column using a net with 55 µm mesh size (Apstein, ∅ 17 cm) (Spisla et al., 2022). After preservation in 70 % ethanol, copepods (main larval prey) and hydrozoans (potential larval competitors and predators) were counted under the microscope.
2.5 Data analysis
To assess the responses of fish and other functional groups to OAE, replicate measures within mesocosms (i.e., individuals or sampling days) were first summed or averaged. This provided one independent measure per mesocosm. Per-capita size of fish had been log10-transformed to reduce the influence of large but rare individuals on the calculated average. The subsequent analyses were conducted at a significance level of α=0.05 with R version 4.0.5 (R Core Team, 2021).
For univariate responses, linear models were employed with ΔTA (continuous), mineral (categorical) and their interaction (ΔTA × mineral) as explanatory variables (type III test). Unexplained variability was particularly high at the level of single taxa (Fig. S2), likely due to random differences in starting numbers and sizes. We therefore restricted our main analyses to the whole fish assemblage. Here, across all fishes, some of the variability was compensated for by competition for limited food resources. Model assumptions were addressed following Lüdecke et al. (2021). Normality of residuals was checked with normal Q–Q plots and homogeneity of variance with residual–fitted plots.
Potential shifts in the taxonomic composition of the fish assemblage were assessed using multivariate analyses based on Bray–Curtis dissimilarity (vegan package; Oksanen et al., 2022). Differences in composition between mesocosms were illustrated via non-metric multidimensional scaling (nMDS). Mantel tests with Pearson correlation were employed to test for ΔTA and permutational multivariate analysis of variance (adonis2 function) to test for mineral based on 999 permutations.
Our study of fish in natural plankton communities considered changes in both water chemistry and food web interactions. We were nevertheless unable to detect negative impacts of OAE, neither via increased alkalinity nor via a silicate-based mineral addition. The fish assemblages were comprised of larvae and young juveniles of different coastal species, including the introduced herring and naturally enclosed species such as Atlantic cod.
During the experiment, fish mortality by processes other than predation was monitored via carcasses that had sunk to the sediment trap. We found this mortality to be throughout unrelated to OAE. The abrupt changes in carbonate chemistry following OAE application were not lethal to any of the species (Fig. 2a, left), including herring (Fig. 2b). These results indicate that elevated pH did not impair physiology at the most basic level that leads to an immediate demise. Surviving the first shock, the fishes now had the chance to adjust to the novel water chemistry. At the end of our experiment, mortality still remained unaffected by OAE (Fig. 2a, right), and species-specific effects were not apparent (Fig. 2c). Hence, there was no sign of physiological or behavioral disruption that rendered fish incapable of foraging for food causing starvation. It should be noted that the unusually high mortality rates in two mesocosms (Ca ΔTA 300 and Si ΔTA 150, Fig. 2a, b) were most likely caused by flappy walls that harmed some larvae during wave action.
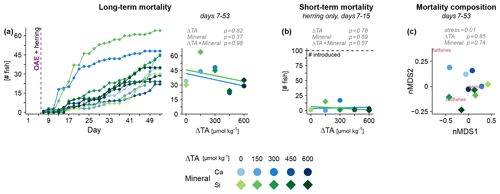
Figure 2Fish mortality under OAE monitored via the sediment trap. (a) Cumulative mortality over time across all species and (b) immediately following the OAE perturbation for herring. (c) Differences in taxonomic composition between mesocosms via non-metric multidimensional scaling (nMDS). Statistical tests in gray (details in Table S1).
We next assessed the assemblage of live fish at the end of the 49 d experiment. Also here, our OAE manipulations of varying alkalinity and mineral did not emerge as dominant stressors for fish. Neither total abundances (Fig. 3a) nor taxonomic composition (Fig. 3b) provided evidence for an effect of OAE. Besides physiological stress and starvation, abundances were also a product of predation by other fish and invertebrate predators. At this point, we have to acknowledge the high variability in some of the data, especially at the level of individual fish species, which may have concealed less severe yet ecologically relevant impacts. The only significant effect in our study was the increase in total fish biomass under alkalization (Fig. 3d). Average fish size showed a similar, albeit non-significant, pattern (Fig. 3c). This positive effect of alkalinity seemed to be mostly driven by one taxon, the codfishes (Fig. S2). In contrast, the high availability of silicate under Si-based OAE seemed of no consequence for the fish assemblage, despite its potential to cause imbalances at the bottom of the food web.
