the Creative Commons Attribution 4.0 License.
the Creative Commons Attribution 4.0 License.
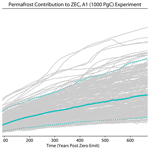
Estimated effect of the permafrost carbon feedback on the zero emissions commitment to climate change
Zero Emissions Commitment (ZEC), the expected change in global temperature following the cessation of anthropogenic greenhouse gas emissions, has recently been assessed by the Zero Emissions Commitment Model Intercomparison Project (ZECMIP). ZECMIP concluded that the component of ZEC from CO2 emissions will likely be close to zero in the decades following the cessation of emissions. However, of the 18 Earth system models that participated in ZECMIP only 2 included a representation of the permafrost carbon feedback to climate change. To better assess the potential impact of permafrost carbon decay on ZEC, a series of perturbed parameter experiments are here conducted with an Earth system model of intermediate complexity. The experiment suggests that the permafrost carbon cycle feedback will directly add 0.06 [0.02 to 0.14] ∘C to the benchmark the ZEC value assesses 50 years after 1000 Pg C of CO2 has been emitted to the atmosphere. An additional 0.04 [0 to 0.06] ∘C is likely to been added relative to the benchmark ZEC value from the thaw-lag effect unaccounted for in the ZECMIP experiment design. Overall I assess that the permafrost carbon feedback is unlikely to change the assessment that ZEC is close to zero on decadal timescales; however, the feedback is expected to become more important over the coming centuries.
- Article
(4092 KB) - Full-text XML
- BibTeX
- EndNote
The Zero Emissions Commitment (ZEC) is the change in global temperature expected to occur following the cessation of anthropogenic emissions of greenhouse gases and aerosols (Hare and Meinshausen, 2006; MacDougall et al., 2020). ZEC is one of five metrics needed to compute the “remaining carbon budget”, which in turn quantifies the total emissions compatible with meeting a given temperature change guardrail (e.g. Rogelj et al., 2018; Matthews et al., 2021), such as those set out in the Paris Agreement (United Nations, 2015). ZEC was recently the focus of a model intercomparison project organized through the Coupled Model Intercomparison Project phase 6 (CMIP6) (Jones et al., 2019). The project, formally called the Zero Emissions Commitment Model Intercomparison Project (ZECMIP) (Jones et al., 2019), gathered simulations from 18 Earth system models of full and intermediate complexity (MacDougall et al., 2020) and assessed the CO2 component of ZEC. For the tier-one idealized experiment where 1000 Pg C of CO2 was emitted to the atmosphere before cessation of emissions, ZEC ranged from −0.36 to 0.29 ∘C with a model ensemble mean of −0.07 ∘C 50 years after emissions ceased. However, only 2 of the 18 models that participated in ZECMIP had a representation of the permafrost carbon feedback to climate change, a feedback process that is expected to release CO2 and CH4 into the atmosphere for centuries after emissions cease (Schuur et al., 2015; McGuire et al., 2018). Thus the effect of the permafrost carbon feedback on ZEC has yet to be well quantified.
The soils of the Northern Hemisphere permafrost region are estimated to contain between 1100 and 1500 Pg C of organic matter (Hugelius et al., 2014), about half of which is held in the perennially frozen zone of these soils (Hugelius et al., 2014). As climate warms and permafrost thaws, organic matter in permafrost-affected soils is exposed to increased periods of time where local temperature is above freezing, and hence to enhanced rate of decay releasing CH4 and CO2 to the atmosphere (e.g. Schuur et al., 2015). A recent informal (non-CMIP) model intercomparison exercise quantifying the permafrost carbon feedback estimated a release of carbon of between 74 and 652 Pg C by year 2300 under the high-end Representative Concentration Pathway 8.5 scenario, with substantially lower release or even gain of soil carbon under mitigation scenarios (McGuire et al., 2018). Thus the permafrost carbon feedback to climate change has the potential to affect the value of ZEC in a fashion that was poorly quantified by ZECMIP.
Uncertainty in projections from Earth system models can be classified into three components: (1) structural uncertainty, (2) parameter uncertainty, and (3) scenario uncertainty (e.g. MacDougall and Knutti, 2016). Structural uncertainty is created from the discrepancy between the system the model is intended to represent and the system the model actually describes (Smith, 2007; Eyring et al., 2016). Examining different models of the same system with standardized forcings through model intercomparison projects such as ZECMIP is the principle way of quantifying structural uncertainty in climate sciences (Eyring et al., 2016). Parameter uncertainty is uncertainty about the value that model parameter should take on (Smith, 2007). As model parameters are sometimes quantities measurable in the natural world, parameter uncertainty is some cases equivalent to measurement uncertainty. In other cases parameters represent an amalgam of natural processes; in such cases defining parameter uncertainty becomes more ambiguous (Smith, 2007). Parameter uncertainty can be quantified with perturbed parameter experiments, wherein ensembles of model variants with parameter values selected from defined probability distribution functions are run under the same experiment conditions (e.g. Forest et al., 2002). Several such experiments have been conducted to assess uncertainty in the permafrost carbon feedback (Schneider von Deimling et al., 2015; MacDougall and Knutti, 2016; Gasser et al., 2018). Scenario uncertainty is created by uncertainty about what humans will do in the future and is well explored by the coordinated scenario framework of CMIP (Eyring et al., 2016; O'Neill et al., 2017).
For the permafrost carbon feedback, uncertainty ranges derived from structural uncertainty and parameter uncertainty assessments have proven similar. The model intercomparison exercise of McGuire et al. (2018) found a range of 74 to 652 Pg C with a mean of 341 Pg C released from permafrost soils by year 2300 under Representative Concentration Pathway 8.5, compared to 159 to 587 Pg C with a mean of 376 Pg C for the perturbed parameter experiment of MacDougall and Knutti (2016), forced under the same scenario.
In addition to 16 out of the 18 ZECMIP models having no permafrost carbon module, the experimental design of ZECMIP is ill designed to quantify the permafrost carbon feedback. The top-tier idealized ZECMIP experiment branches from the idealized 1pctCO2 experiment where atmospheric CO2 concentration rises at 1 % a year compounded leading to a quadrupling of CO2 concentration in 140 years (Jones et al., 2019; Eyring et al., 2016). Following this protocol CO2 and hence global temperatures rise much faster than in the historical trajectory, and since it takes time for permafrost soil to thaw and organic matter within these soils to decay, the experimental protocol will tend to underestimate release of carbon from permafrost soils (MacDougall, 2019).
Here I will use a perturbed parameter ensemble approach to estimate the contribution to CO2-only ZEC from the release of carbon from permafrost soils, following the ZECMIP protocol. I will also conduct an experiment following a more realistic CO2 emission trajectory in order to quantity the thaw-lag effect from the high emission rates of the ZECMIP protocol.
