the Creative Commons Attribution 4.0 License.
the Creative Commons Attribution 4.0 License.
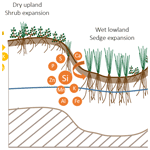
Changing sub-Arctic tundra vegetation upon permafrost degradation: impact on foliar mineral element cycling
Elisabeth Mauclet
Yannick Agnan
Catherine Hirst
Arthur Monhonval
Benoît Pereira
Aubry Vandeuren
Maëlle Villani
Justin Ledman
Meghan Taylor
Briana L. Jasinski
Edward A. G. Schuur
Sophie Opfergelt
Arctic warming and permafrost degradation are modifying northern ecosystems through changes in microtopography, soil water dynamics, nutrient availability, and vegetation succession. Upon permafrost degradation, the release of deep stores of nutrients, such as nitrogen and phosphorus, from newly thawed permafrost stimulates Arctic vegetation production. More specifically, wetter lowlands show an increase in sedges (as part of graminoids), whereas drier uplands favor shrub expansion. These shifts in the composition of vegetation may influence local mineral element cycling through litter production. In this study, we evaluate the influence of permafrost degradation on mineral element foliar stocks and potential annual fluxes upon litterfall. We measured the foliar elemental composition (Al, Ca, Fe, K, Mn, P, S, Si, and Zn) of ∼ 500 samples of typical tundra plant species from two contrasting Alaskan tundra sites, i.e., an experimental sedge-dominated site (Carbon in Permafrost Experimental Heating Research, CiPEHR) and natural shrub-dominated site (Gradient). The foliar concentration of these mineral elements was species specific, with sedge leaves having relatively high Si concentration and shrub leaves having relatively high Ca and Mn concentrations. Therefore, changes in the species biomass composition of the Arctic tundra in response to permafrost thaw are expected to be the main factors that dictate changes in elemental composition of foliar stocks and maximum potential foliar fluxes upon litterfall. We observed an increase in the mineral element foliar stocks and potential annual litterfall fluxes, with Si increasing with sedge expansion in wetter sites (CiPEHR), and Ca and Mn increasing with shrub expansion in drier sites (Gradient). Consequently, we expect that sedge and shrub expansion upon permafrost thaw will lead to changes in litter elemental composition and therefore affect nutrient cycling across the sub-Arctic tundra with potential implications for further vegetation succession.
- Article
(1280 KB) - Full-text XML
-
Supplement
(1387 KB) - BibTeX
- EndNote
Arctic and sub-Arctic ecosystems are typically characterized by persistently frozen ground (soil and/or rock), called permafrost, with a top active layer that seasonally thaws (Burn, 2013; French, 2013). The low soil temperatures and shallow active layers provide constraining environments for vegetation growth and development. Ongoing climate change is driving increases in air temperature, precipitation, and extreme event frequency (such as fires and riverine or coastal flooding; IPCC, 2014) with direct effects on permafrost stability, active layer thickness, and water table depth. For example, in Arctic tundra areas affected by thermokarst processes (i.e., ground subsidence upon ground ice melting; Washburn, 1980), areas of subsidence accumulate water, whereas nearby elevated areas become drier (Schuur et al., 2007).
Arctic and sub-Arctic tundra vegetation is mainly composed of vascular plant functional types such as graminoids, forbs, and deciduous and evergreen shrubs, alongside a large contribution from non-vascular species such as mosses and lichens (Walker et al., 2005). The biomass distribution between Arctic plant functional types responds to the changing water table depth and active layer depth across the permafrost landscape (Heijmans et al., 2022). Upon thermokarst development and increasing microtopography, wetter soil conditions in subsided and poorly drained areas generally favor graminoid expansion (Jorgenson et al., 2015, 2001; van der Kolk et al., 2016), whereas drier soil conditions drive an expansion in shrubs, called shrubification (Jonsdottir et al., 2005; Shaver et al., 2001). Additionally, an increase in air and soil temperatures also influences nutrient availability for vegetation by releasing nutrients from deeper mineral horizons upon permafrost thaw (Beermann et al., 2017; Keuper et al., 2017; Sistla et al., 2013) and stimulating litter microbial decomposition (Hobbie, 1996; Lavoie et al., 2011; Nadelhoffer et al., 1991; Rustad et al., 2001). In nutrient-limited ecosystems, such as Arctic and sub-Arctic tundra, an increase in nutrient availability (e.g., N and P) may strongly contribute to boost vegetation biomass and productivity and further drive shifts in species composition (Dormann and Woodin, 2002; Jonasson et al., 1999; Mack et al., 2004; Nadelhoffer et al., 1991; Van Wijk and Williams, 2003; Walker et al., 2006).
The changes in Arctic and sub-Arctic tundra vegetation composition and productivity may generate important feedbacks on climate change (Heijmans et al., 2022), such as modifying the surface energy balance (Chapin et al., 2005; Sturm et al., 2001a) and changing the net ecosystem C balance (Billings, 1987; Mack et al., 2004; Natali et al., 2019; Schuur et al., 2015, 2008; Shaver et al., 1992). Therefore, ongoing efforts to model vegetation dynamics in these regions are key to improve the Earth system models used to predict the rate of climate change (Druel et al., 2019).
One missing factor in the efforts to model vegetation dynamics is the impact of vegetation changes on nutrient cycling (Druel et al., 2019). As pools of nutrients are limited by the presence of permafrost, nutrient cycling in the active layer provides a crucial source of nutrients to vegetation. Besides the major vegetation organic constituents (C, H, O), mineral nutrients can be sorted into essential macronutrients (e.g., N, P, K, Ca, S), micronutrients (e.g., Fe, Mn, Zn), and non-essential nutrients (e.g., Si, Al) (Marschner, 2012). These elements support physiological functions in the vegetation, such as biomolecule formation, process regulation, and metabolic reactions (Marschner, 2012). As plants take up mineral nutrients from soil to sustain vital functions for growth and development, they play a central role in the biogeochemical cycling and flux of nutrients between soil and plant tissues through root uptake, seasonal leaf senescence, and litterfall. In Arctic and sub-Arctic tundra, the decomposition rates of plant residues are relatively low because of the cold, and they vary according to the plant functional types, with a decreasing order of decomposition rates: sedges > deciduous shrubs > evergreen shrubs (Hobbie, 1996; Parker et al., 2018). Therefore, shifts in vegetation (e.g., sedge or shrub expansion) may accelerate or slow the litter decomposition and further influence the recycling of mineral nutrients.
Many studies across the Arctic have focused on the nutrients nitrogen (N) and phosphorous (P) because of their large requirement for plant development and their limited availability for plants in permafrost regions (Chapin et al., 1995; Finger et al., 2016; Hewitt et al., 2019; Jonasson, 1983; Keuper et al., 2012; Mack et al., 2010; Salmon et al., 2016; Shaver and Chapin, 1991; Zamin et al., 2014), as well as a few on Si (Carey et al., 2019, 2017) and K, Ca, and Mg (Chapin et al., 1980; Jonasson, 1983). To our knowledge, little attention has been paid to the influence of Arctic warming on the plant tissue accumulation of these mineral nutrients and others (i.e., Al, Ca, Fe, K, Ca, Mn, S, Si, and Zn) and to their associated annual return to the soil upon litterfall. According to their physiological and biogeochemical characteristics, Arctic and sub-Arctic tundra plant species display diverse strategies for mineral element uptake and accumulation into their tissues (Chapin, 1980; Marschner, 2012). Therefore, there is a need to investigate the variability in mineral element distribution among the tundra plant species and the cycling rate of these elements by species, information required to improve simulations of vegetation dynamics in the changing northern ecosystems (Druel et al., 2019) and to feed emerging frameworks for spatial ecosystem ecology (Leroux et al., 2017).
In this study, we evaluate how changes in vegetation biomass composition, driven by permafrost degradation, may influence the mineral element fluxes between vegetation tissues and soil litter in a typical sub-Arctic moist acidic tundra. Specifically, we consider the individual contributions of typical plant species from Arctic and sub-Arctic tundra to mineral element stocks and fluxes. Our study investigates tundra species-specific foliar elemental composition to address the following research question: what is the influence of a shift in vegetation biomass composition on the total mineral element foliar stocks at site scale? We hypothesize that the abundance of sedge or shrub species will be the main controlling factor on the vegetation foliar elemental composition and thereby on foliar stocks. We test this hypothesis at a wet-sedge-dominated site of experimental permafrost warming and at a dry-shrub-dominated site of natural permafrost warming. Based on the data, we investigate the influence of a shift in vegetation biomass composition on the maximum potential fluxes of mineral elements from plant leaves to soil litter upon annual leaf senescence and litterfall.
2.1 Study site
The study was conducted in the northern foothills of the Alaska Range in the sub-Arctic tundra at the site of Eight Mile Lake (EML) in Healy, Alaska, USA (63∘52′42′′ N, 149∘15′12′′ W; Schuur et al., 2009). The site is in the discontinuous permafrost zone (Natali et al., 2011; Osterkamp et al., 2009). Climate is characterized by mean monthly temperatures ranging from +16 ∘C in December to −15 ∘C in July (Natali et al., 2011; Vogel et al., 2009) and average annual precipitation of 381 mm (2007–2017; https://www.usclimatedata.com/climate/healy/alaska, last access: February 2020). Soils are characterized by a 35 to 55 cm thick organic layer (> 20 % of organic C content) at the surface, lying above a cryoturbated mineral soil (5 %–20 % of organic C) composed of glacial till and loess parent material (Hicks Pries et al., 2012; Osterkamp et al., 2009; Vogel et al., 2009). The site is located on moist acidic tundra, with a dominance of tussock-forming sedges, such as Eriophorum vaginatum L. and Carex bigelowii Torr. ex Schwein, evergreen shrubs (e.g., Andromeda polifolia L., Rhododendron tomentosum Harmaja, Vaccinium vitis-idaea L., and Empetrum nigrum L.), deciduous shrubs (e.g., Vaccinium uliginosum L. and Betula nana L.), and forbs (e.g., Rubus chamaemorus L.). Non-vascular plant cover is dominated by mosses (mainly Sphagnum spp., Dicranum spp., and feather mosses including Hylocomium splendens and Pleurozium schreberi) and lichen species (e.g., Nephroma spp., Cladonia spp., and Flavocetraria cucullata) (Deane-Coe et al., 2015; Natali et al., 2012; Schuur et al., 2007).
More specifically, this study relied on two contrasted sites at EML, with an experimental warming project (Carbon in Permafrost Experimental Heating Research, CiPEHR) and natural gradient of warming (Gradient) (Fig. 1). The experimental setup at the CiPEHR site was established in 2008 and gathered 48 plots distributed equally into four different artificial warming treatments that are applied on top of a natural background of warming. Treatments will be referred to as follows: control (12 plots with no artificial warming treatment), summer warming (12 plots subjected to summer air warming only), winter warming (12 plots subjected to winter soil warming only), and annual warming (12 plots subjected to both summer and winter warming). An accurate description of the experimental design can be found in Natali et al. (2011). Since the experimental was set up in 2008, warming treatments have amplified the rate of permafrost degradation, with permafrost thaw depth increasing by 2 cm a−1 in control areas and 6 cm a−1 in soil warming areas (Mauritz et al., 2017). Moreover, the CiPEHR site displayed shallower water tables upon permafrost degradation and soil subsidence processes between 2009 and 2017, resulting in wetter soil conditions in 2017 than in 2009 (Rodenhizer et al., 2020) and an increase in sedge biomass over time (Taylor et al., 2018).
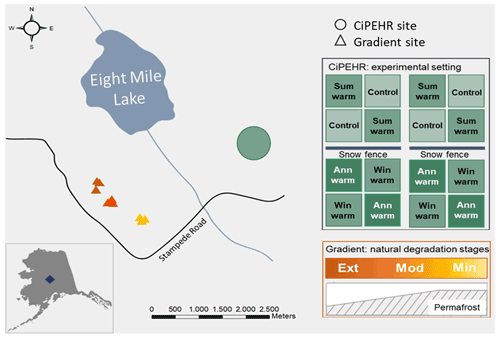
Figure 1Study site at Eight Mile Lake, in Central Alaska, USA. The experimental CiPEHR site (Carbon in Permafrost Experimental Heating Research, in green) presents four treatments: control, summer air warming (Sum warm) with open top chamber settings, winter soil warming (Win warm) with snow fences that insulate the soil upon snow accumulation, and annual warming (Ann warm) with both air and soil warming. The Gradient site is a natural thermokarst gradient composed of three contrasted areas in terms of permafrost degradation with minimal (Min), moderate (Mod), and extensive (Ext) permafrost degradation (yellow to orange triangles). Source: Esri, HERE, Garmin, OpenStreetMap contributors, and GIS user community (February 2021).