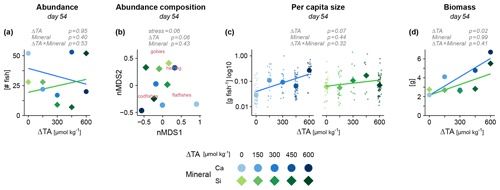
Figure 3Fish growth and survival under OAE, assessed via the assemblage of live fish at the end of the experiment. (a) Abundance, (b) individual size and (d) total biomass across all fish taxa. (c) Differences in taxonomic composition between mesocosms via non-metric multidimensional scaling (nMDS). Larger points represent mesocosms and smaller points in (c) single individuals. Statistical tests in gray (details in Table S1).
Lastly, we assessed functional groups of the plankton food web that have the potential to propagate indirect effects of OAE to fish. Major changes in lower trophic levels due to alkalinity increase or elevated silicate were not apparent, considering primary producers (chlorophyll a, Fig. 4a) and the main prey of fish larvae (copepod zooplankton, Fig. 4b). Invertebrate predators (jellyfish) that compete with fish for food and may prey on small larvae seemed also unaffected (Fig. 4c). There was no sign of an altered predation rate on herring (Fig. 4d), an estimate that also includes the potential predation from larger piscivorous fish present in the mesocosms (e.g., cod juveniles). Overall, we found no evidence for an increased bottom-up or reduced top-down forcing that could explain the positive effect of alkalinity on fish biomass.
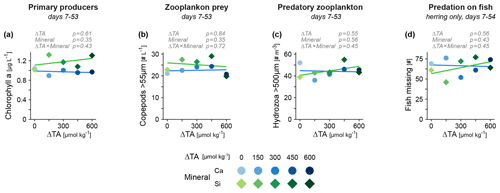
Figure 4Potential sources of indirect effects of OAE on fish mediated via species interactions. Abundance of other functional groups including (a) primary producers, (b) invertebrate grazers and (c) invertebrate predators. (d) Predation on herring estimated via missing individuals. Averages across the treatment period are tested (in gray, details in Table S2).
Our study suggests that larvae and juveniles of some temperate coastal fishes may be viable under ocean alkalinity enhancement (OAE) for carbon dioxide removal. Negative impacts of alkalinity and silicate were not apparent, neither in the shorter nor the longer term. Early life stages from these fish populations may recruit successfully and continue to support fisheries in regions of OAE deployment.
During the first days of OAE exposure in our mesocosms, fish mortality was not elevated, suggesting an absence of severe direct effects on their physiology. In the local ecosystem, natural pH variability peaks at ∼ 8.35 (Omar et al., 2016) and thus remains well below the ∼ 8.7 of our highest alkalinity scenario. The fishes were hence challenged by an instantaneous pH increase that considerably exceeded what their populations should have been pre-adapted to in this system. The ability to compensate despite the severity and abruptness of the perturbation confirms a powerful machinery for acid–base regulation in these vertebrates (Tresguerres et al., 2020). This result may have been expected for herring based on its tolerance to moderate levels of the opposing stressor ocean acidification (Sswat et al., 2018a; Maneja et al., 2015; Franke and Clemmesen, 2011). However, with cod that can experience high larvae mortality under moderate acidification (Stiasny et al., 2016), we also included a supposedly more pH-sensitive species. Interestingly, both species – herring and cod – can develop major organ damage at more extreme levels of acidification (Frommel et al., 2012, 2014). Our study on OAE could have missed minor damage that did not pose an immediate threat to survival. Other life history stages may prove more vulnerable to OAE, in particular reproductive cells and early embryos that lack specialized regulatory tissue (Melzner et al., 2009; Dahlke et al., 2020).
Ecosystems and fish species may differ in their sensitivity to alkalization. We only know of two comprehensive studies on the viability of fish larvae under elevated pH (Brownell, 1980; Parra and Yufera, 2002). These investigated five species in an aquaculture context. After only 24 h exposure to pH above ∼ 8.5, the authors reported reduced first-feeding success and, in one species, even lethality. Interestingly, high pH was considerably more detrimental than low pH (Brownell, 1980; Parra and Yufera, 2002), further emphasizing the impact potential of OAE. Given these acute sensitivities that contrast our finding of tolerance, we may infer a species-specific effect of increased pH on fish larvae. OAE could be more stressful for fishes that have adapted to stable environmental conditions, such as in the open ocean (Blewett et al., 2022; Hofmann et al., 2011), or for fishes that are already challenged physiologically, like in warm, tropical waters (Vinagre et al., 2016; Pörtner, 2008). Our results on temperate coastal species are clearly only a first step towards assessing the safety of OAE.