2.1 Model description
The University of Victoria Earth System Climate Model (UVic ESCM) is a climate model of intermediate complexity founded around a three-dimensional ocean general circulation model coupled to a simplified moisture and energy balance atmosphere (Weaver et al., 2001). The version of the model used here (version 2.9pf) has representation of the oceanic and terrestrial carbon cycles (Schmittner et al., 2008; Meissner et al., 2003). The oceanic carbon cycle has representations of ocean carbonate chemistry (Weaver et al., 2001), phytoplankton–zooplankton–detritus ocean biology scheme (Schmittner et al., 2008), and interaction between ocean sediments and alkalinity (Archer, 1996). The terrestrial component is composed of the Top-down Representation of Interactive Foliage and Flora Including Dynamics (Triffid) dynamic vegetation model (Cox et al., 2001; Meissner et al., 2003), a multi-layer representation soil respiration (MacDougall et al., 2012), and a permafrost carbon module (MacDougall and Knutti, 2016).
The terrestrial subsurface of the model is composed of 14 layers, reaching a total depth of 250 m (Avis et al., 2011). The top eight layers (10 m) are active in the hydraulic cycle and deeper layers are impermeable bedrock (Avis et al., 2011). The freeze–thaw physics of the soil accounts for the effect of soil valence forces on freezing point, and the frozen and unfrozen fraction of the soil water is calculated using equations the minimize Gibbs free energy (Avis, 2012). The top six layers of the model (3.35 m) are active in the carbon cycle. Carbon is assigned to soil layers from Triffid based on the root density in each soil layer, with remaining dead plant matter added to the top soil layer (MacDougall et al., 2012). Root density varies by plant function type and the temperature of the soil layer (roots do not grow in frozen soil) (MacDougall et al., 2012). In model grid cells where permafrost exists (where soil layers have been below 0 ∘C for two or more consecutive years) a diffusion-based cryoturbation scheme is used to redistribute soil carbon in the soil column. The scheme was originally developed by Koven et al. (2009) and modified for implementation in the UVic ESCM in MacDougall and Knutti (2016). The scheme allows for a permafrost carbon pool to be generated alongside regular soil carbon in permafrost soils. The modifications made to the scheme by MacDougall and Knutti (2016) allow the permafrost carbon pool to come into equilibrium during the 5000-year model spin-up. The version of the UVic ESCM used here does not have a methane production module. Thus emissions of carbon from soils to the atmosphere happen only as CO2.
In the version of the UVic ESCM used here (MacDougall and Knutti, 2016) permafrost carbon is a separate carbon pool. Permafrost carbon is created when carbon is advected across the permafrost table by the cryoturbation scheme and can only be destroyed by being respired into CO2. The pool is characterized by a decay rate constant (κp), a fraction of the pool that is available for decay (available fraction, Af), and a passive pool transformation rate (κtf), which is the rate at which the passive permafrost carbon becomes part of the available fraction. The available fraction is essentially the combined size of the fast and slow carbon pools as conceptualized in incubation experiments (Schädel et al., 2014; MacDougall and Knutti, 2016). This scheme accounts for the large fraction of permafrost carbon that is very resistant to decay (Schädel et al., 2014) while still allowing the pool to decay over millennial time periods (MacDougall and Knutti, 2016). A fourth parameter, the saturation factor (S) from the cryoturbation scheme allows the size of the permafrost carbon pool to be tuned (MacDougall and Knutti, 2016). The saturation factor is indexed to the mineral porosity of soils (which vary by grid cell and soil layer) and accounts for the diminishing concentration of soil carbon at depth in permafrost regions (Hugelius et al., 2014).
The model experiments here use the permafrost carbon variant of the UVic ESCM 2.9 detailed in MacDougall and Knutti (2016). A newer version of the UVic ESCM (version 2.10) is now available (Mengis et al., 2020). I use the older version of the model to allow for the use of legacy code and legacy model spin-ups from MacDougall and Knutti (2016). Note that the terrestrial component of UVic ESCM 2.10 was taken from the version developed for MacDougall and Knutti (2016), and thus the terrestrial components of the model versions are virtually identical (Mengis et al., 2020).
By changing the flow of outgoing longwave radiation to space as a function of global surface temperature anomaly, the climate sensitivity of the UVic ESCM can be altered (Zickfeld et al., 2009). Similarly by changing the meridional diffusivity of the atmosphere within the model, arctic amplification can also be altered (Fyke et al., 2014).
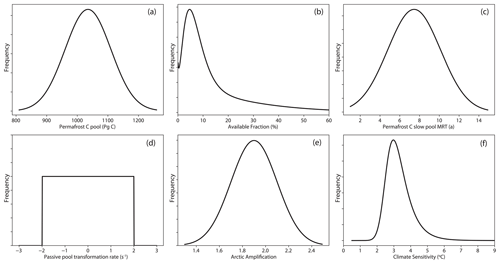
Figure 1Probability distribution functions of the six parameters perturbed in this study. MRT is mean residence time. Note that (d) has a logarithmic scale. All parameter PDFs except climate sensitivity are that same as in MacDougall and Knutti (2016).
2.2 Perturbed parameter experiments
To assess the uncertainty in the strength of the permafrost carbon cycle feedback to climate change, MacDougall and Knutti (2016) generated 250 variants of the UVic ESCM by perturbing six model parameters. Four of these parameters control the size and susceptibility to decay of the permafrost carbon pool, and two (climate sensitivity and arctic amplification) are physical climate parameters. The four permafrost carbon parameters are the following: (1) the permafrost carbon decay constant; (2) the available fraction; (3) the passive pool transformation rate; and (4) the permafrost carbon saturation factor – which controls the size of the permafrost carbon pool. The permafrost carbon decay constant controls how fast available permafrost carbon can decay given the temperature and moisture of the soil. The available fraction is the fraction of permafrost carbon that is allowed to decay, effectively the fraction of permafrost carbon that is unprotected or weakly protected from decay. The passive pool transformation rate is the rate at which highly protected permafrost carbon becomes weakly protected. The probability density functions (PDFs) for the permafrost carbon decay constant and the available fraction were taken from the meta-analysis of permafrost carbon incubation experiments conducted by Schädel et al. (2014). The passive pool transformation rate is constrained primarily by the non-existence of a remnant mid-latitude permafrost carbon pool from the last glacial maximum, yielding an estimated value of to s−1, with a best guess of s−1 (Trumbore, 2000; MacDougall and Knutti, 2016). The analysis of MacDougall and Knutti (2016) showed that the passive pool transformation rate has only a weak effect of the permafrost carbon feedback on decadal and centennial timescales. The estimated uncertainty in the size of the permafrost carbon pool was taken from Hugelius et al. (2014). For each model variant 5000-year model spin-ups were conducted with year 1850 of the Common Era (CE) radiative forcing to bring the permafrost carbon into equilibrium, representing an investment of 1.25 million model years of simulation time. We have re-used these model spin-ups for the present study.