The Gradient site is a natural gradient in permafrost thaw and thermokarst formation that has developed at EML since the middle to late 1980s (Schuur et al., 2007, 2009). Recent Bonanza Creek LTER database (https://www.lter.uaf.edu/data, last access: March 2021) reports contrasted maximum active layer thicknesses and water table depths for the “Minimal”, “Moderate”, and “Extensive” thaw areas (measurements in 2019), which reflect different stages of permafrost degradation towards drier soil conditions. Since the beginning of the monitoring (in 1990), the Minimal thaw area showed less ground subsidence and little-disturbed moist acidic tundra and is dominated by the sedge E. vaginatum and Sphagnum spp. mosses, coexisting with evergreen and deciduous shrubs (Jasinski et al., 2022; Schuur et al., 2007). The Moderate thaw area displays isolated areas of ground subsidence (Schuur et al., 2007) and remains dominated by the sedge E. vaginatum, with a lower moss cover than the Minimal thaw area (Jasinski et al., 2018). The Extensive thaw area is characterized by large-scale ground subsidence leading to an undulating surface microtopography and high heterogeneity in active layer depths and water table depths, with water accumulation in lower areas and drier soil conditions in higher areas. Since the start of the thermokarst development, vegetation cover changed with evergreen and deciduous shrubs (as V. uliginosum and R. tomentosum), and forbs (as R. chamaemorus) became dominant at the expense of tussock forming sedges (Jasinski et al., 2018; Schuur et al., 2007).
2.2 Sampling method
At the CiPEHR site, we collected five of the most abundant vascular plant species from the moist acidic tundra: E. vaginatum, B. nana, V. uliginosum, R. chamaemorus, and V. vitis-idaea. The foliar sampling was performed as one bulk foliar sample per plant species per plot among the 48 treated plots (Table S1 in the Supplement) at peak growing season in July 2009 (n=233, before the experimental warming start) and in July 2017 (n=204, after 8 years of experimental warming). The sampling method was similar between 2009 and 2017 and involved the collection of fully formed green leaves from the current year's growth over an area of ∼ 1 m2 (Jasinski et al., 2018; Natali et al., 2011). At the Gradient site, we were able to collect seven of the most abundant vascular plant species: E. vaginatum, C. bigelowii, B. nana, V. uliginosum, R. chamaemorus, R. tomentosum, and V. vitis-idaea. The foliar sampling was performed as one bulk foliar sample per species per plot among the nine plots distributed equally between the Minimal, Moderate, and Extensive thaw areas (Table S1). We sampled fully formed leaves of each vascular species (n=63) over an area of ∼ 5 m2 at three plots in each thaw area. We also sampled moss (n=3; Sphagnum sp., Brachythecium sp., and Dicranum sp.) and lichen species (n=3; Nephroma sp., Cladonia sp., and Flavocetraria cucullata). The sampling was performed between mid-August and early September 2019 (i.e., at the late-season period). For both sites, leaf samples were dried at 60 ∘C and ground (Jasinski et al., 2022; Natali et al., 2011; Schuur et al., 2007).
2.3 Mineral element analysis
Ex situ mineral element concentrations (Al, Ca, Fe, K, Mn, P, S, Si, and Zn) were determined on the 506 leaf samples (437 at CiPEHR in 2009–2017 and 69 at Gradient in 2019) using the non-destructive portable X-ray fluorescence (pXRF) device Niton XL3t GOLDD+ Analyzer (Thermo Fisher Scientific, Waltham, USA). For the measurement, we fixed a transparent film (prolene 4 µm) at the base of a circular plastic cap, in which we placed the dried and ground foliar sample powder in order to reach ∼ 1 cm of sample thickness (Ravansari et al., 2020; Ravansari and Lemke, 2018). We conducted the analyses in laboratory conditions, using a lead stand to protect the operator from X-rays emission, and the total time of measurement was 90 s. The pXRF-measured concentrations were calibrated with another accurate wet chemistry method using measurements by inductively coupled plasma optical emission spectroscopy (ICP-OES; iCAP 6500, Thermo Fisher Scientific, Waltham, USA) after alkaline fusion for 90 foliar samples (more information in Text S1 and Fig. S1 in the Supplement). Corrected pXRF foliar concentrations of every species at every site are summarized in Mauclet et al. (2021a, b). Raw data were acquired from averaging three individual pXRF measurements and used to get means and standard deviations of site-specific foliar concentrations.
2.4 Data treatment
For both sites (CiPEHR and Gradient), datasets of vegetation aboveground biomass were available for CiPEHR in 2009 and 2017 (Taylor et al., 2018) and for Gradient in 2017 (Jasinski et al., 2018). Aboveground biomasses were determined by a non-destructive point frame method (Natali et al., 2012; Schuur et al., 2007; Shaver et al., 2001) in late July. For vascular plant species, we converted the aboveground biomasses to foliar biomasses using species-specific ratios (r) (Table S2; Salmon et al., 2016; Schuur et al., 2016). For moss and lichen species, we assumed a ratio between foliar biomass and aboveground biomass of 1. The foliar biomass (FB) was calculated with Eq. (1):
with FB being the foliar biomass (g m−2), AB the aboveground biomass (g m−2), and r the ratio between foliar biomass and aboveground biomass (unitless).
The estimation of mineral element foliar stocks (FSs) was then calculated using Eq. (2):
with FS being mineral element foliar stock (mg m−2), FC the elemental foliar concentration (mg kg−1), and FB the foliar biomass (g m−2). We further calculated standard deviations (σFS) in order to assess the heterogeneity of mineral element foliar stocks among species and across the different sites with Eq. (3):
For the estimation of foliar fluxes (FFs) at an annual timescale, we used ratios of aboveground productivity that were evaluated over several growing seasons at EML (Schuur et al., 2016). Therefore, we considered that the sub-Arctic tundra at EML was at its equilibrium, with the annually produced biomass (net primary productivity, NPP) equivalent to the senescing biomass (biomass returning to the soil litter). The estimation of annual FFs of mineral elements from standing leaf to foliar litter was calculated using Eq. (4):
with FF being the mineral element foliar flux (mg m−2 a−1), FC the elemental foliar concentration (mg kg−1), and fNPP the foliar NPP (g m−2 a−1).
In particular, the foliar NPP (fNPP) was evaluated for each species and each warming treatment (at CiPEHR) or thermokarst gradient area (at Gradient) using the aboveground biomass data (CiPEHR: Taylor et al., 2018; Gradient: Jasinski et al., 2018) and the ratios of plant species foliar production established by Schuur et al. (2007). Data are presented in the Appendices (Table S4).
Standard deviations of annual foliar fluxes (σFF) were calculated using Eq. (5):
At CiPEHR, data of elemental foliar concentration (FC), foliar biomass (FB), and thereby foliar NPP (fNPP) were available for each foliar sample among the five vascular plant species and the 48 plots subjected to artificial warming treatment in 2009 and 2017. Therefore, we used these specific parameters (FC, FB, and fNPP) to average FS and FF for each plant species belonging to the same treatment and year. At the Gradient site, the different experimental design led us to average the parameters FC, FB, and fNPP for each (vascular and non-vascular) species belonging to the same area of permafrost thaw gradient (Minimal, Moderate, or Extensive) before calculating FS (Eq. 2) and FF (Eq. 4) for each species at the three thaw gradient areas.
All statistics were performed using R 4.0.2 (R Core Team, 2020) and plots using the ggplot2 package (Wickham, 2016). In each statistical model, we examined the model residuals to test for normality and heteroscedasticity, and data were transformed (log-transformation) when appropriate (Table S5). At CiPEHR, we used mixed-effects models to investigate separately the effects of experimental warming over time on the mineral element foliar stocks and fluxes (Table S5). The mixed-effects models were performed using the lme4 package (Bates et al., 2015) and included a random effect for repeated measurements on individual plots. The models considered are as follows: (i) plot-level foliar stocks and fluxes as the dependent variable, (ii) treatments (summer, winter, and annual warming) and time as covariates, and (iii) interactions between treatments and time to evaluate whether mineral element foliar stocks and fluxes differed in each experimental treatment (Eq. 6). At the Gradient site, we tested the influence of the permafrost degradation (with the Minimal, Moderate, and Extensive thaw areas) on elemental foliar stocks and annual fluxes with a one-way analysis of variance (ANOVA) and a Tukey's honestly significant difference (HSD) post hoc test. For both methods, parameters of significance are shown with 95 % confidence intervals.
with i being the plot and j the year. Specifically, Y stands for the estimate of FS or FF, α corresponds to the intercept, β corresponds to the fixed-effect coefficients, Summer, Winter, Annual, and Year refer to the model covariates, Plot is a random effect variable (), and ε is the residuals ().
3.1 Foliar mineral element concentration of sub-Arctic tundra at Eight Mile Lake
At the experimental warming site (CiPEHR), the average elemental foliar concentrations of the five vascular plant species generally decreased for all treatments in the order Si > K > Ca > Al > P > Mn > S > Fe > Zn in 2009 (Fig. 2a) and in 2017 (Fig. 2b). The foliar elemental abundance in plant functional types remains similar across years, with the exception of an increase in Si and Al foliar concentrations between 2009 and 2017 for the sedge E. vaginatum and the evergreen shrub V. uliginosum. At the thermokarst gradient site (Gradient), elemental foliar concentrations decreased in the order K > Ca > Si > P > Mn > Al > S > Fe > Zn (Fig. 2c). Additional species were included, i.e., the sedge C. bigelowii, the evergreen shrub R. tomentosum, and non-vascular moss and lichen species. C. bigelowii displayed high Si foliar concentration that corroborates the sedge affinity for Si. Moreover, mosses and lichens also displayed singularities in nutrient concentrations compared to vascular species, with high concentrations in Si (up to 6 g kg−1), Al (up to 1.5 g kg−1), and Fe (up to 0.6 g kg−1).
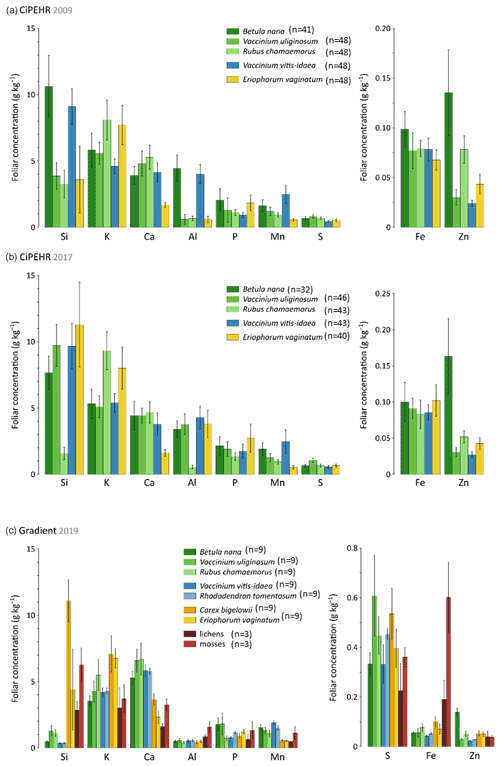
Figure 2Mineral element concentrations (Si, K, Ca, Al, P, Mn, S, Fe, and Zn) in foliage at (a) the experimental warming site (CiPEHR; average over all warming treatments) in 2009 and (b) 2017, as well as (c) the thermokarst gradient site (Gradient; average over all degradation stages) in 2019. Vegetation is sorted by plant functional types: deciduous shrubs and forbs (green), evergreen shrubs (blue), sedges (yellow), and lichens and mosses (red). Error bars represent standard deviations.