Once fish survive the water chemistry perturbation itself, time is given for the food-web-mediated impacts of OAE. Several mechanisms were proposed through which alkalinity may lower the quantity and quality of prey for higher trophic levels. These include slowed primary production (Hansen, 2002), proliferation of heavily armored calcifiers (Renforth and Henderson, 2017) and pH stress in invertebrate prey (Melzner et al., 2009). Yet, in our mesocosms, we could not find evidence for these indirect effects on fish, not even after longer-term exposure. However, what we did observe was a positive effect of alkalization on fish biomass. This result was not easily explained through major changes elsewhere in the community. Neither prey availability was enhanced nor the potential for competition with or predation by jellyfish reduced. Altered intraguild predation – larger fish eating smaller ones – seemed not to be responsible either. The biogeochemical perspective including inorganic nutrients and export of matter (Ferderer et al., 2024) also provided no clues as to how alkalization may have facilitated the build-up of fish biomass. With that, we explored the more obvious bottom-up and top-down processes that can propagate environmental change to fish (Sswat et al., 2018b; Goldenberg et al., 2017; Spisla et al., 2022). Overall, it appears that our alkalinity manipulation did not cause major ecosystem shifts.
Addition of silicate mineral, in contrast, induced strong responses in our primary producers, as presented in Ferderer et al. (2024). The authors observed an increase in diatom cell silicification and a prominence of their shells in suspended matter. Other studies on silicate enrichment in Norway even described diatoms as low in nutritional value (Gilpin et al., 2004; Davidson et al., 2002) and poor food for zooplankton (Nejstgaard et al., 2001). It is thus surprising that the excess silicate in our mesocosms did not indirectly impact copepod grazers and their fish predators. This finding emphasizes the complexity of species interactions that will require careful consideration in the emerging research field of OAE.
Whilst our study may have overseen more subtle responses, the fishes appeared able to maintain basic physiological and ecological capabilities under OAE. This is a significant first step towards the classification of OAE as a stressor, since under these circumstances fish populations are given an opportunity to adjust. At the organismal level, individuals may avoid the OAE plume, acclimate physiologically (Pörtner, 2008) or simply persevere until the conditions improve. The perturbation will be alleviated with time through mixing with surrounding water masses (He and Tyka, 2023), CO2 equilibration (Bach et al., 2019), and the removal of nutrients and trace metals via the biological pump (Morel and Price, 2003; Ferderer et al., 2024). At the community level, behavioral plasticity may enable individuals to react to changing resources and predation (Wong and Candolin, 2015; McMeans et al., 2016), while the selection of robust phenotypes over generations allows for genetic adaptation (Sunday et al., 2014). The basic survivability demonstrated in our study is a prerequisite for these compensatory mechanisms that could improve population resistance and recovery.
Over geological timescales, natural rock weathering and the resulting alkalization of the ocean have been buffering earth's climate. An artificial acceleration of this process could be accomplished through different scenarios, each one causing a distinct perturbation of seawater (Renforth and Henderson, 2017; Hartmann et al., 2023). We focused on changes in carbonate chemistry before CO2 equilibration of the alkalized water. Our inability to detect severe impacts here may classify the alternative, pre-CO2-equilibrated application as especially safe, given its stable CO2 partial pressure and smaller increases in pH and calcium carbonate saturation. With silicate addition, we tested a secondary driver of OAE that is hypothesized to cause major ecosystem shifts (Bach et al., 2019). There are several further drivers, however, such as trace metal toxicity/enrichment (Morel and Price, 2003) or suspended mineral particles (Affandi and Ishak, 2019), that are likely to play a role as well. A large-scale intervention such as OAE will inevitably bring about some level of environmental change, not just via the manipulation itself but also via supporting activities on land and at sea. OAE has to be seen in relation to other carbon dioxide removal technologies and the environmental, economic and social harm caused by unrestrained climate change. Our study on temperate coastal species helps to develop such a risk–benefit analysis towards the preservation of fish in marine ecosystems and the livelihoods they support.
The raw data supporting the conclusions of this article are available at https://doi.org/10.1594/PANGAEA.967059 (Goldenberg et al., 2024b).
The supplement related to this article is available online at: https://doi.org/10.5194/bg-21-4521-2024-supplement.
SUG, UR, MM, MS, GB and AF conceptualized the study; all authors conducted the experiments; SUG analyzed the data; SUG wrote the manuscript with particular input from MM and DB; and all authors revised the manuscript.
The contact author has declared that none of the authors has any competing interests.
Fish research was approved by the Norwegian Animal Research Authority (NARA) via the University of Bergen (laboratory, FOTS ref. 29008) and University of Agder (mesocosm, FOTS 28931).
Publisher’s note: Copernicus Publications remains neutral with regard to jurisdictional claims made in the text, published maps, institutional affiliations, or any other geographical representation in this paper. While Copernicus Publications makes every effort to include appropriate place names, the final responsibility lies with the authors.
This article is part of the special issue “Environmental impacts of ocean alkalinity enhancement”. It is not associated with a conference.