MacDougall and Knutti (2016) also perturbed two physical model parameters: climate sensitivity and arctic amplification. These parameters do not affect the model spin-up as both affect deviations from the pre-industrial climate; thus they can be changed for the present study. To my knowledge there has been no major update in the uncertainty range of arctic amplification since 2016. However, Sherwood et al. (2020) have substantially constrained the uncertainty in equilibrium climate sensitivity to a 5th to 95th percentile range of 2.3 to 4.7 ∘C for a doubling of atmospheric CO2 concentration. Thus I have computed new climate sensitivity parameters for the 250 model variants. I use the same functional form for the climate sensitivity PDFs as previous papers (e.g. Olson et al., 2012; MacDougall and Knutti, 2016), a product of two normal-inverse Gaussian functions. To get new parameter values for the climate sensitivity PDF a Monte Carlo method was used to fit the function to the distribution outlined by Sherwood et al. (2020): a 5th to 95th percentile range of 2.3 to 4.7 ∘C, a 66 % range of 2.6 to 3.9 ∘C, and a median value of 3.0 ∘C. The new parameter values for the PDF are given in Appendix A.
Figure 1 shows the PDFs of each parameter perturbed in this study. Note that all but equilibrium climate sensitivity are identical to MacDougall and Knutti (2016).
2.3 Model experiments
To quantify the effect of the permafrost carbon feedback on ZEC, we have three key questions: (1) How much warming will the permafrost carbon feedback add to ZEC? (2) What is the magnitude of the thaw-lag permafrost effect from using the 1pctCO2 experiment to quantify ZEC? And (3) how sensitive is the permafrost carbon feedback contribution to ZEC to total CO2 emitted before cessation of emissions?
To answer the first question we would ideally compare simulations with and without permafrost carbon that are otherwise identical. In the UVic ESCM framework we can create a version without permafrost carbon by setting the cryoturbation diffusion parameter to zero during model spin-up. Without cryoturbation there will be no permafrost carbon pool, and the active layer carbon pool will also be reduced in size. However, the presence of carbon in soils subtly changes soil thermal and hydraulic properties in the UVic ESCM (Avis, 2012) such that the absence of a permafrost carbon pool could change the baseline climate conditions of the model. To test the magnitude of this effect simulations were conducted with the UVic ESCM with the cryoturbation diffusion parameter set to zero and all other model parameters held at their default settings. The model version was spun up for 5000 years. Both the version of the model with cryoturbation set to zero and the default version of the model were forced with the 1pctCO2 experiment (where CO2 concentration rises at 1 % per year compounded). Figure B1 shows that the difference between the two simulations is minimal with respect to global average temperature, with a 0.01 ∘C difference in baseline global temperature and a smaller difference by the time atmospheric CO2 concentration doubled in year 70 of the experiment. The difference in regular (non-permafrost) soil carbon is also small between the two simulation. The simulation without cryoturbation has 1837 Pg C of regular soil carbon in the pre-industrial state, and the simulation with cryoturbation has 1853 Pg C in the regular soil carbon pools in the pre-industrial state.
Thus to quantify the effect of permafrost carbon feedback on ZEC, two parallel sets of experiments were conducted. In one set of experiments model spin-ups from MacDougall and Knutti (2016) were used along with the 250 variants of the model to compute ZEC including permafrost. In a second set of experiments a single model spin-up with the cryoturbation diffusion parameter set to zero was used and 250 model variants were generated using just the climate sensitivity and arctic amplification parameters from the perturbed parameter sets. Thus each parallel variant pair will have the same climate sensitivity and arctic amplification parameters with only the existence of permafrost carbon different between the parallel variants. All model variants were forced with the esm-1pct-brch-1000PgC (A1) ZEC experiment described in Jones et al. (2019) where the 1000 Pg C of carbon is emitted following the 1pctCO2 experiment pathway, and emissions instantaneously go to zero once 1000 Pg C is reached. All non-CO2 forcings are held either at their year 1850 CE values or their long-term mean for volcanic and solar forcing. The simulations are forced with CO2 emissions diagnosed from the default version of the UVic ESCM 2.9pf such that all simulations are forced with the same CO2 emissions pathway. Thus most model variants will only approximately follow the 1pctCO2 CO2 trajectory, but all variants have the same point in time that emissions cease, greatly simplifying analysis of the results. The difference between parallel variants with and without permafrost carbon quantifies the effect of the permafrost carbon pool on ZEC.
To quantify the permafrost thaw-lag effect a set of experiments were conducted with the 250 model variants with permafrost carbon. The model variants were forced with a CO2 emissions trajectory that follows historical emissions until year 2019 (Friedlingstein et al., 2020) and afterwards follows the CO2 emissions trajectory of a Shared Socioeconomic Pathway (SSP) (O'Neill et al., 2017) until 1000 Pg C has been emitted (Fig. 2). Thereafter CO2 emissions are set to zero. All non-CO2 forcings are held either at their year 1850 CE values or their long-term mean for volcanic and solar forcing. Eight SSPs were used by CMIP6 to quantify scenario uncertainty (O'Neill et al., 2017). For the experiment conducted here I selected two SSP-based criteria: that the SSP reaches 1000 Pg C of CO2 emissions and that the 1000 Pg C cumulative CO2 emissions total is reached before emissions begin to approach zero. The second criterion is needed as the ZECMIP bell-shaped curve experiments showed that the Transient Climate Response to Cumulative CO2 Emissions (TCRE) and ZEC effects become mixed as emissions approach zero (MacDougall et al., 2020). Therefore a sudden cessation of emissions is needed to separate TCRE from ZEC. SSP4-6.0 is the lowest emission SSP that reaches 1000 Pg C whilst maintaining near-peak CO2 emissions. Thus using SSP4-6.0 maximizes the time need to reach 1000 Pg C and therefore is optimal for assessing the thaw-lag effect. Under SSP4-6.0 CO2 emissions 1000 Pg C is reached in year 2067 of the Common Era (CE), allowing permafrost the time to thaw and the organic matter within it to decay.
The effect of total CO2 emissions on the permafrost carbon contribution to ZEC is quantified by forcing each of the 250 parallel model variants with the esm-1pct-brch-2000PgC (A3) ZEC experiment from Jones et al. (2019), wherein 2000 Pg C of CO2 is emitted following the 1pctCO2 experiment pathway and emissions instantaneously go to zero once 2000 Pg C is reached. Again the permafrost carbon effect on ZEC is computed from the difference between parallel variants with and without permafrost carbon. Model experiments are summarized in Table 1.
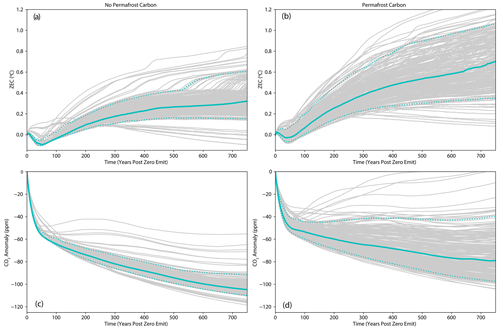
Figure 3(a, b) Zero Emission Commitment (ZEC) for model versions without (a) and with (b) a permafrost carbon pool forced by the A1 (1000 Pg C) experiment. ZEC is temperature change relative to the year emissions cease. (c, d) Change in atmospheric CO2 concentration relative to year emissions cease for model versions without (c) and with (d) a permafrost carbon pool, forced by the A1 (1000 Pg C) experiment. Grey lines are individual model variants, solid line is the median of the variants, and dashed lines are the 5th and 95th percentile. Sudden increase in warming rate seen in many warmer model variants is associated with the disappearance of perennial sea ice in the Weddell and Ross seas and concurrent changes in overturning circulation.