Plant functional types showed contrasting nutrient foliar concentrations according to their physiology and growing strategies (Aerts and Chapin, 1999). For instance, high Si foliar concentrations observed in sedge tissues (here in E. vaginatum and C. bigelowii) can be attributed to strategies of Si root uptake (Ma and Takahashi, 2002). Silicon is known to promote better access to light and oxygen by strengthening the leaves (Hodson et al., 2005; Ma and Takahashi, 2002; Quigley et al., 2017). Moreover, the differences in Ca foliar concentrations between sedges and shrubs can be attributed to different Ca requirements for growth between monocotyledons and dicotyledons (Loneragan and Snowball, 1969; Marschner, 2012). A low demand in Ca for monocotyledons may reflect a low capacity for Ca uptake (through Ca2+ binding sites in the cell walls; White and Broadley, 2003), storage, or management into cells. Finally, the accumulation of Si, Al, and Fe in moss and lichen tissues might be induced by the presence of local minerogenic dust deposition (Reimann et al., 2001). Overall, we observed that mineral element foliar concentrations were mostly specific to species, reflecting different vegetation uptake and storage strategies. Therefore, elemental composition of vegetation foliar tissues constitutes a key parameter to further address the objectives of the study, i.e., evaluate how the mineral element foliar stock and litterfall fluxes may evolve upon changing vegetation composition and biomass.
3.2 Influence of wetter soil conditions on mineral element foliar stocks and fluxes
The CiPEHR site was used to test the impact of wetter soils on mineral element foliar stocks and potential annual fluxes (online data: Mauclet et al., 2021a) because the rising water table depths (Rodenhizer et al., 2020) point at an overall wetting of the surface soils with different warming treatments that created favorable conditions for sedge development at the expense of woody shrubs (Taylor et al., 2018).
3.2.1 Influence of wetter soil conditions on mineral element foliar stocks
At CiPEHR, the average elemental foliar stocks in 2009 varied from 800–900 mg m−2 for K to 5–6 mg m−2 for Zn (as K > Si > Ca > P > Al > Mn > S > Fe > Zn), whereas the average elemental foliar stocks in 2017 ranged from 2000–2500 mg m−2 for Si to 10 mg m−2 for Zn (as illustrated for control site in Fig. 3). Upon natural warming (at control site), the foliar stocks in Si, Al, P, S, Fe, and Zn significantly increased over time (p < 0.05; Table S5), with at least a 40 % increase between 2009 and 2017 (Fig. 3). More specifically, the sedge contribution to nutrient foliar stocks has more than doubled over time; it ranged between 25 % (for Al) and 60 % (for K and P) in 2009 and went up to 55 % (for Ca and Mn) and more than 80 % (for Si, Al, K, P, and Fe) in 2017. The effect of the four artificial warming treatments on foliar stocks was evaluated in 2009 and in 2017 for Si, Ca, and Mn (Fig. 4) and for K, Zn, S, P, Fe, and Al (Fig. S2). In 2009 as in 2017, mineral element foliar stocks showed no significant difference (p>0.05) between warming treatments and control sites. Despite the increasing degree of permafrost degradation between the four warming treatments in terms of subsidence and rising water table (control < summer warming < winter warming < annual warming; Rodenhizer et al., 2020), only time had a positive effect on mineral element foliar stocks with total foliar stocks being 1.5 to 5 times higher in 2017 than in 2009 (p<0.05 for almost every mineral element except for Ca and Mn). In particular, total Si foliar stocks ranged from 550–650 mg m−2 in 2009 before reaching 3 to 4 times higher values in 2017 (Fig. 4a). This was induced by the large increase in sedge contribution to Si foliar stocks (from ∼ 40 % in 2009 to ∼ 85 % in 2017), while shrub (B. nana, V. uliginosum, and V. vitis-idaea) and forb (R. chamaemorus) contributions to Si foliar stocks remained relatively constant between 2009 and 2017 (Mauclet et al., 2021a). The total foliar stocks of Ca and Mn (Fig. 4b–c) showed lower increases than Si between 2009 and 2017, with respective increasing factors ranging around 1.3 (Mauclet et al., 2021a).
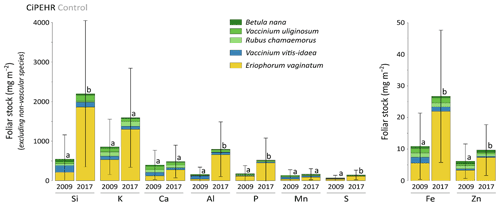
Figure 3Evolution over time of the cumulative foliar stocks of mineral elements (mg m−2) into five vascular plant species across the control area of the experimental warming site (CiPEHR). Vegetation is sorted by plant functional types: deciduous shrubs and forbs (green), evergreen shrubs (blue), and sedges (yellow). Elemental content of mosses and lichens was not measured at the CiPEHR site. Letters correspond to a mixed-effects model analysis and compare the total foliar elemental stocks between 2009 and 2017. Error bars represent standard deviations.
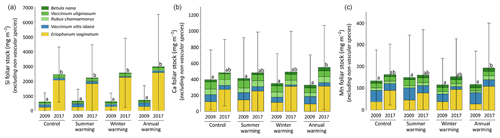
Figure 4Evolution over time of the cumulative foliar stocks (mg m−2) of (a) silicon, (b) calcium, and (c) manganese into five vascular plant species between the four warming treatments of the experimental site (CiPEHR). Vegetation is sorted by plant functional types: deciduous shrubs and forbs (green), evergreen shrubs (blue), and sedges (yellow). Elemental content of mosses and lichens was not measured at the CiPEHR site. The four treatments (control, summer warming, winter warming, and annual warming) refer to no artificial treatment, air warming, soil warming, and both air and soil warming, respectively. Letters correspond to a mixed‐effects model analysis and compare the total foliar elemental stocks between warming treatments and years. Error bars represent standard deviations.
At the CiPEHR site, the major changes in elemental foliar stocks over time were mainly biomass-driven, with the massive increase in E. vaginatum biomass observed across the site over time (from 1.7–3 times higher in 2017 than in 2009; Taylor et al., 2018), upon permafrost soil subsidence and wetter soil conditions (Rodenhizer et al., 2020). Among the mineral elements studied, the massive increase in Si foliar stocks followed (i) the large increase in E. vaginatum biomass (i.e., biomass-driven; Taylor et al., 2018) but also (ii) the increase in sedge Si foliar concentration (i.e., concentration-driven; Fig. 4a) between 2009 and 2017. Conversely, mineral elements that accumulate in higher concentrations into shrubs and forbs than into sedges (such as Ca and Mn; Fig. 2a–b) displayed a lower increase in their total foliar stocks between 2009 and 2017 (Fig. 4b–c). This resulted from the very low increase or decrease in the shrub (B. nana, V. uliginosum, and V. vitis-idaea) and forb species (R. chamaemorus) biomasses over time (Taylor et al., 2018). Overall, the mineral elements concentrated in E. vaginatum foliar tissues (such as Si; Fig. 2a–b) are those showing high increase in their foliar stocks with time as a result of the large sedge expansion upon the artificial warming.
In summary, we show that in degraded permafrost landscapes, with wetter soil conditions and resulting graminoid (including sedge) expansion (van der Kolk et al., 2016), there is a subsequent increase in the sedge contribution to the nutrient foliar stocks. A couple of additional insights should be noted regarding these data. Firstly, the high variability associated with average elemental foliar stocks (Figs. 4 and S2) mostly reflected the important heterogeneity in species foliar biomasses among sites from similar treatment. On average, foliar biomasses had relative standard deviations 2 to 5 times higher than foliar concentrations (Table S3) (Mauclet et al., 2021a). This highlights the large influence of the heterogeneity in vegetation biomass distribution on the foliar stock standard deviation. Secondly, mineral element foliar stock estimates at the CiPEHR site only included contributions from the five most abundant vascular plant species on site, covering ∼ 55 % of the total foliar biomass in 2009 and ∼ 72 % in 2017 (Taylor et al., 2018). Foliar stock estimates did not include non-vascular species (i.e., mosses and lichens), accounting for ∼ 28 % of the foliar biomass in 2009 and ∼ 12 % in 2017. Therefore, the results (Figs. 4 and S2) provide lower estimates compared to the actual total foliar stocks at CiPEHR.
3.2.2 Influence of wetter soil conditions on potential mineral element foliar fluxes
Changes in mineral element foliar stocks described above have important implications for potential changes in the elemental composition of foliar litterfall fluxes. We quantified potential annual elemental foliar fluxes from deciduous species (sedges, deciduous shrubs, and forbs) that lose their leaves each season. We did not include evergreen species because of the complexity of their leaf and tissue lifespan and senescence dynamics (Park et al., 2020; Vitt, 2007).
As we based our foliar litterfall flux calculations on elemental foliar concentrations measured in July (at the peak of the growing season), some mobile nutrients may translocate from mature leaves to storage organs or growing tissues throughout the season (i.e., between the sampling period in July and the actual foliar litterfall fluxes in August). Therefore, we may overestimate total annual foliar fluxes for P and K (Fig. S3) because of their specific retranslocation (Berendse and Jonasson, 1992; Chapin and Shaver, 1989; Chapin et al., 1980; Jonasson and Chapin, 1985). Conversely, our estimates for annual foliar fluxes of non-mobile nutrients, such as Ca and Mn (Chapin et al., 1980; Maillard et al., 2015; Marschner, 2012; White, 2012), are representative of the actual foliar fluxes (Fig. 6b–c). Lastly, there is a lack of consensus or species-dependent complexity regarding mobility of Si, S, Zn, Al, and Fe (Maillard et al., 2015; Marschner, 2012; Shi et al., 2011). For all these reasons, we will further talk about “maximum potential foliar flux” of mineral elements upon litterfall.
In 2009, potential mineral element foliar fluxes varied from 680 mg m−2 a−1 for K to 5 mg m−2 a−1 for Zn and were 2 to 10 times higher in 2017, as illustrated for the control site (Fig. 5). Upon natural warming (at control site), the potential elemental foliar fluxes increased significantly over time (p<0.05, Table S5), with the highest increase for Si and Al (mean of 5 and 10 times, respectively). While the sedge contribution to the potential foliar fluxes went from 40 %–70 % in 2009 (for Ca and P, respectively) and up to 90 % in 2017 (for Si, P, and Al), the shrub and forb contribution to the potential foliar fluxes decreased over time. The effect of the four artificial warming treatments on the potential annual foliar fluxes was evaluated in 2009 and in 2017 for Si, Ca, and Mn (Fig. 6) and for K, Zn, S, P, Fe, and Al (Fig. S3). In 2009 as in 2017, some mineral elements (Ca and Mn: Fig. 6b–c; K, Fe, S, and Zn: Fig. S4) showed differences in their potential annual foliar fluxes with warming treatments. However, this variability in the potential foliar fluxes between the four treatments was much less pronounced than the large increase in the potential elemental foliar fluxes that occurred over time, with total foliar fluxes 1.5 to 10 times higher in 2017 than in 2009 (p<0.05, Table S5). More specifically, the potential annual foliar fluxes of Si increased more than 5 times between 2009 (320–360 mg m−2 a−1) and 2017 (1650–2300 mg m−2 a−1) (Fig. 6a). The main contributors to annual Si foliar fluxes were E. vaginatum (∼ 50 % in 2009 and ∼ 90 % in 2017) and V. uliginosum (15 %–20 % in 2009 and 6 %–10 % in 2017). In parallel, the potential annual foliar fluxes of Ca and Mn (Fig. 6b–c) only increased from 1.3–2.3 times between 2009 and 2017. Potential Ca foliar fluxes increased from 200–285 mg m−2 a−1 in 2009 to 380–390 mg m−2 a−1 in 2017, and potential Mn foliar fluxes increased from ∼ 60–85 mg m−2 a−1 in 2009 to 110–130 mg m−2 a−1 in 2017. In particular, the shrub (mostly V. uliginosum) and forb (R. chamaemorus) contribution was more important to potential annual Ca and Mn foliar fluxes than to Si. It should be noted that the lower standard deviations for annual foliar flux estimates (compared to foliar stock estimates) may be underestimated because we did not integrate standard deviation of the foliar NPP.
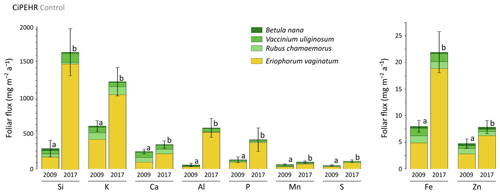
Figure 5Evolution over time of the cumulative annual foliar fluxes (mg m−2 a−1) of mineral elements for the considered species across the control area of the experimental warming site (CiPEHR). Vegetation is sorted by plant functional types: deciduous shrubs and forbs (green) and sedges (yellow). Letters correspond to a mixed-effects model analysis and compare the total foliar elemental stocks between 2009 and 2017. Error bars represent standard deviations.