We thank the Espegrend Marine Biological Station and the University of Bergen for providing facilities and technical support. Further, we thank the whole KOSMOS team, including Andrea Ludwig for logistics, Jana Meyer for fish sediment sampling, Kai Schulz and Julieta Schneider for their expertise in carbonate chemistry, Leila Kittu and Anna Groen for chlorophyll filtrations, and Juliane Tammen for nutrient analyses.
This study was funded by the European Union's Horizon 2020 research and innovation program via the projects OceanNETS (869357) and AQUACOSM-plus (871081). Additional funding was provided through the German Research Foundation (THRESHOLDS, MO 2873/3-1), the Center for Coastal Research at the University of Agder, Villum Fonden (25512) and the Fundación CajaCanarias–Fundación Bancaria La Caixa via the project IMDEEP (ref: 2022CLISA15). María Couret was supported by a postgraduate grant (TESIS2022010116) from the Agencia Canaria de Investigación, Innovación y Sociedad de la Información (ACIISI).
This paper was edited by Lydia Kapsenberg and reviewed by two anonymous referees.
Affandi, F. A. and Ishak, M. Y.: Impacts of suspended sediment and metal pollution from mining activities on riverine fish population – a review, Environ. Sci. Pollut. Res., 26, 16939–16951, https://doi.org/10.1007/s11356-019-05137-7, 2019.
Bach, L. T., Gill, S. J., Rickaby, R. E. M., Gore, S., and Renforth, P.: CO2 Removal With Enhanced Weathering and Ocean Alkalinity Enhancement: Potential Risks and Co-benefits for Marine Pelagic Ecosystems, Front. Clim., 1, 7, https://doi.org/10.3389/fclim.2019.00007, 2019.
Blewett, T. A., Binning, S. A., Weinrauch, A. M., Ivy, C. M., Rossi, G. S., Borowiec, B. G., Lau, G. Y., Overduin, S. L., Aragao, I., and Norin, T.: Physiological and behavioural strategies of aquatic animals living in fluctuating environments, J. Exp. Biol., 225, jeb242503, https://doi.org/10.1242/jeb.242503, 2022.
Brownell, C. L.: Water quality requirements for first-feeding in marine fish larvae, II. pH, oxygen, and carbon dioxide, J. Exp. Mar. Biol. Ecol., 44, 285–298, https://doi.org/10.1016/0022-0981(80)90159-8, 1980.
Carstensen, J. and Duarte, C. M.: Drivers of pH variability in coastal ecosystems, Environ. Sci. Technol., 53, 4020–4029, https://doi.org/10.1021/acs.est.8b03655, 2019.
Cattano, C., Claudet, J., Domenici, P., and Milazzo, M.: Living in a high CO2 world: a global meta-analysis shows multiple trait-mediated fish responses to ocean acidification, Ecol. Monogr., 88, 320–335, https://doi.org/10.1002/ecm.1297, 2018.
Dahlke, F. T., Wohlrab, S., Butzin, M., and Pörtner, H. O.: Thermal bottlenecks in the life cycle define climate vulnerability of fish, Science, 369, 65–70, https://doi.org/10.1126/science.aaz3658, 2020.
Davidson, K., Roberts, E. C., and Gilpin, L. C.: The relationship between carbon and biovolume in marine microbial mesocosms under different nutrient regimes, Eur. J. Phycol., 37, 501–507, https://doi.org/10.1017/s096702620200389x, 2002.
Dickson, A. G., Sabine, C. L., and Christian, J. R.: Guide to best practices for ocean CO2 measurements, North Pacific Marine Science Organization, Sidney, BC, Canada, https://doi.org/10.25607/OBP-1342, 2007.
Doney, S. C., Busch, D. S., Cooley, S. R., and Kroeker, K. J.: The Impacts of Ocean Acidification on Marine Ecosystems and Reliant Human Communities, in: Annual Review of Environment and Resources, edited by: Gadgil, A. and Tomich, T. P., Annu. Rev. Environ. Res., 45, 83–112, https://doi.org/10.1146/annurev-environ-012320-083019, 2020.
Esbaugh, A. J.: Physiological implications of ocean acidification for marine fish: emerging patterns and new insights, J. Comp. Physiol. B, 188, 1–13, https://doi.org/10.1007/s00360-017-1105-6, 2018.
FAO: The State of World Fisheries and Aquaculture 2022, FAO, Rome, https://doi.org/10.4060/cc0461en, 2022.
Ferderer, A., Schulz, K. G., Riebesell, U., Baker, K. G., Chase, Z., and Bach, L. T.: Investigating the effect of silicate- and calcium-based ocean alkalinity enhancement on diatom silicification, Biogeosciences, 21, 2777–2794, https://doi.org/10.5194/bg-21-2777-2024, 2024.