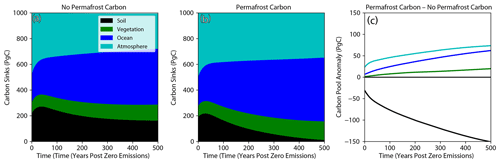
Figure 4(a, b) Size of carbon sinks following cessation of emissions in the A1 (1000 Pg C) experiments with and without permafrost carbon. (c) Difference in size of carbon pools between the simulation with and the simulation without permafrost carbon. The total of all carbon sinks remains 1000 Pg C after emissions cease.
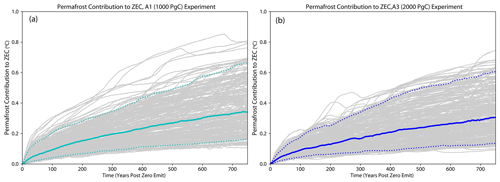
Figure 5Difference in ZEC between experiments with and without permafrost carbon. (a) Anomalies for the A1 (1000 Pg C) experiment. (b) Anomalies for the A2 (2000 Pg C) experiment. Grey lines are individual model variants, solid line is the median of the variants, and dashed lines are the 5th and 95th percentile.
Table 2Median anomalies in ZEC created by release of carbon from permafrost soils, and the magnitude of the respective carbon release. Values in square brackets are 5th to 95th percentile ranges from the perturbed parameter experiments.

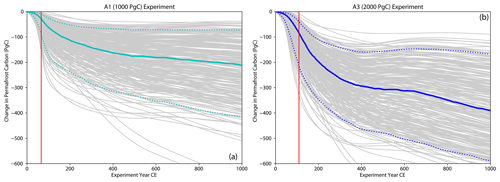
Figure 6Change in the soil carbon held in permafrost regions relative to pre-industrial size. (a) Change for the A1 (1000 Pg C) experiment. (b) Change for the A2 (2000 Pg C) experiment. Grey lines are individual model variants, solid line is the median of the variants, and dashed lines are the 5th and 95th percentile. Vertical red line marks the time emissions cease.
Figure 3 shows ZEC for the A1 (1000 Pg C) experiment for the model versions with and without permafrost carbon. The figure shows that over centennial timescales the model version with permafrost carbon has a higher ZEC. Consistent with a higher ZEC, the model version with permafrost carbon exhibits a slower decline in atmospheric CO2 concentration after emissions cease. Figure 5a displays the difference in ZEC between the simulations with and without permafrost carbon for the A1 (1000 Pg C) experiment. Fifty years after emissions cease the existence of a permafrost carbon pool has added 0.06 [0.02 to 0.14] ∘C to ZEC (median [5th to 95th percentile]), rising to an addition of 0.09 [0.04 to 0.21] ∘C 100 years after emissions cease, and 0.27 [0.12 to 0.49] ∘C 500 years after emissions cease (Table 2). The additional warming is being driven by release of carbon from permafrost soils, which totals 29 [10 to 90] Pg C by the time emissions cease, 73 [32 to 190] Pg C by 50 years after emissions cease, 100 [46 to 222] Pg C 100 years after emissions cease, and 178 [70 to 346] Pg C 500 years after emissions cease (Fig. 6a, Table 2). The global mean temperature anomaly at the time emissions cease is 1.51 [1.41 to 1.58] ∘C for the non-permafrost carbon experiment and 1.55 [1.47 to 1.67] ∘C for the permafrost carbon experiment. Thus, the carbon released from permafrost soils by the time emissions ceases causes 0.04 [0.01 to 0.12] ∘C of additional warming in the model versions with permafrost carbon. The value of ZEC is determined by a balance of the warming effect of diminishing ocean heat uptake and the cooling effect of declining atmospheric CO2 concentration (MacDougall et al., 2020); thus the initial cooling after emissions cease is likely caused by the initial rapid drop in atmospheric CO2 concentration (3c, and d).
Figure 4 shows mean difference in global carbon pool sized in the simulations with and without permafrost carbon, averaged across all of the model variants, for the A1 (1000 Pg C) experiment. The excess carbon released from permafrost soils is taken up by vegetation, the ocean, and the atmosphere. Most of the excess carbon resides in the atmosphere for centuries after emissions cease, with the ocean gradually becoming a more significant sink. Vegetation remains a relatively small sink throughout the experiments. Figure 4 also suggests that the source of the rapid fall in atmospheric CO2 in the decades after emissions cease is continued growth of the vegetation and soil carbon sinks. Within a century of cessation of emissions the terrestrial biosphere transitions from a carbon sink to carbon source, a process exacerbated by the existence of permafrost carbon pool.
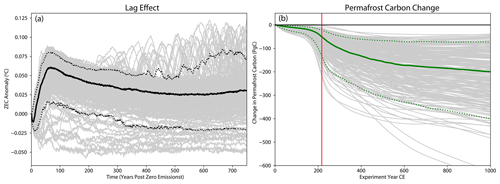
Figure 7(a) Difference in ZEC between the SSP4-6.0-based 1000 Pg C experiment and the standard ZECMIP A1 (1000 Pg C) experiment. (b) Change in the soil carbon held in permafrost regions relative to pre-industrial size under the SSP4-6.0-based experiment. Vertical red line marks the time emissions cease. Grey lines are individual model variants, solid line is the median of the variants, and dashed lines are the 5th and 95th percentile.
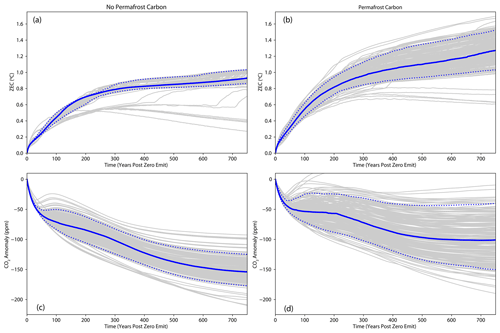
Figure 8(a, b) Zero Emission Commitment (ZEC) for model versions without (a) and with (b) a permafrost carbon pool forced by the 2000 Pg C A3 experiment. ZEC is temperature change relative to the year emissions cease. (c, d) Change in atmospheric CO2 concentration relative to year emissions cease for model versions without (c) and with (d) a permafrost carbon pool forced by the A3 (2000 Pg C) experiment. Grey lines are individual model variants, solid line is the median of the variants, and dashed lines are the 5th and 95th percentile.
Table 3Correlation coefficients between perturbed model parameters and the anomaly in ZEC 50 years after emissions cease. Stronger correlation indicates increased influence for a given parameter. MRT is mean residence time.