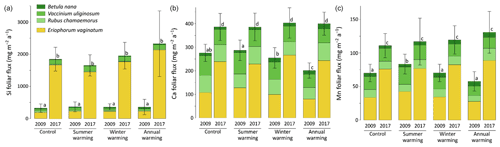
Figure 6Evolution over time of the cumulative annual foliar fluxes (mg m−2 a−1) of (a) silicon, (b) calcium, and (c) manganese for the considered species between the four warming treatments of the experimental site (CiPEHR). Vegetation is sorted by plant functional types: deciduous shrubs and forbs (green) and sedges (yellow). The four treatments (control, summer warming, winter warming, and annual warming) refer to no artificial treatment, air warming, soil warming, and both air and soil warming, respectively. Letters correspond to a mixed‐effect model analysis and compare total foliar elemental fluxes between warming treatments and years. Error bars represent standard deviations.
At the CiPEHR site, the changes in potential mineral element fluxes from plant leaves to soil litter were mainly biomass-driven and induced by the large increase in E. vaginatum foliar biomass (Table S3) and productivity (Table S4). Thereby, the sedge (E. vaginatum) foliar elemental composition largely influenced the patterns of change for every elemental foliar flux over time (Fig. 5). Mineral elements more concentrated in E. vaginatum than in shrub and forb foliar tissues (such as Si; Fig. 2a–b) are those showing high increase in their potential foliar fluxes with time as a result of the large sedge expansion upon the artificial warming. To a lesser extent, some changes in the potential elemental foliar fluxes (i.e., for Si and Al) were also concentration-driven, i.e., emphasized by the increase in Si and Al concentration into sedge foliar tissues (Fig. 2a–b).
In summary, patterns of change in mineral element foliar stocks, and thereby in potential foliar fluxes between vegetation tissues and soil litter, were mostly biomass-driven with the sedge (E. vaginatum) expansion across the site. At the CiPEHR site, the warming experiment generated soil subsidence and wetter soil conditions upon rising water table depths (Rodenhizer et al., 2020), which created favorable conditions for sedge development at the expense of woody shrubs (van der Kolk et al., 2016). Consequently, elements highly concentrated in sedge leaves (here, E. vaginatum), such as Si, P, or Fe (Fig. 2a–b), displayed important changes in their foliar stocks (Figs. 4a and S2), and thereby in their annual foliar fluxes, between 2009 and 2017 (Figs. 6a and S4). On the other hand, elements highly concentrated in deciduous shrubs and/or forbs, such as Ca and Mn (Fig. 2a–b), displayed a more mitigated increase in their foliar stocks (Fig. 4b–c), and thereby in their potential annual foliar fluxes, between 2009 and 2017 (Fig. 6b–c).
3.3 Influence of drier soil conditions on mineral element foliar stocks and fluxes
The Gradient site was used to test the impact of drier, degraded soils on mineral element foliar stocks and fluxes. Upon the thermokarst development, the high variability in active layer depths and water table depths points at an overall drying and deepening of the active layer that generated a vegetation shift towards shrubification (Jasinski et al., 2018; Schuur et al., 2007). In this section, we focus on seven vascular species and two groups of non-vascular species (mosses and lichens) to assess the influence of drier soil conditions upon natural permafrost degradation on mineral element foliar stocks and fluxes from plant leaves to soil (online data: Mauclet et al., 2021b).
3.3.1 Influence of drier soil conditions on mineral element foliar stocks
At the Gradient site, we collected leaf samples late in the growing season when retranslocation of nutrients already occurred from mature leaves to storage organs or growing tissues. As discussed in Sect. 3.2.2, we may therefore underestimate the mineral element foliar stocks (Fig. S3) of mobile mineral elements such as P and K (Berendse and Jonasson, 1992; Chapin et al., 1980; Maillard et al., 2015; Marschner, 2012). For Ca and Mn, which are non-mobile nutrients in plant tissues (Chapin et al., 1980; Maillard et al., 2015; Marschner, 2012; White, 2012), the foliar stock estimations (Fig. 8b–c) can be considered as representative of the actual foliar stocks. For Si, S, Zn, Al, and Fe, for which the mobility remains poorly documented, the foliar stocks of some elements may also be underestimated to a lesser extent.
Total mineral element foliar stocks ranged from ∼ 1350 mg m−2 for K to ∼ 13 mg m−2 for Zn (as illustrated for the Minimal thaw area; Fig. 7). The relative contributions of plant functional types to elemental foliar stocks varied widely across the Gradient site. Overall, mosses and sedges were important contributors to most of the elemental foliar stocks, with moss contribution ranging from 30 % (for Zn and K) to more than 60 % (for Si, Al, and Fe) and sedge contribution ranging from 7 % (for Fe) to 40 % (for Mn). Together, shrubs and forbs accounted for 5 % of Si and Fe foliar stocks and for ∼ 40 % of Ca and Mn foliar stocks. When looking at the different areas spanning the thermokarst development at Gradient, the Minimal, Moderate, and Extensive thaw areas displayed differences in their mineral element foliar stocks (Si, Ca, and Mn in Fig. 8 and K, Al, P, S, Fe, and Zn in Fig. S4) and in the species contribution to these foliar stocks. Firstly, Si foliar stocks (Fig. 8a) reached ∼ 1000 mg m−2 at the Minimal thaw area and decreased to ∼ 635 mg m−2 at the Moderate thaw area and to ∼ 740 mg m−2 at the Extensive thaw area. The major contributors to Si foliar stocks were the mosses and sedges (E. vaginatum and C. bigelowii), with variation in their respective contributions to Si foliar stocks across the thermokarst gradient. The Si foliar stocks at the Minimal and Extensive thaw areas showed higher moss contribution (∼ 50 %–60 %) than sedge (∼ 25 %), while the Moderate thaw area displayed the opposite (sedge contribution ∼ 64 % and moss contribution ∼ 20 %). Secondly, Ca and Mn showed common patterns of foliar stock distribution upon thermokarst development. The Minimal and Extensive thaw areas had similar foliar stocks of Ca (∼ 1015 mg m−2) and Mn (270 mg m−2), and the Moderate thaw area showed lower foliar stocks of Ca (∼ 780 mg m−2) and Mn (∼ 200 mg m−2) (Fig. 8b–c). Despite the differences between the total foliar stocks of Ca and Mn being non-significant (p>0.05), the high Ca and Mn concentrations in shrub and forb foliage (Fig. 2c) resulted in much larger contributions of shrubs and forbs to Ca and Mn foliar stocks (∼ 40 % at the Minimal, ∼ 50 % at the Moderate, and ∼ 60 % at the Extensive thaw area) compared to Si foliar stocks.
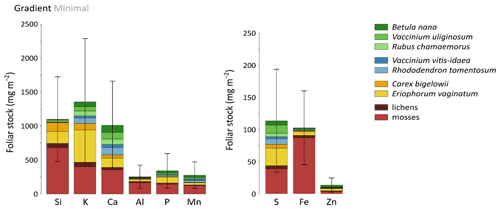
Figure 7Cumulative mineral element stocks (mg m−2) into foliar tissues of vascular species and tissues of non-vascular species across the least degraded area (Minimal) of the thermokarst gradient (Gradient) in 2017. Vegetation is sorted by plant functional types: deciduous shrubs and forbs (green), evergreen shrubs (blue), sedges (yellow), and lichens and mosses (red). Error bars represent standard deviations.
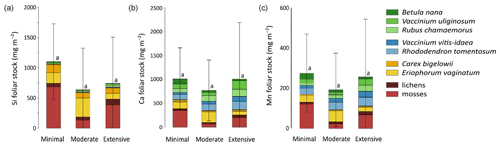
Figure 8Cumulative stocks (mg m−2) of (a) silicon, (b) calcium, and (c) manganese into foliar tissues of vascular species and tissues of non-vascular species between the different permafrost thaw areas from the thermokarst gradient (Gradient) in 2017. Vegetation is sorted by plant functional types: deciduous shrubs and forbs (green), evergreen shrubs (blue), sedges (yellow), and lichens and mosses (red). Stages of permafrost degradation are classified as Minimal, Moderate, and Extensive, based on permafrost thaw and subsidence rate. Letters correspond to a one-way ANOVA test and compare the total foliar elemental stocks between the three stages of permafrost degradation. Error bars represent standard deviations.
At the Gradient site, our results suggest that changes in elemental foliar stocks were biomass-driven and followed the change in vegetation biomass distribution induced by the changing soil conditions upon thermokarst development (Schuur et al., 2007). Drier soil conditions upon thermokarst development initiated a shift in vegetation towards shrub expansion, with shrub and forb aboveground biomasses accounting for ∼ 40 % at the Minimal thaw area and reaching up to ∼ 60 % at the Extensive thaw area (Jasinski et al., 2018). Consequently, mineral elements highly concentrated in shrub and forb leaves, such as Ca and Mn (Fig. 2c), have seen their respective shrub and forb foliar stocks increase upon the early shrubification occurring at the Gradient site. Upon more advanced shrubification, we can expect a significant increase in the Ca and Mn total foliar stocks, led by the shrub foliar contribution. Therefore, our results support that foliar elemental composition (Fig. 2c) and species biomass distribution conjointly influenced the site-specific total mineral element stocks, as well as the relative species contributions to these foliar stocks. The large standard deviations for the mineral element foliar stocks at the Gradient site arose from the large heterogeneity in species foliar biomass, with relative standard deviation between 2 and 10 times higher for foliar biomasses (Table S3) than for foliar concentrations (Mauclet et al., 2021b).
3.3.2 Influence of drier soil conditions on potential mineral element foliar fluxes
Shifts in mineral element foliar stocks upon shrubification may directly affect annual foliar fluxes from plant leaves to soil. To assess the influence of drier soil conditions (upon thermokarst process) on the mineral element cycling, we focused on the five vascular species subjected to seasonal senescence and litterfall (E. vaginatum, C. bigelowii, R. chamaemorus, V. uliginosum, and B. nana). We did not include evergreen species, nor mosses and lichens.
At the least degraded area (Minimal) of the Gradient site, mineral element annual foliar fluxes ranged from 670 mg m−2 a−1 for K to 9–6 mg m−2 a−1 for Fe and Zn (Fig. 9). Depending on the mineral element, the species contribution to annual foliar fluxes varied greatly, but sedge contribution remained important to every annual foliar flux (between 40 % for Ca and 90 % for Si foliar fluxes). The influence of thermokarst development on annual foliar fluxes was evaluated for Si, Ca, and Mn (Fig. 10a–c) and K, Al, P, S, Fe, and Zn (Fig. S5). Firstly, annual Si foliar fluxes were significantly different across thermokarst development (p<0.05) and varied from 390 mg m−2 a−1 at the Moderate thaw area to 220 mg m−2 a−1 at the Extensive thaw area (Fig. 10a). Similar to Si, other mineral elements such as K, Fe, and Zn also showed a significant decrease (p<0.05) in their annual foliar fluxes between the Moderate and Extensive thaw areas (30 % to 40 % decrease), i.e., upon thermokarst development and early shrubification (Fig. S5). Secondly, in contrast to the net decrease in annual Si, K, Fe, and Zn foliar fluxes, Ca and Mn showed similar annual foliar fluxes across the thermokarst gradient sites, with an increasing contribution of shrubs and forbs to these foliar fluxes: from ∼ 45 % at the Moderate to ∼ 75 % at the Extensive thaw area (Fig. 10b–c). It should be noticed that elemental foliar stocks and fluxes at the Gradient site have been estimated with the foliar concentrations measured on leaves sampled in August 2019 and the available foliar biomass and productivity data from July 2017. Although this may add a confounding factor, we have seen that foliar elemental composition was specific to vegetation functional types (Sect. 3.1), well in line with other studies (Aerts et al., 1999; Hobbie and Gough, 2002; Shaver and Chapin, 1991; Urbina et al., 2017).