Folkvord, A., Vollset, K. W., and Catalán, I. A.: Differences in growth and survival between cod Gadus morhua and herring Clupea harengus early stages co-reared at variable prey concentrations, J. Fish Biol., 87, 1176–1190, https://doi.org/10.1111/jfb.12783, 2015.
Folkvord, A., Lakso, E., Laupsa, M., Meier, S., Musialak, L. A., and Sundby, S.: Swimbladder filling in herring larvae: effects of food oil on the water surface, Mar. Biol. Res., 16, 446–457, https://doi.org/10.1080/17451000.2020.1837882, 2020.
Franke, A. and Clemmesen, C.: Effect of ocean acidification on early life stages of Atlantic herring (Clupea harengus L.), Biogeosciences, 8, 3697–3707, https://doi.org/10.5194/bg-8-3697-2011, 2011.
Frommel, A. Y., Maneja, R., Lowe, D., Pascoe, C. K., Geffen, A. J., Folkvord, A., Piatkowski, U., and Clemmesen, C.: Organ damage in Atlantic herring larvae as a result of ocean acidification, Ecol. Appl., 24, 1131–1143, https://doi.org/10.1890/13-0297.1, 2014.
Frommel, A. Y., Maneja, R., Lowe, D., Malzahn, A. M., Geffen, A. J., Folkvord, A., Piatkowski, U., Reusch, T. B., and Clemmesen, C.: Severe tissue damage in Atlantic cod larvae under increasing ocean acidification, Nat. Clim. Change, 2, 42–46, 2012.
Gattuso, J.-P., Williamson, P., Duarte, C. M., and Magnan, A. K.: The potential for ocean-based climate action: negative emissions technologies and beyond, Front. Clim., 2, 575716, https://doi.org/10.3389/fclim.2020.575716, 2021.
Gilpin, L. C., Davidson, K., and Roberts, E. C.: The influence of changes in nitrogen: silicon ratios on diatom growth dynamics, J. Sea Res., 51, 21–35, https://doi.org/10.1016/j.seares.2003.05.005, 2004.
Goldenberg, S. U., Nagelkerken, I., Ferreira, C. M., Ullah, H., and Connell, S. D.: Boosted food web productivity through ocean acidification collapses under warming, Glob. Change Biol., 23, 4177–4184, https://doi.org/10.1111/gcb.13699, 2017.
Goldenberg, S. U., Nagelkerken, I., Marangon, E., Bonnet, A., Ferreira, C. M., and Connell, S. D.: Ecological complexity buffers the impacts of future climate on marine consumers, Nat. Clim. Change, 8, 229–233, https://doi.org/10.1038/s41558-018-0086-0, 2018.
Goldenberg, S. U., Spisla, C., Sánchez, N., Taucher, J., Spilling, K., Sswat, M., Fiesinger, A., Fernández-Méndez, M., Krock, B., Hauss, H., Haussmann, J., and Riebesell, U.: Diatom-mediated food web functioning under ocean artificial upwelling, Sci. Rep., 14, 3955, https://doi.org/10.1038/s41598-024-54345-w, 2024a.
Goldenberg, S. U., Sswat, M., and Brüggemann, D.: KOSMOS mesocosm experiment Bergen 2022 on ocean alkalinity enhancement: fish abundance, biomass and sedimentation, PANGAEA [data set], https://doi.org/10.1594/PANGAEA.967059, 2024b.
Hansen, B., Bjornsen, P. K., and Hansen, P. J.: The size ratio between planktonic predators and their prey, Limnol. Oceanogr., 39, 395–403, https://doi.org/10.4319/lo.1994.39.2.0395, 1994.
Hansen, H. P. and Koroleff, F.: Determination of nutrients, Methods of seawater analysis, Wiley-VCH, Weinheim, Germany, 159–228, https://doi.org/10.1002/9783527613984.ch10, 1999.
Hansen, P. J.: Effect of high pH on the growth and survival of marine phytoplankton: implications for species succession, Aquat. Microb. Ecol., 28, 279–288, https://doi.org/10.3354/ame028279, 2002.
Hartmann, J., Suitner, N., Lim, C., Schneider, J., Marín-Samper, L., Arístegui, J., Renforth, P., Taucher, J., and Riebesell, U.: Stability of alkalinity in ocean alkalinity enhancement (OAE) approaches – consequences for durability of CO2 storage, Biogeosciences, 20, 781–802, https://doi.org/10.5194/bg-20-781-2023, 2023.
He, J. and Tyka, M. D.: Limits and CO2 equilibration of near-coast alkalinity enhancement, Biogeosciences, 20, 27–43, https://doi.org/10.5194/bg-20-27-2023, 2023.