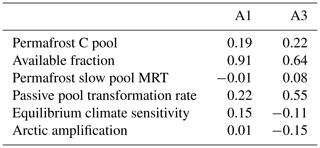
Figure 7 shows the difference in ZEC between simulations following the A1 (1000 Pg C) tier-1 ZECMIP experiment protocol and the experiment where emissions follow historical and SSP4-6.0 emissions until 1000 Pg C of CO2 has been emitted. In the SSP4-6.0-based experiment ZEC is 0.04 [0 to 0.06] ∘C, 0.03 [−0.01 to 0.05] ∘C, and 0.03 [−0.02 to 0.08] ∘C warmer 50, 100, and 500 years after emission cease, respectively. The slightly larger ZEC is being driven by additional carbon being released from permafrost soils under the SSP4-6.0-based experiment with 51 [22 to 132] Pg C released when emissions cease compared to 29 [10 to 90] Pg C in the A1 experiment; 50, 100, and 500 years after emissions cease 84 [39 to 204] Pg C, 107 [50 to 230] Pg C, and 180 [71 to 354] Pg C have been released under the SSP4-6.0-based experiment compared to 73 [32 to 190] Pg C, 100 [46 to 222] Pg C, and 178 [70 to 346] Pg C under the A1 experiment, respectively. Notably the effect of the thaw-lag diminishes with time after emissions cease.
The ZEC for model variants without and with permafrost carbon for the A3 (2000 Pg C) experiments is shown in Fig. 8. Similar to the A1 experiment the existence of a permafrost carbon pool adds to the magnitude of ZEC. The difference between the model versions with and without permafrost carbon is shown in Fig. 5b for the A3 experiment. The difference in ZEC 50, 100, and 500 years after emissions cease is 0.06 [0.03 to 0.12] ∘C, 0.09 [0.05 to 0.18] ∘C, and 0.24 [0.11 to 0.50] ∘C (Table 2), respectively, corresponding to a release of carbon from permafrost soils of 84 [40 to 213] Pg C when emissions cease and 159 [85 to 300] Pg C, 205 [114 to 354] Pg C, and 312 [148 to 505] Pg C 50, 100, and 500 years after emissions cease (Fig. 6b, Table 2). Consistent with previous results that examined representative concentration pathway scenarios, the temperature effect of the permafrost carbon cycle feedback is not strongly effected by the total cumulative emissions (e.g. MacDougall et al., 2012; Schneider von Deimling et al., 2015).
To explore which of the perturbed parameters has the greatest effect on the anomaly in ZEC created by the inclusion of permafrost carbon, correlations were computed between the perturbed parameter values and the anomaly in ZEC 50 years after emissions cease. These correlations are shown in Table 3. For the A1 (1000 Pg C) experiment the available fraction parameter has by far the strongest influence with a correlation of 0.91. None of the other parameters have large correlations. For the A3 (2000 Pg C) experiment both the available fraction (r=0.64) and the passive pool transformation rate (r=0.55) have substantial correlation values. These results contrast those for release of carbon from permafrost soils under future scenarios computed by MacDougall and Knutti (2016), where both available fraction and equilibrium climate sensitivity played the most important roles, and the passive pool transformation rate had little effect on results. The difference may partly be due to the reduced uncertainty range in equilibrium climate sensitivity used here. The prominence of the Passive pool transformation rate in the A3 experiment results is concerning as this parameter is the most poorly constrained of all parameters considered. The available fraction parameter is effectively the combined size of the fast and slow pools as conceptualized in incubation experiments (MacDougall and Knutti, 2016). Thus these results suggest that increased field sampling of, and incubation experiments on, permafrost carbon could substantially reduce the uncertainty in permafrost carbon's contribution to ZEC.
ZECMIP found that the inter-model range of ZEC 50 years after emissions cease for the A1 (1000 Pg C) experiment is −0.36 to 0.29 ∘C with a median value of −0.05 ∘C (MacDougall et al., 2020). Thus the additional warming expected from the permafrost carbon cycle feedback of 0.06 [0.02 to 0.14] ∘C directly and 0.04 [0 to 0.06] ∘C from the thaw-lag effect will not substantially change the expected value of ZEC on decadal timescales. Thus, the overall conclusion that ZEC will be close to zero in the decades following cessation of emissions remains unchanged.
Here we have assessed the parameter uncertainty of the permafrost carbon cycle feedback contribution to ZEC and have left structural uncertainty unassessed. To quantify structural uncertainty other Earth system models would have to conduct ZECMIP experiments with and without their permafrost components turned on. As many of the models that participated in ZECMIP do have permafrost carbon capable versions of their models (e.g. Burke et al., 2012), such a study is possible and would make a valuable contribution to the next iteration of ZECMIP.
Here we found that the permafrost carbon feedback contribution to ZEC was insensitive to cumulative CO2 emissions, despite a larger release of CO2 to the atmosphere from permafrost soils in the A3 (2000 Pg C) experiment. The linear relationship between cumulative emissions of CO2 and global temperature change is generated by an atmosphere–ocean phenomenon (MacDougall, 2017); thus the atmosphere–ocean system does not distinguish between emissions from the terrestrial biosphere and fossil fuel emissions (Simmons and Matthews, 2016). Therefore this insensitivity of temperature to carbon released from permafrost soils appears anomalous. However, for intermediate complexity models like the UVic ESCM the cumulative emissions of CO2 vs. global temperature change curve are only approximately linear and the change in temperature with a unit of CO2 emitted declines at high cumulative emission totals, as the logarithmic radiative forcing from CO2 begins to become the dominate effect (MacDougall and Friedlingstein, 2015). Thus the emissions of CO2 from permafrost soil are less effective at warming after 2000 Pg C of CO2 has been emitted than when 1000 Pg C has been emitted in the UVic ESCM. However, it has been shown the full Earth system models do not have non-linear cumulative emissions of CO2 vs. global temperature change curves (Tokarska et al., 2016). Therefore the insensitivity of the permafrost carbon feedback contribution to ZEC to cumulative CO2 emissions found here should be treated with caution.
To date no Earth system model (McGuire et al., 2018) accounts for abrupt thaw processes in permafrost systems. These processes, including thermokarst production, active hill slope erosion, and coastal erosion, could accelerate thaw processes by 40 % over the next few centuries (Turetsky et al., 2020). Additionally the 2.9pf version of the UVic ESCM (used here) and publicly available 2.10 version of the UVic ESCM do not account for enhanced methane production from permafrost thaw. A methane production scheme has recently been added to a newly developed thread of the model, which preliminarily suggests a warming effect from CH4 production from permafrost soils of 0 to 0.24 ∘C, depending on parameter values and scenario followed (Nzotungicimpaye, 2021). Such values are consistent with expert assessment of (Schuur et al., 2015). Accounting for these processes will likely increase the estimated effect of the permafrost carbon cycle feedback on ZEC, and therefore the effect of the permafrost carbon feedback on ZEC should be reassessed when these processes are better accounted for in Earth system models.