Considering the thermokarst gradient (i.e., the Minimal, Moderate, and Extensive thaw areas), overall differences in potential elemental foliar fluxes between areas were biomass-driven. For instance, the Moderate thaw area showed the highest total annual Si foliar flux due to high biomass and productivity of Si-rich plant species (such as sedges), whereas the Extensive thaw area showed the lowest total annual Si foliar flux due to high biomass and productivity of Si-poor plant species (such as shrubs). Conversely, annual Ca and Mn foliar fluxes were positively affected by the increase in shrub biomass and productivity observed at the Extensive thaw area. More broadly, deciduous shrubs and forbs showed increasing annual Ca and Mn foliar fluxes upon permafrost degradation and the induced early shrubification (Mauclet et al., 2021b), which therefore offset the simultaneous decrease in sedge contribution. At the Gradient site, where permafrost has been thawing over the past decades and thermokarst has developed (Schuur et al., 2008), differences in vegetation community biomasses suggest that shrubification occurs upon permafrost degradation (Jasinski et al., 2018). Foliar productivity followed the same pattern as foliar biomass, with sedges dominating plant productivity at the Minimal and Moderate thaw areas and shrub dominating plant productivity at the Extensive thaw area. We emphasize that shifts in vegetation biomass distribution and productivity upon shrubification led to an increase in foliar fluxes of mineral elements relatively more concentrated in shrub than sedge foliar tissues (i.e., Ca and Mn) and to a decrease in mineral elements relatively less concentrated in shrub than sedge foliar tissues (i.e., Si, K, Fe, and Zn; Fig. 2c).
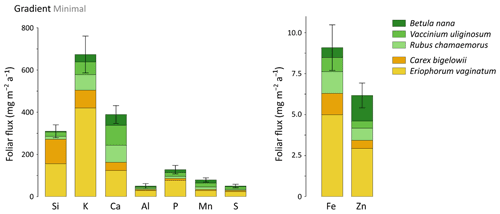
Figure 9Cumulative annual foliar fluxes (mg m−2 a−1) of mineral elements for the considered plant species at the least degraded area (Minimal) of the thermokarst gradient (Gradient) in 2017. Vegetation is sorted by plant functional types: deciduous shrubs and forbs (green) and sedges (yellow). Error bars represent standard deviations.
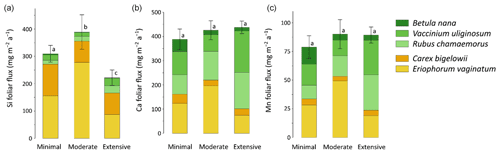
Figure 10Cumulative annual foliar flux (mg m−2 a−1) of (a) silicon, (b) calcium, and (c) manganese for the considered plant species between the different permafrost thaw areas from the thermokarst gradient (Gradient) in 2017. Vegetation is sorted by plant functional types: deciduous shrubs and forbs (green) and sedges (yellow). The stages of permafrost degradation are classified as Minimal, Moderate, and Extensive, based on permafrost thaw and subsidence rate. Letters correspond to a one-way ANOVA test and compare total foliar elemental fluxes between the three stages of permafrost degradation. Error bars represent standard deviations.
3.4 Implications for vegetation shifts in the Arctic and sub-Arctic
Vegetation communities across the Arctic and sub-Arctic are sensitive to a warming environment, as supported by many field studies (Chapin and Shaver, 1985; Shaver, 1996; Viers et al., 2013; Wookey et al., 2009) and satellite observations (Bhatt et al., 2017; Myneni et al., 1997; Pouliot et al., 2009; Xu et al., 2013). Upon changing environmental conditions (i.e., air temperature, growing season length, snow cover, and soil moisture regime), shifts in plant functional group dominance may spatially differ (i.e., shrub or sedge dominance) and have contrasting implications on the surrounding environment (such as effects on heat transfer; e.g., Chapin et al., 2005; Sturm et al., 2001a). Furthermore, vegetation is central to the C balance regulation, and there is a challenging need to assess to what extent the shift in vegetation and thereby in litter elemental composition will accelerate or slow down the litter decomposition (source of C emission), as well as how fast these mineral elements may be available again for vegetation uptake (source of C sequestration). In this section, we discuss the influence of vegetation community structure and composition on mineral element cycling at a larger scale of the Arctic upon (i) shrubification, (ii) graminoid expansion, and (iii) changes in non-vascular species composition.
The shrub expansion across the Arctic is an important and widely observed response of high-latitude ecosystems to rapid climate warming, and the woody shrub cover is projected to increase by as much as 52 % by 2050 (Pearson et al., 2013). In addition to the multiple implications on surrounding ecosystems (e.g., changing the Arctic tundra albedo and soil surface roughness: Chapin et al., 2005; Sturm et al., 2001a; Weintraub and Schimel, 2005), shrubification may also affect biogeochemical cycles. Here, we demonstrate that an increase in shrub biomass may increase the potential annual foliar fluxes of mineral elements highly concentrated in shrubs (such as Ca and Mn; Fig. 2c) or decrease the potential annual foliar fluxes of mineral elements poorly concentrated in shrubs (such as Si, K, Fe, and Zn; Fig. 2c). While Ca is a non-limiting macronutrient for plants, Mn is a micronutrient sensitive to soil redox conditions that can influence the organic matter decomposition (Jones et al., 2020; Keiluweit et al., 2015). Soil saturation regime (Herndon et al., 2020) and the ability of plants and microorganisms to supply, accumulate, and regenerate short-lived Mn3+ species in the litter (Keiluweit et al., 2015) govern the forms of Mn present in the soil, which in turn affects litter decomposition efficiency. Upon Arctic shrubification, the potential increase in foliar Mn fluxes from plant to soil surface could therefore promote litter decomposition rate, a key factor in nutrient cycling and availability, plant growth, and terrestrial C balance, thereby contributing to amplify the permafrost C feedback (Schuur et al., 2015).
A parallel change in tundra vegetation composition is the expansion of sedges (as part of the graminoids) at localized sites across the Arctic and sub-Arctic regions, including the CiPEHR site at EML (Alaska, USA). At numerous warming sites, an increase in sedges (as E. vaginatum) occurred at the expense of shrubs during abrupt permafrost thaw, with pond development and wetter soil conditions (van der Kolk et al., 2016). While shrub growth can be limited by very wet soil conditions and low nutrient supply, sedges can develop in a wide range of soil moisture conditions thanks to their deeper rooting systems, and they are able to reach underlying soil layers and access deeper nutrient pools. At CiPEHR, the evolution of mineral element foliar stocks (Fig. 4) and potential fluxes (Fig. 6) with time demonstrated that nutrient uptake promoted by sedges (such as Si, P, and Fe) may positively influence the global mineral element storage in tundra plant tissues and thereby the transfer of mineral elements from plant leaves to soil litter. For example, we observed a large increase in Si, P, and Fe foliar stocks (Figs. 4a and S2) and potential annual foliar fluxes (Figs. 6a and S4) upon sedge expansion. While Si and Fe are non-essential plant nutrients, P is a limiting macronutrient for the vegetation (DalCorso et al., 2014; Marschner, 2012; Schachtman et al., 1998). In moist tundra ecosystems, P recycling from organic soils supplies most of the P taken up by plants (Shaver et al., 1991). Therefore, a potential increase in P input in the upper soil layers through higher annual P foliar fluxes may positively influence the global vegetation productivity. However, P mineralisation and availability may not simultaneously increase upon warmer soil conditions, and competition between vegetation and microbial demand for mineral nutrients can still severely limit plant P availability in Arctic soils (Jonasson et al., 1993; Nadelhoffer et al., 1991). Additional factors (such as litter decomposition rate) must be taken into account to evaluate the benefits from higher P litterfall cycling. Upon stimulated microbial activity, P bioavailability may evolve and directly influence vegetation growth and development. As vegetation is a key element of the total C budget, an increase in the cycling rates of limiting nutrients such as P may indirectly influence the role of vegetation in C sequestration through higher plant development and photosynthesis.
Finally, non-vascular species, such as mosses and lichens, dominate the groundcover biomass and productivity of many northern tundra ecosystems (Beringer et al., 2001; Longton, 1988; Oechel and Van Cleve, 1986; Viereck et al., 1986). Mosses display physiological and ecological traits that greatly influence soil thermal (Luthin and Guymon, 1974) and hydrological regimes (Zimov et al., 1995) but also plant nutrient availability (Cornelissen et al., 2007; Turetsky, 2003; Turetsky et al., 2010). Our low-scale study at the Gradient site highlighted the important moss contribution to mineral element foliar stocks (Figs. 7 and 8). Besides the important variability in moss biomass distribution across the Gradient site (with coefficients of variation ranging from 80 % at the Extensive thaw area up to more than 100 % at the Minimal thaw area; data from Jasinski et al., 2018), mosses showed a particularly high concentration in mineral elements such as Si, Al, and Fe (Fig. 2c, with coefficients of variation ranging around 24 %). Therefore, changes in moss biomass composition may influence nutrient cycling and availability and therefore future patterns of vegetation shift (Beringer et al., 2001).
In this study, we tested the influence of permafrost degradation on the mineral element foliar stocks and potential litterfall fluxes upon shifts to wetter and drier conditions in the sub-Arctic tundra vegetation. Our results at the species level showed that sedges (i.e., E. vaginatum and C. bigelowii) have relatively high Si, P, and Fe foliar concentrations, whereas shrubs (V. uliginosum, B. nana, V. vitis-idaea, and R. tomentosum) have relatively high Ca and Mn foliar concentrations. As a consequence, the main conclusions for the plant community are as follows.
Upon wetter soil conditions and sedge expansion, mineral elements that were highly concentrated in sedge foliar tissues (such as Si, P, and Fe) showed a large increase in their foliar stocks. This results mainly from the increase in sedge biomass across the site (CiPEHR). In parallel, the higher sedge foliar productivity generated higher annual maximum potential foliar transfers from plant to soil litter for those mineral elements (considering that fluxes of elements such as P are overestimates).
Upon drier soil conditions and shrubification, mineral elements highly concentrated in shrub foliar tissues (such as Ca and Mn) would increase in their total foliar stocks. This mainly results from the increase in the shrub contribution to their respective foliar stocks upon the early shrubification occurring across the thermokarst gradient site (Gradient). We expect a larger increase in the total foliar Ca and Mn stocks upon a wider shrubification. Similarly, the increasing shrub foliar productivity upon permafrost degradation promoted annual fluxes of Ca and Mn from plant to soil litter.
The spatial heterogeneity in permafrost soil degradation and soil moisture conditions leads to contrasted shifts in vegetation (sedge and shrub expansion) across the Arctic. We observed that mineral element cycling directly depends on the vegetation biomass and diversity. We therefore suggest that the different plant functional groups should be considered in future studies in order to more accurately predict the influence of vegetation shifts on biogeochemical cycling of the elements in Arctic ecosystems.
Here, we provided first estimations of potential mineral element fluxes from plant to soil via annual leaf fall, and we highlighted that shifts in vegetation (i.e., sedge or shrub expansion) influence the mineral element composition of the litter. To evaluate the implications of these results on tundra mineral nutrient cycling, future work would require integrating specific rates of litter decomposition and mineralisation through microbial activity with local shifts in vegetation biomass and productivity. As vegetation is central in the C balance regulation, there is a challenging need to assess to what extent the shift in vegetation and thereby in litter elemental composition will accelerate or slow down the litter decomposition (source of C emission) and how fast these mineral elements may be available again for vegetation uptake (source of C sequestration).
Elemental foliar stocks and potential litterfall fluxes presented in this paper are available in the Bonanza Creek LTER (CiPEHR dataset: https://doi.org/10.6073/pasta/597c40c5d699eec918da3e9c2eaa7bea, Mauclet et al., 2021a; and Gradient dataset: https://doi.org/10.6073/pasta/7fad9398ec3a596b8efc092fc8fbf55d, Mauclet et al., 2021b).
The supplement related to this article is available online at: https://doi.org/10.5194/bg-19-2333-2022-supplement.
EM, CH, AM, and SO planned the campaign. SO obtained the grant that funded this research. JL, MT, BLJ, and EAGS provided great help for the field work, supplied foliar samples, and shared massive datasets. EM, CH, AM, and MV performed the measurements. EM, YA, and SO analyzed the data. BP and AV helped with statistical analyses. EM wrote the manuscript. CH, SO, YA, MT, and EAGS reviewed and edited the manuscript. All authors gave final approval for publication.
The contact author has declared that neither they nor their co-authors have any competing interests.
Publisher’s note: Copernicus Publications remains neutral with regard to jurisdictional claims in published maps and institutional affiliations.
We warmly acknowledge the master students who participated to the data collection: Loïc Debry, Laurentine Debruxelles, and Simon Malvaux. Thanks to Anne Iserentant, Hélène Dailly, and Elodie Devos from the MOCA analytical platform at UCLouvain for mineral elemental analyses and to the SMCS platform from UCLouvain for the statistical support. We also greatly thank the Schuur Lab (Northern Arizona University) for their scientific support and the sample collection. We sincerely thank Jonathan von Oppen and the anonymous reviewer for their careful reading and helpful comments and suggestions to improve the manuscript.