Hofmann, G. E., Smith, J. E., Johnson, K. S., Send, U., Levin, L. A., Micheli, F., Paytan, A., Price, N. N., Peterson, B., Takeshita, Y., Matson, P. G., Crook, E. D., Kroeker, K. J., Gambi, M. C., Rivest, E. B., Frieder, C. A., Yu, P. C., and Martz, T. R.: High-frequency dynamics of ocean pH: a multi-ecosystem comparison, PLoS One, 6, e28983, https://doi.org/10.1371/journal.pone.0028983, 2011.
Houde, E. D.: Emerging from Hjort's shadow, J. Northw. Atlant. Fish. Sci., 41, 53–70, https://doi.org/10.2960/J.v41.m634, 2008.
Ianora, A. and Miralto, A.: Toxigenic effects of diatoms on grazers, phytoplankton and other microbes: a review, Ecotoxicology, 19, 493–511, https://doi.org/10.1007/s10646-009-0434-y, 2010.
Lackner, K. S.: Carbonate chemistry for sequestering fossil carbon, Annu. Rev. Energ. Env., 27, 193–232, https://doi.org/10.1146/annurev.energy.27.122001.083433, 2002.
Lüdecke, D., Ben-Shachar, M. S., Patil, I., Waggoner, P., and Makowski, D.: performance: an R package for assessment, comparison and testing of statistical models, Journal of Open Source Software, 6, 3139, https://doi.org/10.21105/joss.03139, 2021.
Lueker, T. J., Dickson, A. G. and Keeling, C. D.: Ocean pCO2 calculated from dissolved inorganic carbon, alkalinity, and equations for K1 and K2: validation based on laboratory measurements of CO2 in gas and seawater at equilibrium, Mar. Chem., 70, 105–119, https://doi.org/10.1016/S0304-4203(00)00022-0, 2000.
Maneja, R. H., Frommel, A. Y., Browman, H. I., Geffen, A. J., Folkvord, A., Piatkowski, U., Durif, C. M. F., Bjelland, R., Skiftesvik, A. B., and Clemmesen, C.: The swimming kinematics and foraging behavior of larval Atlantic herring (Clupea harengus L.) are unaffected by elevated pCO2, J. Exp. Mar. Biol. Ecol., 466, 42–48, https://doi.org/10.1016/j.jembe.2015.02.008, 2015.
McMeans, B. C., McCann, K. S., Tunney, T. D., Fisk, A. T., Muir, A. M., Lester, N., Shuter, B., and Rooney, N.: The adaptive capacity of lake food webs: from individuals to ecosystems, Ecol. Monogr., 86, 4–19, https://doi.org/10.1890/15-0288.1, 2016.
Melzner, F., Gutowska, M. A., Langenbuch, M., Dupont, S., Lucassen, M., Thorndyke, M. C., Bleich, M., and Portner, H. O.: Physiological basis for high CO2 tolerance in marine ectothermic animals: pre-adaptation through lifestyle and ontogeny?, Biogeosciences, 6, 2313–2331, https://doi.org/10.5194/bg-6-2313-2009, 2009.
Morel, F. M. M. and Price, N. M.: The biogeochemical cycles of trace metals in the oceans, Science, 300, 944–947, https://doi.org/10.1126/science.1083545, 2003.
Nagelkerken, I. and Connell, S. D.: Global alteration of ocean ecosystem functioning due to increasing human CO2 emissions, P. Natl. Acad. Sci. USA, 112, 13272–13277, https://doi.org/10.1073/pnas.1510856112, 2015.
Nawaz, S., Lezaun, J., Valenzuela, J. M., and Renforth, P.: Broaden research on ocean alkalinity enhancement to better characterize social impacts, Environ. Sci. Technol., 57, 8863–8869, https://doi.org/10.1021/acs.est.2c09595, 2023.
Nejstgaard, J. C., Hygum, B. H., Naustvoll, L. J., and Bamstedt, U.: Zooplankton growth, diet and reproductive success compared in simultaneous diatom- and flagellate-microzooplankton-dominated plankton blooms, Mar. Ecol.-Prog. Ser., 221, 77–91, https://doi.org/10.3354/meps221077, 2001.
Ockendon, N., Baker, D. J., Carr, J. A., White, E. C., Almond, R. E. A., Amano, T., Bertram, E., Bradbury, R. B., Bradley, C., Butchart, S. H. M., Doswald, N., Foden, W., Gill, D. J. C., Green, R. E., Sutherland, W. J., Tanner, E. V. J., and Pearce-Higgins, J. W.: Mechanisms underpinning climatic impacts on natural populations: altered species interactions are more important than direct effects, Glob. Change Biol., 20, 2221–2229, https://doi.org/10.1111/gcb.12559, 2014.