UVic ESCM 2.10 was one of the two models that participated in ZECMIP that included a permafrost carbon scheme (the other was CESM). The ZEC 50 years after CO2 emissions cease for the A1 experiment (1000 Pg C) was 0.03 ∘C for the model version that participated in ZECMIP (UVic ESCM 2.10). This value places UVic ESCM close to the centre of the inter-model range ranking 8th highest of the 18 models that participated in ZECMIP (MacDougall et al., 2020). The model version with permafrost carbon used here ZEC 50 years after emissions cease is −0.02 [−0.07 to 0.08] ∘C; 750 years after emission cease ZEC is 0.70 [0.35 to 1.06] ∘C for the A1 experiment for UVic ESCM 2.9pf and was 0.20 ∘C for UVic ESCM 2.10 in MacDougall et al. (2020). Evidently the two model versions have similar decadal ZEC values but diverge substantially on centennial timescales. The main difference between UVic ESCM versions 2.9pf and 2.10 is the representation of the ocean (Mengis et al., 2020), with the newer version of the model having substantially improved ocean dynamics and a state-of-the-art representation of ocean biogeochemistry (Mengis et al., 2020). Ocean heat and carbon uptake are two of the processes that determine the value of ZEC (MacDougall et al., 2020). Therefore, it is not unexpected that differences in the representation of the ocean would change the ZEC value of a model.
Here we have used a perturbed parameter ensemble with the UVic ESCM to estimate the impact of the permafrost carbon cycle feedback on the value of the CO2 component of the Zero Emissions Commitment. We find 50 years after emissions cease in an experiment where 1000 Pg C of CO2 is emitted to the atmosphere that the permafrost carbon feedback adds 0.06 [0.02 to 0.14] ∘C to ZEC, rising to 0.27 [0.12 to 0.49] ∘C 500 years after emissions cease. Additionally following a more realistic emissions trajectory based on historical and SSP4-6.0 emissions adds 0.04 [0 to 0.06] ∘C to ZEC 50 years after emissions cease. This thaw-lag effect diminishes with time after emissions cease. Overall accounting for the permafrost carbon feedback does not change the conclusion that ZEC will be close to zero on decadal timescales (MacDougall et al., 2020), though the effect of abrupt thaw remains unaccounted for and the feedback is of greater concern over longer timeframes.
The functional form of the climate sensitivity PDF was taken to be the product of two normal-inverse Gaussian functions following Olson et al. (2012). The normal-inverse Gaussian function is as follows:
where μ is location, α is tail heaviness, β is an asymmetry parameter, δ is a scale parameter, and K1 is a modified Bessel function of the third kind (Olson et al., 2012). The Python scipy.stats software package was used to compute the PDF.
The new parameter values fitting the PDF to the Sherwood et al. (2020) constraints on equilibrium climate sensitivity are shown in Table A1 below.
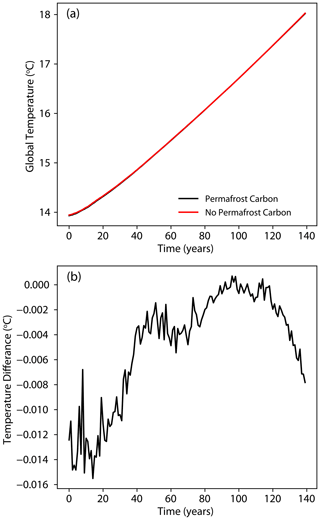
Figure B1Simulations of the 1pctCO2 experiment for two versions of the UVic ESCM one with default settings (permafrost carbon) and a second starting from a model spin-up where cryoturbation diffusion parameter has been set to zero, and hence there is no permafrost carbon pool. Since the 1pctCO2 is a concentration-driven scenario, the presence or absence of permafrost carbon does not have an effect on the atmospheric carbon pool.
UVic ESCM 2.9 and 2.10 are available from http://terra.seos.uvic.ca/model/ (Eby, 2021).
Model output produced for this study is available at https://doi.org/10.5683/SP2/I75BZ0 (MacDougall, 2021).
The contact author has declared that there are no competing interests.
Publisher's note: Copernicus Publications remains neutral with regard to jurisdictional claims in published maps and institutional affiliations.
I am grateful for support from the Natural Sciences and Engineering Research Council of Canada Discovery Grant program and for computational support from Compute Canada. Chris Jones and Charles Koven provided helpful critiques of an early draft of the manuscript. I thank the two anonymous reviewers for their helpful comments.
This research has been supported by the Natural Sciences and Engineering Research Council of Canada.
This paper was edited by Alexey V. Eliseev and reviewed by two anonymous referees.
Archer, D.: A data-driven model of the global calcite lysocline, Global Biogeochem. Cy., 10, 511–526, 1996. a
Avis, C. A.: Simulating the present-day and future distribution of permafrost in the UVic Earth system climate model, PhD thesis, University of Victoria, 2012. a, b
Avis, C. A., Weaver, A. J., and Meissner, K. J.: Reduction in areal extent of high–latitude wetlands in response to permafrost thaw, Nat. Geosci., 4, 444–448, https://doi.org/10.1038/ngeo1160, 2011. a, b
Burke, E. J., Hartley, I. P., and Jones, C. D.: Uncertainties in the global temperature change caused by carbon release from permafrost thawing, The Cryosphere, 6, 1063–1076, https://doi.org/10.5194/tc-6-1063-2012, 2012. a
Cox, P. M., Betts, R. A., Jones, C. D., Spall, S. A., and Totterdell, I. J.: Modelling vegetation and the carbon cycle as interactive elements of the climate system, Proceedings of the RMS millennium conference, available at: http://terra.seos.uvic.ca/model/common/HCTN_23.pdf (last access: 8 September 2021), 2001. a
Eby, M.: Earth System Climate Model UVic ESCM, SEOS [code], available at: http://terra.seos.uvic.ca/model/, last access: 7 September 2021. a
Eyring, V., Bony, S., Meehl, G. A., Senior, C. A., Stevens, B., Stouffer, R. J., and Taylor, K. E.: Overview of the Coupled Model Intercomparison Project Phase 6 (CMIP6) experimental design and organization, Geosci. Model Dev., 9, 1937–1958, https://doi.org/10.5194/gmd-9-1937-2016, 2016. a, b, c, d
Forest, C. E., Stone, P. H., Sokolov, A. P., Allen, M. R., and Webster, M. D.: Quantifying uncertainties in climate system properties with the use of recent climate observations, Science, 295, 113–117, 2002. a