This work was supported by the European Union's Horizon 2020 research and innovation program (grant agreement no. 714617, 2017–2022) and by the Fund for Scientific Research FNRS in Belgium to SO (grant no. FC69480).
This paper was edited by Anja Rammig and reviewed by Jonathan von Oppen and one anonymous referee.
Aerts, R. and Chapin, F. S.: The mineral nutrition of wild plants revisited: a re-evaluation of processes and patterns, in: Advances in Ecological Research, Academic Press, 1–67, https://doi.org/10.1016/S0065-2504(08)60016-1, 1999.
Aerts, R., Verhoeven, J. T. A., and Whigham, D. F.: Plant-mediated controls on nutrient cycling in temperate fens and bogs, Ecology, 80, 2170–2181, https://doi.org/10.1890/0012-9658(1999)080[2170:PMCONC]2.0.CO;2, 1999.
Bates, D., Mächler, M., Bolker, B., and Walker, S.: Fitting Linear Mixed-Effects Models Using lme4, J. Stat. Softw., 67, 1–48, https://doi.org/10.18637/jss.v067.i01, 2015.
Beermann, F., Langer, M., Wetterich, S., Strauss, J., Boike, J., Fiencke, C., Schirrmeister, L., Pfeiffer, E.-M., and Kutzbach, L.: Permafrost thaw and liberation of inorganic nitrogen in Eastern Siberia, Permafrost Periglac. Process., 28, 605–618, https://doi.org/10.1002/ppp.1958, 2017.
Berendse, F. and Jonasson, S.: Nutrient use and nutrient cycling in northern ecosystems, in: Chapin, F. S., Jefferies, R. L., Reynolds, J. F., Shaver, G. R., and Svoboda, J., Arctic Ecosystems in a Changing Climate: an Ecophysiological Perspective, Academic Press, Toronto, Canada, 337–356, 1992.
Beringer, J., Lynch, A. H., Chapin, F. S., Mack, M., and Bonan, G. B.: The representation of Arctic soils in the land surface model: the importance of mosses, J. Climate, 14, 3324–3335, https://doi.org/10.1175/1520-0442(2001)014<3324:TROASI>2.0.CO;2, 2001.
Bhatt, U. S., Walker, D. A., Raynolds, M. K., Bieniek, P. A., Epstein, H. E., Comiso, J. C., Pinzon, J. E., Tucker, C. J., Steele, M., Ermold, W., and Zhang, J.: Changing seasonality of panarctic tundra vegetation in relationship to climatic variables, Environ. Res. Lett., 12, 055003, https://doi.org/10.1088/1748-9326/aa6b0b, 2017.
Billings, W. D.: Constraints to plant growth, reproduction, and establishment in Arctic environments, Arctic Alpine Res., 19, 357–365, https://doi.org/10.2307/1551400, 1987.
Burn, C. R.: Permafrost, in: Encyclopedia of Quaternary Science, 2nd Edn., Elsevier, 464–471, https://doi.org/10.1016/B978-0-444-53643-3.00099-6, 2013.
Carey, J. C., Parker, T. C., Fetcher, N., and Tang, J.: Biogenic silica accumulation varies across tussock tundra plant functional type, Funct. Ecol., 31, 2177–2187, https://doi.org/10.1111/1365-2435.12912, 2017.
Carey, J. C., Abbott, B. W., and Rocha, A. V.: Plant uptake offsets silica release from a large Arctic tundra wildfire, Earths Future, 7, 1044–1057, https://doi.org/10.1029/2019EF001149, 2019.
Chapin, F. S.: The mineral nutrition of wild plants, Annu. Rev. Ecol. Syst., 11, 233–260, https://doi.org/10.1146/annurev.es.11.110180.001313, 1980.
Chapin, F. S. and Shaver, G. R.: Individualistic growth response of tundra plant species to environmental manipulations in the field, Ecology, 66, 564–576, https://doi.org/10.2307/1940405, 1985.
Chapin, F. S. and Shaver, G. R.: Differences in growth and nutrient use among Arctic plant growth forms, Funct. Ecol., 3, 73–80, https://doi.org/10.2307/2389677, 1989.
Chapin, F. S., Johnson, D. A., and McKendrick, J. D.: Seasonal movement of nutrients in plants of differing growth form in an alaskan tundra ecosystem: implications for herbivory, J. Ecol., 68, 189–209, 1980.
Chapin, F. S., Shaver, G. R., Giblin, A. E., Nadelhoffer, K. J., and Laundre, J. A.: Responses of Arctic tundra to experimental and observed changes in climate, Ecology 76, 694–711, https://doi.org/10.2307/1939337, 1995.
Chapin, F. S., Sturm, M., Serreze, M. C., Mcfadden, J. P., Key, J. R., Lloyd, A. H., McGuire, A. D., Rupp, T. S., Lynch, A. H., Schimel, J. P., Chapman, W. L., Epstein, H. E., Euskirchen, E. S., Hinzman, L. D., Jia, G., Ping, C. L., Tape, K. D., Thompson, C. D. C., Walker, D. A., and Welker, J. M.: Role of land-surface changes in Arctic summer warming, Science, 310, 657–660, https://doi.org/10.1126/science.1117368, 2005.
Cornelissen, J. H. C., van Bodegom, P. M., Aerts, R., Callaghan, T. V., van Logtestijn, R. S. P., Alatalo, J., Chapin, F. S., Gerdol, R., Gudmundsson, J., Gwynn-Jones, D., Hartley, A. E., Hik, D. S., Hofgaard, A., Jónsdóttir, I. S., Karlsson, S., Klein, J. A., Laundre, J., Magnusson, B., Michelsen, A., Molau, U., Onipchenko, V. G., Quested, H. M., Sandvik, S. M., Schmidt, I. K., Shaver, G. R., Solheim, B., Soudzilovskaia, N. A., Stenström, A., Tolvanen, A., Totland, Ø., Wada, N., Welker, J. M., and Zhao, X., and M.O.L. Team: Global negative vegetation feedback to climate warming responses of leaf litter decomposition rates in cold biomes, Ecol. Lett., 10, 619–627, https://doi.org/10.1111/j.1461-0248.2007.01051.x, 2007.
DalCorso, G., Manara, A., Piasentin, S., and Furini, A.: Nutrient metal elements in plants, Metallomics, 6, 1770–1788, https://doi.org/10.1039/C4MT00173G, 2014.
Deane-Coe, K. K., Mauritz, M., Celis, G., Salmon, V., Crummer, K. G., Natali, S. M., and Schuur, E. A. G.: Experimental warming alters productivity and isotopic signatures of tundra mosses, Ecosystems, 18, 1070–1082, https://doi.org/10.1007/s10021-015-9884-7, 2015.
Dormann, C. F. and Woodin, S. J.: Climate change in the Arctic: using plant functional types in a meta-analysis of field experiments, Funct. Ecol., 16, 4–17, https://doi.org/10.1046/j.0269-8463.2001.00596.x, 2002.
Druel, A., Ciais, P., Krinner, G., and Peylin, P.: Modeling the vegetation dynamics of northern shrubs and mosses in the ORCHIDEE Land Surface Model, J. Adv. Model. Earth Sy., 11, 2020–2035, https://doi.org/10.1029/2018MS001531, 2019.
Finger, R. A., Turetsky, M. R., Kielland, K., Ruess, R. W., Mack, M. C., and Euskirchen, E. S.: Effects of permafrost thaw on nitrogen availability and plant–soil interactions in a boreal Alaskan lowland, J. Ecol., 104, 1542–1554, https://doi.org/10.1111/1365-2745.12639, 2016.
French, H. M.: Permafrost, in: The Periglacial Environment, John Wiley & Sons Ltd., West Sussex, England, 83–115, https://doi.org/10.1002/9781118684931.ch5, 2013.
Heijmans, M. M. P. D., Magnússon, R. Í., Lara, M. J., Frost, G. V., Myers-Smith, I. H., van Huissteden, J., Jorgenson, M. T., Fedorov, A. N., Epstein, H. E., Lawrence, D. M., and Limpens, J.: Tundra vegetation change and impacts on permafrost, Nat. Rev. Earth Environ., 3, 68–84, https://doi.org/10.1038/s43017-021-00233-0, 2022.
Herndon, E., Kinsman-Costello, L., and Godsey, S.: Biogeochemical Cycling of Redox-Sensitive Elements in Permafrost-Affected Ecosystems, in: Biogeochemical Cycles, edited by: Dontsova, K., Balogh-Brunstad, Z., and Le Roux, G., AGU Geophysical Monograph Series, Wiley, 245–265, https://doi.org/10.1002/9781119413332.ch12, 2020.
Hewitt, R. E., Taylor, D. L., Genet, H., McGuire, A. D., and Mack, M. C.: Below-ground plant traits influence tundra plant acquisition of newly thawed permafrost nitrogen, J. Ecol., 107, 950–962, https://doi.org/10.1111/1365-2745.13062, 2019.
Hicks Pries, C. E., Schuur, E. A. G., and Crummer, K. G.: Holocene carbon stocks and carbon accumulation rates altered in soils undergoing permafrost thaw, Ecosystems, 15, 162–173, https://doi.org/10.1007/s10021-011-9500-4, 2012.
Hobbie, S. E.: Temperature and plant species control over litter decomposition in Alaskan tundra, Ecol. Monogr. 66, 503–522, https://doi.org/10.2307/2963492, 1996.
Hobbie, S. E. and Gough, L.: Foliar and soil nutrients in tundra on glacial landscapes of contrasting ages in northern Alaska, Oecologia 131, 453–462, https://doi.org/10.1007/s00442-002-0892-x, 2002.
Hodson, M. J., White, P. J., Mead, A., and Broadley, M. R.: Phylogenetic variation in the silicon composition of plants, Ann. Bot., 96, 1027–1046, https://doi.org/10.1093/aob/mci255, 2005.
IPCC: Climate Change 2014: Synthesis Report. Contribution of Working Groups I, II and III to the Fifth Assessment Report of the Intergovernmental Panel on Climate Change, edited by: Core Writing Team, Pachauri, R. K., and Meyer, L. A., IPCC, Geneva, Switzerland, 151 pp., 2014.
Jasinski, B. L., Hewitt, R. E., Mauritz, M., Miller, S. N., Schuur, E. A. G., Taylor, M. A., Walker, X. J., and Mack, M. C.: Plant foliar nutrient response to active layer and water table depth in warming permafrost soils, J. Ecol., https://doi.org/10.1111/1365-2745.13864, online first, 2022.
Jasinski, B. L., Schuur, E. A. G., Mack, M. C., and Bonanza Creek LTER: Eight Mile Lake Research Watershed, Thaw Gradient: peak growing season aboveground biomass 2017, Environmental Data Initiative [data set], https://doi.org/10.6073/PASTA/40F9AE60D635E5AAC0E562EE006D24E2, 2018.
Jonasson, S.: Nutrient content and dynamics in north Swedish shrub tundra areas, Ecography, 6, 295–304, https://doi.org/10.1111/j.1600-0587.1983.tb01093.x, 1983.
Jonasson, S. and Chapin, F. S.: Significance of sequential leaf development for nutrient balance of the cotton sedge, Eriophorum vaginatum L., Oecologia, 67, 511–518, https://doi.org/10.1007/BF00790022, 1985.
Jonasson, S., Havström, M., Jensen, M., and Callaghan, T. V.: In situ mineralization of nitrogen and phosphorus of arctic soils after perturbations simulating climate change, Oecologia, 95, 179–186, https://doi.org/10.1007/BF00323488, 1993.
Jonasson, S., Michelsen, A., and Schmidt, I. K.: Coupling of nutrient cycling and carbon dynamics in the Arctic, integration of soil microbial and plant processes, Appl. Soil Ecol., 11, 135–146, https://doi.org/10.1016/S0929-1393(98)00145-0, 1999.
Jones, M. E., La Croix, R. E., Zeigler, J., Ying, S. C., Nico, P. S., and Keiluweit, M.: Enzymes, manganese, or iron? Drivers of oxidative organic matter decomposition in soils, Environ. Sci. Technol., 54, 14114–14123, https://doi.org/10.1021/acs.est.0c04212, 2020.
Jonsdottir, I. S., Magnusson, B., Gudmundsson, J., Elmarsdottir, A., and Hjartarson, H.: Variable sensitivity of plant communities in Iceland to experimental warming, Glob. Change Biol., 11, 553–563, https://doi.org/10.1111/j.1365-2486.2005.00928.x, 2005.