Oksanen, J., Simpson, G., Blanchet, F., Kindt, R., Legendre, P., Minchin, P., O'Hara, R., Solymos, P., Stevens, M., Szoecs, E., Wagner, H., Barbour, M., Bedward, M., Bolker, B., Borcard, D., Carvalho, G., Chirico, M., De Caceres, M., Durand, S., Evangelista, H., FitzJohn, R., Friendly, M., Furneaux, B., Hannigan, G., Hill, M., Lahti, L., McGlinn, D., Ouellette, M., Ribeiro Cunha, E., Smith, T., Stier, A., Ter Braak, C., and Weedon, J.: vegan: community ecology package, R package version 2.6-4, https://vegandevs.github.io/vegan/ (last access: 10 October 2024), 2022.
Omar, A. M., Skjelvan, I., Erga, S. R., and Olsen, A.: Aragonite saturation states and pH in western Norwegian fjords: seasonal cycles and controlling factors, 2005–2009, Ocean Sci., 12, 937–951, https://doi.org/10.5194/os-12-937-2016, 2016.
Pancic, M., Torres, R. R., Almeda, R., and Kiorboe, T.: Silicified cell walls as a defensive trait in diatoms, Proc. R. Soc. B, 286, 20190184, https://doi.org/10.1098/rspb.2019.0184, 2019.
Parra, G. and Yufera, M.: Tolerance response to water pH in larvae of two marine fish species, gilthead seabream, Sparus aurata (L.) and Senegal sole, Solea senegalensis (Kaup), during development, Aquac. Res., 33, 747–752, https://doi.org/10.1046/j.1365-2109.2002.00713.x, 2002.
Paulino, A. I., Larsen, A., Bratbak, G., Evens, D., Erga, S. R., Bye-Ingebrigtsen, E., and Egge, J. K.: Seasonal and annual variability in the phytoplankton community of the Raunefjord, west coast of Norway from 2001–2006, Mar. Biol. Res., 14, 421–435, 2018.
Perry, S. F. and Gilmour, K. M.: Acid-base balance and CO2 excretion in fish: unanswered questions and emerging models, Resp. Physiol. Neurobi., 154, 199–215, https://doi.org/10.1016/j.resp.2006.04.010, 2006.
Pierrot, D., Epitalon, J.-M., Orr, J. C., Lewis, E., and Wallace, D. W. R.: MS Excel program developed for CO2 system calculations – version 3.0, GitHub repository, https://github.com/dpierrot/co2sys_xl (last access: 10 December 2023), 2021.
Pörtner, H. O.: Ecosystem effects of ocean acidification in times of ocean warming: a physiologist's view, Mar. Ecol.-Prog. Ser., 373, 203–217, https://doi.org/10.3354/meps07768, 2008.
R Core Team: R: A language and environment for statistical computing, R Foundation for Statistical Computing, Vienna, Austria, URL https://www.R-project.org/ (last access: 10 October 2024), 2021.
Renforth, P. and Henderson, G.: Assessing ocean alkalinity for carbon sequestration, Rev. Geophys., 55, 636–674, https://doi.org/10.1002/2016rg000533, 2017.
Riebesell, U., Czerny, J., von Brockel, K., Boxhammer, T., Budenbender, J., Deckelnick, M., Fischer, M., Hoffmann, D., Krug, S. A., Lentz, U., Ludwig, A., Muche, R., and Schulz, K. G.: Technical Note: A mobile sea-going mesocosm system – new opportunities for ocean change research, Biogeosciences, 10, 1835–1847, https://doi.org/10.5194/bg-10-1835-2013, 2013.
Riebesell, U., Basso, D., Geilert, S., Dale, A. W., and Kreuzburg, M.: Mesocosm experiments in ocean alkalinity enhancement research, in: Guide to Best Practices in Ocean Alkalinity Enhancement Research, edited by: Oschlies, A., Stevenson, A., Bach, L. T., Fennel, K., Rickaby, R. E. M., Satterfield, T., Webb, R., and Gattuso, J.-P., Copernicus Publications, State Planet, 2-oae2023, 6, https://doi.org/10.5194/sp-2-oae2023-6-2023, 2023.
Rogelj, J., Popp, A., Calvin, K. V., Luderer, G., Emmerling, J., Gernaat, D., Fujimori, S., Strefler, J., Hasegawa, T., Marangoni, G., Krey, V., Kriegler, E., Riahi, K., van Vuuren, D. P., Doelman, J., Drouet, L., Edmonds, J., Fricko, O., Harmsen, M., Havlik, P., Humpenoder, F., Stehfest, E., and Tavoni, M.: Scenarios towards limiting global mean temperature increase below 1.5 °C, Nat. Clim. Change, 8, 325–332, https://doi.org/10.1038/s41558-018-0091-3, 2018.
Sarthou, G., Timmermans, K. R., Blain, S., and Treguer, P.: Growth physiology and fate of diatoms in the ocean: a review, J. Sea Res., 53, 25–42, https://doi.org/10.1016/j.seares.2004.01.007, 2005.