Friedlingstein, P., O'Sullivan, M., Jones, M. W., Andrew, R. M., Hauck, J., Olsen, A., Peters, G. P., Peters, W., Pongratz, J., Sitch, S., Le Quéré, C., Canadell, J. G., Ciais, P., Jackson, R. B., Alin, S., Aragão, L. E. O. C., Arneth, A., Arora, V., Bates, N. R., Becker, M., Benoit-Cattin, A., Bittig, H. C., Bopp, L., Bultan, S., Chandra, N., Chevallier, F., Chini, L. P., Evans, W., Florentie, L., Forster, P. M., Gasser, T., Gehlen, M., Gilfillan, D., Gkritzalis, T., Gregor, L., Gruber, N., Harris, I., Hartung, K., Haverd, V., Houghton, R. A., Ilyina, T., Jain, A. K., Joetzjer, E., Kadono, K., Kato, E., Kitidis, V., Korsbakken, J. I., Landschützer, P., Lefèvre, N., Lenton, A., Lienert, S., Liu, Z., Lombardozzi, D., Marland, G., Metzl, N., Munro, D. R., Nabel, J. E. M. S., Nakaoka, S.-I., Niwa, Y., O'Brien, K., Ono, T., Palmer, P. I., Pierrot, D., Poulter, B., Resplandy, L., Robertson, E., Rödenbeck, C., Schwinger, J., Séférian, R., Skjelvan, I., Smith, A. J. P., Sutton, A. J., Tanhua, T., Tans, P. P., Tian, H., Tilbrook, B., van der Werf, G., Vuichard, N., Walker, A. P., Wanninkhof, R., Watson, A. J., Willis, D., Wiltshire, A. J., Yuan, W., Yue, X., and Zaehle, S.: Global Carbon Budget 2020, Earth Syst. Sci. Data, 12, 3269–3340, https://doi.org/10.5194/essd-12-3269-2020, 2020. a
Fyke, J., Eby, M., Mackintosh, A., and Weaver, A.: Impact of climate sensitivity and polar amplification on projections of Greenland Ice Sheet loss, Clim. Dynam., 43, 2249–2260, 2014. a
Gasser, T., Kechiar, M., Ciais, P., Burke, E., Kleinen, T., Zhu, D., Huang, Y., Ekici, A., and Obersteiner, M.: Path-dependent reductions in CO2 emission budgets caused by permafrost carbon release, Nat. Geosci., 11, 830–835, 2018. a
Hare, B. and Meinshausen, M.: How much warming are we committed to and how much can be avoided?, Climatic Change, 75, 111–149, 2006. a
Hugelius, G., Strauss, J., Zubrzycki, S., Harden, J. W., Schuur, E. A. G., Ping, C.-L., Schirrmeister, L., Grosse, G., Michaelson, G. J., Koven, C. D., O'Donnell, J. A., Elberling, B., Mishra, U., Camill, P., Yu, Z., Palmtag, J., and Kuhry, P.: Estimated stocks of circumpolar permafrost carbon with quantified uncertainty ranges and identified data gaps, Biogeosciences, 11, 6573–6593, https://doi.org/10.5194/bg-11-6573-2014, 2014. a, b, c, d
Jones, C. D., Frölicher, T. L., Koven, C., MacDougall, A. H., Matthews, H. D., Zickfeld, K., Rogelj, J., Tokarska, K. B., Gillett, N. P., Ilyina, T., Meinshausen, M., Mengis, N., Séférian, R., Eby, M., and Burger, F. A.: The Zero Emissions Commitment Model Intercomparison Project (ZECMIP) contribution to C4MIP: quantifying committed climate changes following zero carbon emissions, Geosci. Model Dev., 12, 4375–4385, https://doi.org/10.5194/gmd-12-4375-2019, 2019. a, b, c, d, e
Koven, C., Friedlingstein, P., Ciais, P., Khvorostyanov, D., Krinner, G., and Tarnocai, C.: On the formation of high-latitude soil carbon stocks: Effects of cryoturbation and insulation by organic matter in a land surface model, Geophys. Res. Lett., 36, L21501, https://doi.org/10.1029/2009GL040150, 2009. a
MacDougall, A. H.: The oceanic origin of path-independent carbon budgets, Sci. Rep.-UK, 7, 10373, https://doi.org/10.1038/s41598-017-10557-x, 2017. a
MacDougall, A. H.: Limitations of the 1 % experiment as the benchmark idealized experiment for carbon cycle intercomparison in C4MIP, Geosci. Model Dev., 12, 597–611, https://doi.org/10.5194/gmd-12-597-2019, 2019. a
MacDougall, A. H.: Estimated effect of the permafrost carbon feedback on the zero emissions commitment to climate change, Scholars Portal Dataverse [data set], V3, https://doi.org/10.5683/SP2/I75BZ0, last access: 7 September 2021. a
MacDougall, A. H. and Friedlingstein, P.: The origin and limits of the near proportionality between climate warming and cumulative CO2 emissions, J. Climate, 28, 4217–4230, https://doi.org/10.1175/JCLI-D-12-00751.1, 2015. a
MacDougall, A. H. and Knutti, R.: Projecting the release of carbon from permafrost soils using a perturbed parameter ensemble modelling approach, Biogeosciences, 13, 2123–2136, https://doi.org/10.5194/bg-13-2123-2016, 2016. a, b, c, d, e, f, g, h, i, j, k, l, m, n, o, p, q, r, s, t, u, v, w
MacDougall, A. H., Avis, C. A., and Weaver, A. J.: Significant existing commitment to warming from the permafrost carbon feedback, Nat. Geosci., 5, 719–721, https://doi.org/10.1038/NGEO1573, 2012. a, b, c, d
MacDougall, A. H., Frölicher, T. L., Jones, C. D., Rogelj, J., Matthews, H. D., Zickfeld, K., Arora, V. K., Barrett, N. J., Brovkin, V., Burger, F. A., Eby, M., Eliseev, A. V., Hajima, T., Holden, P. B., Jeltsch-Thömmes, A., Koven, C., Mengis, N., Menviel, L., Michou, M., Mokhov, I. I., Oka, A., Schwinger, J., Séférian, R., Shaffer, G., Sokolov, A., Tachiiri, K., Tjiputra, J., Wiltshire, A., and Ziehn, T.: Is there warming in the pipeline? A multi-model analysis of the Zero Emissions Commitment from CO2, Biogeosciences, 17, 2987–3016, https://doi.org/10.5194/bg-17-2987-2020, 2020. a, b, c, d, e, f, g, h, i
Matthews, H. D., Tokarska, K. B., Rogelj, J., Smith, C. J., MacDougall, A. H., Haustein, K., Mengis, N., Sippel, S., Forster, P. M., and Knutti, R.: An integrated approach to quantifying uncertainties in the remaining carbon budget, Communications Earth and Environment, 2, 1–11, 2021. a
McGuire, A. D., Lawrence, D. M., Koven, C., Clein, J. S., Burke, E., Chen, G., Jafarov, E., MacDougall, A. H., Marchenko, S., Nicolsky, D., Peng, S., Rinke, A., Ciais, P., Gouttevin, I., Hayesf, D. J., Jin, D., Krinner, G., Mooren, J. C., Romanovsky, V., Schädel, C., Schaefer, K., Schuurt, E. A. G., and Zhuang, Q.: Dependence of the evolution of carbon dynamics in the northern permafrost region on the trajectory of climate change, P. Natl. Acad. Sci. USA, 115, 201719903, https://doi.org/10.1073/pnas.1719903115, 2018. a, b, c, d
Meissner, K. J., Weaver, A. J., Matthews, H. D., and Cox, P. M.: The role of land–surface dynamics in glacial inception: A study with the UVic Earth System Model, Clim. Dynam., 21, 515–537, 2003. a, b