Jorgenson, M. T., Racine, C. H., Walters, J. C., and Osterkamp, T. E.: Permafrost degradation and ecological changes associated with a warming climate in Central Alaska, Climatic Change, 48, 551–579, https://doi.org/10.1023/A:1005667424292, 2001.
Jorgenson, J. C., Raynolds, M. K., Reynolds, J. H., and Benson, A. M.: Twenty-five year record of changes in plant cover on tundra of Northeastern Alaska, Arct. Antarct. Alp. Res., 47, 785–806, https://doi.org/10.1657/AAAR0014-097, 2015.
Keiluweit, M., Nico, P., Harmon, M. E., Mao, J., Pett-Ridge, J., and Kleber, M.: Long-term litter decomposition controlled by manganese redox cycling, P. Natl. Acad. Sci. USA, 112, E5253–E5260, https://doi.org/10.1073/pnas.1508945112, 2015.
Keuper, F., Bodegom, P. M., Dorrepaal, E., Weedon, J. T., Hal, J., Logtestijn, R. S. P., and Aerts, R.: A frozen feast: thawing permafrost increases plant-available nitrogen in subarctic peatlands, Glob. Change Biol., 18, 1998–2007, https://doi.org/10.1111/j.1365-2486.2012.02663.x, 2012.
Keuper, F., Dorrepaal, E., van Bodegom, P.M., van Logtestijn, R., Venhuizen, G., van Hal, J., and Aerts, R.: Experimentally increased nutrient availability at the permafrost thaw front selectively enhances biomass production of deep-rooting subarctic peatland species, Glob. Change Biol., 23, 4257–4266, https://doi.org/10.1111/gcb.13804, 2017.
Lavoie, M., Mack, M. C., and Schuur, E. A. G.: Effects of elevated nitrogen and temperature on carbon and nitrogen dynamics in Alaskan arctic and boreal soils, J. Geophys. Res.-Biogeo., 116, G03013, https://doi.org/10.1029/2010JG001629, 2011.
Leroux, S. J., Wal, E. V., Wiersma, Y. F., Charron, L., Ebel, J. D., Ellis, N. M., Hart, C., Kissler, E., Saunders, P. W., Moudrá, L., Tanner, A. L., and Yalcin, S.: Stoichiometric distribution models: ecological stoichiometry at the landscape extent, Ecol. Lett., 20, 1495–1506, https://doi.org/10.1111/ele.12859, 2017.
Loneragan, J. F. and Snowball, K.: Calcium requirements of plants, Aust. J. Agr. Res., 20, 465–478, https://doi.org/10.1071/AR9690465, 1969.
Longton, R. E. (Ed.): Cryptogams in polar ecosystems, in: Biology of Polar Bryophytes and Lichens, Studies in Polar Research, 253–309, Cambridge University Press, https://doi.org/10.1017/CBO9780511565212.008, 1988.
Luthin, J. N. and Guymon, G. L.: Soil moisture-vegetation-temperature relationships in Central Alaska, J. Hydrol., 23, 233–246, https://doi.org/10.1016/0022-1694(74)90005-5, 1974.
Ma, J. F. and Takahashi, E. (Eds.): Soil, fertilizer, and plant silicon research in Japan, Elsevier, Amsterdam, p. 294, https://doi.org/10.1016/B978-0-444-51166-9.X5000-3, 2002.
Mack, M. C., Schuur, E. A. G., Bret-Harte, M. S., Shaver, G. R., and Chapin, F. S.: Ecosystem carbon storage in arctic tundra reduced by long-term nutrient fertilization, Nature, 431, 440–443, https://doi.org/10.1038/nature02887, 2004.
Mack, M. C., Finlay, J. C., DeMarco, J., Chapin, F., Schuur, E. A. G., Neff, J. C., and Zimov, S. A.: Nitrogen and phosphorus in Yedoma soils of Northeast Siberia: stocks, fluxes and the ecosystem consequences of nutrient release from permafrost thaw, Presented at the American Geophysical Union, Fall Meeting 2010, December 2010, San Fransisco, California, abstract id. GC52A-05, 2010.
Maillard, A., Diquélou, S., Billard, V., Laîné, P., Garnica, M., Prudent, M., Garcia-Mina, J.-M., Yvin, J.-C., and Ourry, A.: Leaf mineral nutrient remobilization during leaf senescence and modulation by nutrient deficiency, Front. Plant Sci., 6, 317, https://doi.org/10.3389/fpls.2015.00317, 2015.
Marschner, H.: Marschner's mineral nutrition of higher plants, 3rd Edn., Elsevier, Academic Press, London, Waltham, MA, https://doi.org/10.1016/C2009-0-63043-9, 2012.
Mauclet, E., Opfergelt, S., Agnan, Y., Hirst, C., Monhonval, A., Ledman, J., Taylor, M., Schuur, E. A. G., and Bonanza Creek LTER: Carbon in Permafrost Experimental Heating Research (CIPEHR) project: Foliar mineral element concentrations, stocks, and annual litterfall fluxes in July 2009 and 2017, Environmental Data Initiative [data set], https://doi.org/10.6073/pasta/597c40c5d699eec918da3e9c2eaa7bea, 2021a.
Mauclet, E., Opfergelt, S., Agnan, Y., Hirst, C., Monhonval, A., Ledman, J., Taylor, M., Schuur, E. A. G., and Bonanza Creek LTER: Eight Mile Lake Research Watershed, Thaw Gradient: Foliar mineral element concentrations, stocks and annual litterfall fluxes estimated for July 2017, Environmental Data Initiative [data set] 5, https://doi.org/10.6073/pasta/7fad9398ec3a596b8efc092fc8fbf55d, 2021b.
Mauritz, M., Bracho, R., Celis, G., Hutchings, J., Natali, S. M., Pegoraro, E., Salmon, V. G., Schädel, C., Webb, E. E., and Schuur, E. A. G.: Nonlinear CO2 flux response to 7 years of experimentally induced permafrost thaw, Glob. Change Biol., 23, 3646–3666, https://doi.org/10.1111/gcb.13661, 2017.
Myneni, R. B., Keeling, C. D., Tucker, C. J., Asrar, G., and Nemani, R. R.: Increased plant growth in the northern high latitudes from 1981 to 1991, Nature, 386, 698–702, https://doi.org/10.1038/386698a0, 1997.
Nadelhoffer, K. J., Giblin, A. E., Shaver, G. R., Laundre, J. A.: Effects of temperature and substrate quality on element mineralization in six Arctic soils, Ecology, 72, 242–253, https://doi.org/10.2307/1938918, 1991.
Natali, S. M., Schuur, E. A. G., Trucco, C., Hicks Pries, C. E., Crummer, K. G., and Baron Lopez, A. F.: Effects of experimental warming of air, soil and permafrost on carbon balance in Alaskan tundra, Glob. Change Biol., 17, 1394–1407, https://doi.org/10.1111/j.1365-2486.2010.02303.x, 2011.
Natali, S. M., Schuur, E. A. G., and Rubin, R. L.: Increased plant productivity in Alaskan tundra as a result of experimental warming of soil and permafrost: Increased plant productivity in Alaskan tundra, J. Ecol., 100, 488–498, https://doi.org/10.1111/j.1365-2745.2011.01925.x, 2012.
Natali, S. M., Watts, J. D., Rogers, B. M., Potter, S., Ludwig, S. M., Selbmann, A. K., Sullivan, P. F., Abbott, B. W., Arndt, K. A., Birch, L., Björkman, M. P., Bloom, A. A., Celis, G., Christensen, T. R., Christiansen, C. T., Commane, R., Cooper, E. J., Crill, P., Czimczik, C., Davydov, S., Du, J., Egan, J. E., Elberling, B., Euskirchen, E. S., Friborg, T., Genet, H., Göckede, M., Goodrich, J. P., Grogan, P., Helbig, M., Jafarov, E. E., Jastrow, J. D., Kalhori, A. A. M., Kim, Y., Kimball, J. S., Kutzbach, L., Lara, M. J., Larsen, K. S., Lee, B.-Y., Liu, Z., Loranty, M. M., Lund, M., Lupascu, M., Madani, N., Malhotra, A., Matamala, R., McFarland, J., McGuire, A. D., Michelsen, A., Minions, C., Oechel, W. C., Olefeldt, D., Parmentier, F.-J. W., Pirk, N., Poulter, B., Quinton, W., Rezanezhad, F., Risk, D., Sachs, T., Schaefer, K., Schmidt, N. M., Schuur, E. A. G., Semenchuk, P. R., Shaver, G., Sonnentag, O., Starr, G., Treat, C. C., Waldrop, M. P., Wang, Y., Welker, J., Wille, C., Xu, X., Zhang, Z., Zhuang, Q., and Zona, D.: Large loss of CO2 in winter observed across the northern permafrost region, Nat. Clim. Change, 9, 852–857, https://doi.org/10.1038/s41558-019-0592-8, 2019.
Oechel, W. C. and Van Cleve, K.: The role of bryophytes in nutrient cycling in the taiga, in: Forest Ecosystems in the Alaskan Taiga, edited by: Van Cleve, K., Chapin, F. S., Flanagan, P. W., Viereck, L. A., and Dyrness, C. T., Ecological Studies, Springer New York, New York, NY, 121–137, https://doi.org/10.1007/978-1-4612-4902-3_9, 1986.
Osterkamp, T. E., Jorgenson, M. T., Schuur, E. A. G., Shur, Y. L., Kanevskiy, M. Z., Vogel, J. G., and Tumskoy, V. E.: Physical and ecological changes associated with warming permafrost and thermokarst in Interior Alaska, Permafrost Periglac., 20, 235–256, https://doi.org/10.1002/ppp.656, 2009.
Park, B. B., Rahman, A., Han, S. H., Youn, W. B., Hyun, H. J., Hernandez, J., and An, J. Y.: Carbon and nutrient inputs by litterfall in evergreen and deciduous forests in Korea, Forests, 11, 143, https://doi.org/10.3390/f11020143, 2020.
Parker, T. C., Sanderman, J., Holden, R. D., Blume-Werry, G., Sjögersten, S., Large, D., Castro-Díaz, M., Street, L. E., Subke, J. A., and Wookey, P. A.: Exploring drivers of litter decomposition in a greening Arctic: results from a transplant experiment across a treeline, Ecology, 99, 2284–2294, https://doi.org/10.1002/ecy.2442, 2018.
Pearson, R. G., Phillips, S. J., Loranty, M. M., Beck, P. S. A., Damoulas, T., Knight, S. J., and Goetz, S. J.: Shifts in Arctic vegetation and associated feedbacks under climate change, Nat. Clim. Change, 3, 673–677, https://doi.org/10.1038/nclimate1858, 2013.
Pouliot, D., Latifovic, R., and Olthof, I.: Trends in vegetation NDVI from 1 km AVHRR data over Canada for the period 1985–2006, Int. J. Remote Sens., 30, 149–168, https://doi.org/10.1080/01431160802302090, 2009.
Quigley, K. M., Donati, G. L., and Anderson, T. M.: Variation in the soil “silicon landscape” explains plant silica accumulation across environmental gradients in Serengeti, Plant Soil, 410, 217–229, https://doi.org/10.1007/s11104-016-3000-4, 2017.
Ravansari, R. and Lemke, L. D.: Portable X-ray fluorescence trace metal measurement in organic rich soils: pXRF response as a function of organic matter fraction, Geoderma, 119, 175–184, https://doi.org/10.1016/j.geoderma.2018.01.011, 2018.
Ravansari, R., Wilson, S. C., and Tighe, M.: Portable X-ray fluorescence for environmental assessment of soils: not just a point and shoot method, Environ. Int., 134, 105250, https://doi.org/10.1016/j.envint.2019.105250, 2020.
R Core Team: R: A language and environment for statistical computing. R Foundation for Statistical Computing, Vienna, Austria, https://www.R-project.org/ (last access: December 2021), 2020.
Reimann, C., Koller, F., Kashulina, G., Niskavaara, H., and Englmaier, P.: Influence of extreme pollution on the inorganic chemical composition of some plants, Environ. Pollut., 115, 239–252, https://doi.org/10.1016/S0269-7491(01)00106-3, 2001.
Rodenhizer, H., Ledman, J., Mauritz, M., Natali, S. M., Pegoraro, E., Plaza, C., Romano, E., Schädel, C., Taylor, M., and Schuur, E. A. G.: Carbon thaw rate doubles when accounting for subsidence in a permafrost warming experiment, J. Geophys. Res.-Biogeo., 125, e2019JG005528, https://doi.org/10.1029/2019JG005528, 2020.