Schulz, K. G., Bach, L. T., and Dickson, A. G.: Seawater carbonate chemistry considerations for ocean alkalinity enhancement research: theory, measurements, and calculations, in: Guide to Best Practices in Ocean Alkalinity Enhancement Research, edited by: Oschlies, A., Stevenson, A., Bach, L. T., Fennel, K., Rickaby, R. E. M., Satterfield, T., Webb, R., and Gattuso, J.-P., Copernicus Publications, State Planet, 2-oae2023, 2, https://doi.org/10.5194/sp-2-oae2023-2-2023, 2023.
Schulz, K. G., Bach, L. T., Bellerby, R. G. J., Bermúdez, R., Büdenbender, J., Boxhammer, T., Czerny, J., Engel, A., Ludwig, A., Meyerhöfer, M., Larsen, A., Paul, A. J., Sswat, M., and Riebesell, U.: Phytoplankton blooms at increasing levels of atmospheric carbon dioxide: experimental evidence for negative effects on prymnesiophytes and positive on small picoeukaryotes, Front. Mar. Sci., 4, 64, https://doi.org/10.3389/fmars.2017.00064, 2017.
Sommer, U., Stibor, H., Katechakis, A., Sommer, F., and Hansen, T.: Pelagic food web configurations at different levels of nutrient richness and their implications for the ratio fish production: primary production, Hydrobiologia, 484, 11–20, https://doi.org/10.1023/a:1021340601986, 2002.
Spisla, C., Taucher, J., Sswat, M., Wunderow, H., Kohnert, P., Clemmesen, C., and Riebesell, U.: Ocean acidification alters the predator – prey relationship between hydrozoa and fish larvae, Front. Mar. Sci., 9, 831488, https://doi.org/10.3389/fmars.2022.831488, 2022.
Sswat, M., Stiasny, M. H., Jutfelt, F., Riebesell, U., and Clemmesen, C.: Growth performance and survival of larval Atlantic herring, under the combined effects of elevated temperatures and CO2, PLoS One, 13, e0191947, https://doi.org/10.1371/journal.pone.0191947, 2018a.
Sswat, M., Stiasny, M. H., Taucher, J., Alguero-Muniz, M., Bach, L. T., Jutfelt, F., Riebesell, U., and Clemmesen, C.: Food web changes under ocean acidification promote herring larvae survival, Nat. Ecol. Evol., 2, 836–840, https://doi.org/10.1038/s41559-018-0514-6, 2018b.
Stiasny, M. H., Mittermayer, F. H., Sswat, M., Voss, R., Jutfelt, F., Chierici, M., Puvanendran, V., Mortensen, A., Reusch, T. B., and Clemmesen, C.: Ocean acidification effects on Atlantic cod larval survival and recruitment to the fished population, PLoS One, 11, e0155448, https://doi.org/10.1371/journal.pone.0155448, 2016.
Sunday, J. M., Calosi, P., Dupont, S., Munday, P. L., Stillman, J. H., and Reusch, T. B. H.: Evolution in an acidifying ocean, Trend. Ecol. Evol., 29, 117–125, https://doi.org/10.1016/j.tree.2013.11.001, 2014.
Toresen, R., Skjoldal, H. R., Vikelao, F., and Martinussen, M. B.: Sudden change in long-term ocean climate fluctuations corresponds with ecosystem alterations and reduced recruitment in Norwegian spring-spawning herring (Clupea harengus, Clupeidae), Fish. Fish., 20, 686–696, https://doi.org/10.1111/faf.12369, 2019.
Tresguerres, M., Clifford, A. M., Harter, T. S., Roa, J. N., Thies, A. B., Yee, D. P., and Brauner, C. J.: Evolutionary links between intra- and extracellular acid-base regulation in fish and other aquatic animals, J. Exp. Zool. Part A, 333, 449–465, https://doi.org/10.1002/jez.2367, 2020.
Turner, J. T.: The feeding ecology of some zooplankters that are important prey items of larval fish, NOAA Technical Report NMFS 7, https://repository.library.noaa.gov/view/noaa/5581 (last access: 10 October 2024), 1984.
Vinagre, C., Leal, I., Mendonça, V., Madeira, D., Narciso, L., Diniz, M. S., and Flores, A. A. V.: Vulnerability to climate warming and acclimation capacity of tropical and temperate coastal organisms, Ecol. Indic., 62, 317–327, https://doi.org/10.1016/j.ecolind.2015.11.010, 2016.
Wong, B. B. M. and Candolin, U.: Behavioral responses to changing environments, Behav. Ecol., 26, 665–673, https://doi.org/10.1093/beheco/aru183, 2015.