Mengis, N., Keller, D. P., MacDougall, A. H., Eby, M., Wright, N., Meissner, K. J., Oschlies, A., Schmittner, A., MacIsaac, A. J., Matthews, H. D., and Zickfeld, K.: Evaluation of the University of Victoria Earth System Climate Model version 2.10 (UVic ESCM 2.10), Geosci. Model Dev., 13, 4183–4204, https://doi.org/10.5194/gmd-13-4183-2020, 2020. a, b, c, d
Nzotungicimpaye, C. M.: Investigating the importance of methane for future climate change: wetland methane emissions, the permafrost carbon feedback, and methane mitigation, PhD thesis, Simon Fraser University, Vancouver, Canada, 2021. a
Olson, R., Sriver, R., Goes, M., Urban, N. M., Matthews, H. D., Haran, M., and Keller, K.: A climate sensitivity estimate using Bayesian fusion of instrumental observations and an Earth System model, J. Geophys. Res.-Atmos., 117, D04103, https://doi.org/10.1029/2011JD016620, 2012. a, b, c
O'Neill, B. C., Kriegler, E., Ebi, K. L., Kemp-Benedict, E., Riahi, K., Rothman, D. S., van Ruijven, B. J., van Vuuren, D. P., Birkmann, J., Kok, K., Levy, M., and Soleckim, W.: The roads ahead: Narratives for shared socioeconomic pathways describing world futures in the 21st century, Global Environ. Chang., 42, 169–180, 2017. a, b, c
Rogelj, J., Shindell, D., Jiang, K., Fifita, S., Forster, P., Ginzburg, V., Handa, C., Kheshgi, H., Kobayashi, S., Kriegler, E., Mundaca, L., Seferian, R., and Vilarino, M. V.: Mitigation pathways compatible with 1.5 ∘C in the context of sustainable development, in: Global warming of 1.5 ∘C. An IPCC Special Report on the impacts of global warming of 1.5 ∘C above pre-industrial levels and related global greenhouse gas emission pathways, in the context of strengthening the global response to the threat of climate change, sustainable development, and efforts to eradicate poverty, World Meteorological Organization, Geneva Switzerland, 2018. a
Schädel, C., Schuur, E. A., Bracho, R., Elberling, B., Knoblauch, C., Lee, H., Luo, Y., Shaver, G. R., and Turetsky, M. R.: Circumpolar assessment of permafrost C quality and its vulnerability over time using long-term incubation data, Glob. Change Biol., 20, 641–652, 2014. a, b, c
Schmittner, A., Oschlies, A., Matthews, H. D., and Galbraith, E. D.: Future changes in climate, ocean circulation, ecosystems, and biogeochemical cycling simulated for a business-as-usual CO2 emission scenario until year 4000 AD, Global Biogeochem. Cy., 22, GB1013, https://doi.org/10.1029/2007GB002953, 2008. a, b
Schneider von Deimling, T., Grosse, G., Strauss, J., Schirrmeister, L., Morgenstern, A., Schaphoff, S., Meinshausen, M., and Boike, J.: Observation-based modelling of permafrost carbon fluxes with accounting for deep carbon deposits and thermokarst activity, Biogeosciences, 12, 3469–3488, https://doi.org/10.5194/bg-12-3469-2015, 2015. a, b
Schuur, E., McGuire, A., Schädel, C., Grosse, G., Harden, J., Hayes, D., Hugelius, G., Koven, C., Kuhry, P., Lawrence, D., Natali, S. M., Olefeldt, D., Romanovsky, V. E., Schaefer, K., Turetsky, M. R., Treat, C. C., and Vonk, J. E.: Climate change and the permafrost carbon feedback, Nature, 520, 171–179, 2015. a, b, c
Sherwood, S., Webb, M. J., Annan, J. D., Armour, K., Forster, P. M., Hargreaves, J. C., Hegerl, G., Klein, S. A., Marvel, K. D., Rohling, E. J., Watanabe, M., Andrews, T., Braconnot, P., Bretherton, C., Foster, G., Hausfather, Z., von der Heydt, A., Knutti, R., Mauritsen, T., Norris, J., Proistosescu, C., Rugenstein, M., Schmidt, G., Tokarska, K., and Zelinka, M.: An assessment of Earth's climate sensitivity using multiple lines of evidence, Rev. Geophys., 58, e2019RG000678, 2020. a, b, c
Simmons, C. and Matthews, H.: Assessing the implications of human land-use change for the transient climate response to cumulative carbon emissions, Environ. Res. Lett., 11, 035001, https://doi.org/10.1088/1748-9326/11/3/035001, 2016. a
Smith, L.: Chaos: a very short introduction, Oxford University Press, London, United Kingdom, 2007. a, b, c
Tokarska, K. B., Gillett, N. P., J.Weaver, A., Arora, V. K., and Eby, M.: The climate response to five trillion tonnes of carbon, Nat. Clim. Change, 6, 851–855, https://doi.org/10.1038/NCLIMATE3036, 2016. a
Trumbore, S.: Age of soil organic matter and soil respiration: radiocarbon constraints on belowground C dynamics, Ecol. Appl., 10, 399–411, 2000. a
Turetsky, M. R., Abbott, B. W., Jones, M. C., Anthony, K. W., Olefeldt, D., Schuur, E. A., Grosse, G., Kuhry, P., Hugelius, G., Koven, C., Lawrence, D. M., Gibson, C., Sannel, A. B. K., and McGuire, A. D.: Carbon release through abrupt permafrost thaw, Nat. Geosci., 13, 138–143, 2020. a
United Nations: Paris Agreement: Twenty-first conference of the parties of the United Nations Framework Convention on Climate Change, United Nations, Geneva, Switzerland, 2015. a
Weaver, A. J., Eby, M., Wiebe, E. C., ans P. B. Duffy, C. M. B., Ewen, T. L., Fanning, A. F., Holland, M. M., MacFadyen, A., Matthews, H. D., Meissner, K. J., Saenko, O., Schmittner, A., Wang, H., and Yoshimori, M.: The UVic Earth System Climate Model: Model description, climatology, and applications to past, present and future climates, Atmos. Ocean, 39, 1–67, 2001. a, b
Zickfeld, K., Eby, M., Matthews, H. D., and Weaver, A. J.: Setting cumulative emissions targets to reduce the risk of dangerous climate change, P. Natl. Acad. Sci. USA, 106, 16129–16134, 2009. a
- Abstract
- Introduction
- Methods
- Results
- Discussion
- Conclusions
- Appendix A: Probability distribution function for climate sensitivity
- Appendix B: Effect of existence of permafrost carbon pool on simulated global climate
- Code availability
- Data availability
- Competing interests
- Disclaimer
- Acknowledgements
- Financial support
- Review statement
- References
- Abstract
- Introduction
- Methods
- Results
- Discussion
- Conclusions
- Appendix A: Probability distribution function for climate sensitivity
- Appendix B: Effect of existence of permafrost carbon pool on simulated global climate
- Code availability
- Data availability
- Competing interests
- Disclaimer
- Acknowledgements
- Financial support
- Review statement
- References