Rustad, L., Campbell, J., Marion, G., Norby, R., Mitchell, M., Hartley, A., Gurevitch, J., and Cornelissen, J.: A meta-analysis of the response of soil respiration, net nitrogen mineralization, and aboveground plant growth to experimental ecosystem warming, Oecologia, 126, 543–562, https://doi.org/10.1007/s004420000544, 2001.
Salmon, V. G., Soucy, P., Mauritz, M., Celis, G., Natali, S. M., Mack, M. C., and Schuur, E. A. G.: Nitrogen availability increases in a tundra ecosystem during five years of experimental permafrost thaw, Glob. Change Biol., 22, 1927–1941, https://doi.org/10.1111/gcb.13204, 2016.
Schachtman, D. P., Reid, R. J., and Ayling, S. M.: Phosphorus uptake by plants: from soil to cell, Plant Physiol., 116, 447–453, https://doi.org/10.1104/pp.116.2.447, 1998.
Schuur, E. A. G. and Mack, M. C.: Ecological response to permafrost thaw and consequences for local and global ecosystem services, Annu. Rev. Ecol. Evol. Syst., 49, 279–301, https://doi.org/10.1146/annurev-ecolsys-121415-032349, 2018.
Schuur, E. A. G., Crummer, K. G., Vogel, J. G., and Mack, M. C.: Plant species composition and productivity following permafrost thaw and thermokarst in Alaskan tundra, Ecosystems, 10, 280–292, https://doi.org/10.1007/s10021-007-9024-0, 2007.
Schuur, E. A. G., Bockheim, J., Canadell, J. G., Euskirchen, E., Field, C. B., Goryachkin, S. V., Hagemann, S., Kuhry, P., Lafleur, P. M., Lee, H., Mazhitova, G., Nelson, F. E., Rinke, A., Romanovsky, V. E., Shiklomanov, N., Tarnocai, C., Venevsky, S., Vogel, J. G., and Zimov, S. A.: Vulnerability of permafrost carbon to climate change: implications for the global carbon cycle, BioScience, 58, 701–714, https://doi.org/10.1641/B580807, 2008.
Schuur, E. A. G., Vogel, J. G., Crummer, K. G., Lee, H., Sickman, J. O., and Osterkamp, T. E.: The effect of permafrost thaw on old carbon release and net carbon exchange from tundra, Nature, 459, 556–559, https://doi.org/10.1038/nature08031, 2009.
Schuur, E. A. G., McGuire, A. D., Schädel, C., Grosse, G., Harden, J. W., Hayes, D. J., Hugelius, G., Koven, C. D., Kuhry, P., Lawrence, D. M., Natali, S. M., Olefeldt, D., Romanovsky, V. E., Schaefer, K., Turetsky, M. R., Treat, C. C., and Vonk, J. E.: Climate change and the permafrost carbon feedback, Nature, 520, 171–179, https://doi.org/10.1038/nature14338, 2015.
Schuur, E. A. G., Crummer, K. G., and Bonanza Creek LTER: Eight Mile Lake Research Watershed, Thaw Gradient: Plant species composition and productivity 2004, Environmental Data Initiative [data set], https://doi.org/10.6073/pasta/141d911e791ee2c2903884f8719f5a3e, 2016.
Shaver, G. R.: Integrated ecosystem research in northern Alaska, 1947–1994, in: Landscape Function and Disturbance in Arctic Tundra, Ecological Studies, edited by: Reynolds, J. F. and Tenhunen, J. D., Springer Berlin Heidelberg, 19–34, https://doi.org/10.1007/978-3-662-01145-4_2, 1996.
Shaver, G. R. and Chapin, F. S.: Production: biomass relationships and element cycling in contrasting Arctic vegetation types, Ecol. Monogr., 61, 1–31, https://doi.org/10.2307/1942997, 1991.
Shaver, G. R., Nadelhoffer, K. J., and Giblin, A. E.: Biogeochemical diversity and element transport in a heterogeneous landscape, the north slope of Alaska, Ecol. Stud., 82, 105–115, 1991.
Shaver, G. R., Billings, W. D., Chapin, F. S., Giblin, A. E., Nadelhoffer, K. J., Oechel, W. C., and Rastetter, E. B.: Global change and the carbon balance of Arctic ecosystems, BioScience, 42, 433–441, https://doi.org/10.2307/1311862, 1992.
Shaver, G. R., Bret-Harte, M. S., Jones, M. H., Johnstone, J., Gough, L., Laundre, J., and Chapin, F. S.: Species composition interacts with fertilizer to control long-term change in tundra productivity, Ecology, 82, 3163–3181, https://doi.org/10.1890/0012-9658(2001)082[3163:SCIWFT]2.0.CO;2, 2001.
Shi, R., Bäßler, R., Zou, C., and Römheld, V.: Is iron phloem mobile during senescence in trees? A reinvestigation of Rissmüller's finding of 1874, Plant Physiol. Biochem., 49, 489–493, https://doi.org/10.1016/j.plaphy.2011.03.004, 2011.
Sistla, S. A., Moore, J. C., Simpson, R. T., Gough, L., Shaver, G. R., and Schimel, J. P.: Long-term warming restructures Arctic tundra without changing net soil carbon storage, Nature, 497, 615–618, https://doi.org/10.1038/nature12129, 2013.
Sturm, M., Mcfadden, J. P., Liston, G. E., Chapin, F. S., Racine, C. H., and Holmgren, J.: Snow–shrub interactions in Arctic tundra: a hypothesis with climatic implications, J. Climate, 14, 336–344, https://doi.org/10.1175/1520-0442(2001)014<0336:SSIIAT>2.0.CO;2, 2001a.
Taylor, M., Schuur, E. A. G., Mauritz, M., Pegoraro, E. F., Salmon, V. G., Natali, S. M. N., and Bonanza Creek LTER: Eight Mile Lake Research Watershed, Carbon in Permafrost Experimental Heating Research (CiPEHR): aboveground plant biomass, 2009–2017, Environmental Data Initiative [data set], https://doi.org/10.6073/PASTA/1AF376985D83CD7E01C61B67ABFA9F91, 2018.
Turetsky, M. R.: The Role of Bryophytes in Carbon and Nitrogen Cycling, Bryologist, 106, 395–409, https://doi.org/10.1639/05, 2003.
Turetsky, M. R., Mack, M. C., Hollingsworth, T. N., and Harden, J. W.: The role of mosses in ecosystem succession and function in Alaska's boreal forest, Can. J. Forest Res., 40, 1237–1264, https://doi.org/10.1139/X10-072, 2010.
Urbina, I., Sardans, J., Grau, O., Beierkuhnlein, C., Jentsch, A., Kreyling, J., and Peñuelas, J.: Plant community composition affects the species biogeochemical niche, Ecosphere, 8, e01801, https://doi.org/10.1002/ecs2.1801, 2017.
van der Kolk, H.-J., Heijmans, M. M. P. D., van Huissteden, J., Pullens, J. W. M., and Berendse, F.: Potential Arctic tundra vegetation shifts in response to changing temperature, precipitation and permafrost thaw, Biogeosciences, 13, 6229–6245, https://doi.org/10.5194/bg-13-6229-2016, 2016.
Van Wijk, M. T. and Williams, M.: Interannual variability of plant phenology in tussock tundra: modelling interactions of plant productivity, plant phenology, snowmelt and soil thaw, Glob. Change Biol., 9, 743–758, https://doi.org/10.1046/j.1365-2486.2003.00625.x, 2003.
Viereck, L. A., Van Cleve, K., and Dyrness, C. T.: Forest ecosystem distribution in the taiga environment, in: Forest Ecosystems in the Alaskan Taiga, edited by: Van Cleve, K., Chapin, F. S., Flanagan, P. W., Viereck, L. A., and Dyrness, C. T., Ecological Studies, Springer New York, New York, NY, 22–43, https://doi.org/10.1007/978-1-4612-4902-3_3, 1986.
Viers, J., Prokushkin, A. S., Pokrovsky, O. S., Auda, Y., Kirdyanov, A. V., Beaulieu, E., Zouiten, C., Oliva, P., and Dupré, B.: Seasonal and spatial variability of elemental concentrations in boreal forest larch foliage of Central Siberia on continuous permafrost, Biogeochemistry, 113, 435–449, https://doi.org/10.1007/s10533-012-9770-8, 2013.
Vitt, D. H.: Estimating moss and lichen ground layer net primary production in tundra, peatlands, and forests, in: Principles and Standards for Measuring Primary Production Long-Term Ecological Research Network Series, edited by: Fahey, T. J. and Knapp, A. K., Oxford University Press, p. 288, https://doi.org/10.1093/acprof:oso/9780195168662.003.0006, 2007.
Vogel, J., Schuur, E. A. G., Trucco, C., and Lee, H.: Response of CO2 exchange in a tussock tundra ecosystem to permafrost thaw and thermokarst development, J. Geophys. Res., 114, G04018, https://doi.org/10.1029/2008JG000901, 2009.
Walker, D. A., Raynolds, M. K., Daniëls, F. J. A., Einarsson, E., Elvebakk, A., Gould, W. A., Katenin, A. E., Kholod, S. S., Markon, C. J., Melnikov, E. S., Moskalenko, N. G., Talbot, S. S., Yurtsev, B. A., and the other members of the CAVM Team: The Circumpolar Arctic vegetation map, J. Veg. Sci., 16, 267–282, https://doi.org/10.1111/j.1654-1103.2005.tb02365.x, 2005.
Walker, M. D., Wahren, C. H., Hollister, R. D., Henry, G. H. R., Ahlquist, L. E., Alatalo, J. M., Bret-Harte, M. S., Calef, M. P., Callaghan, T. V., Carroll, A. B., and Epstein, H. E.: Plant community responses to experimental warming across the tundra biome, P. Natl. Acad. Sci. USA, 103, 1342–1346, https://doi.org/10.1073/pnas.0503198103, 2006.
Washburn, A. L. (Ed.): Geocryology: a survey of periglacial processes and environments, 2nd Edn., Wiley, New York, NY, ISBN-10 193284628X, 1980.
Weintraub, M. N. and Schimel, J. P.: Nitrogen cycling and the spread of shrubs control changes in the carbon balance of Arctic tundra ecosystems, BioScience, 55, 408–415, https://doi.org/10.1641/0006-3568(2005)055[0408:NCATSO]2.0.CO;2, 2005.
White, P. J.: Long-distance transport in the xylem and phloem, in: Marschner's Mineral Nutrition of Higher Plants, edited by: Marschner, P., Elsevier, 49–70, https://doi.org/10.1016/B978-0-12-384905-2.00003-0, 2012.
White, P. J. and Broadley, M. R.: Calcium in plants, Ann. Bot., 92, 487–511, https://doi.org/10.1093/aob/mcg164, 2003.
Wickham, H. (Ed.): ggplot2: elegant graphics for data analysis, 2nd Edn., Use R, Springer, New York, NY, 2016.
Wookey, P. A., Aerts, R., Bardgett, R. D., Baptist, F., Bråthen, K. A., Cornelissen, J. H. C., Gough, L., Hartley, I. P., Hopkins, D. W., Lavorel, S., and Shaver, G. R.: Ecosystem feedbacks and cascade processes: understanding their role in the responses of Arctic and alpine ecosystems to environmental change, Glob. Change Biol., 15, 1153–1172, https://doi.org/10.1111/j.1365-2486.2008.01801.x, 2009.
Xu, L., Myneni, R. B., Chapin, F. S., Callaghan, T. V., Pinzon, J. E., Tucker, C. J., Zhu, Z., Bi, J., Ciais, P., Tømmervik, H., Euskirchen, E. S., Forbes, B. C., Piao, S. L., Anderson, B. T., Ganguly, S., Nemani, R. R., Goetz, S. J., Beck, P. S. A., Bunn, A. G., Cao, C., and Stroeve, J. C.: Temperature and vegetation seasonality diminishment over northern lands, Nat. Clim. Change, 3, 581–586, https://doi.org/10.1038/nclimate1836, 2013.
Zamin, T. J., Bret-Harte, M. S., and Grogan, P.: Evergreen shrubs dominate responses to experimental summer warming and fertilization in Canadian mesic low arctic tundra, J. Ecol., 102, 749–766, https://doi.org/10.1111/1365-2745.12237, 2014.
Zimov, S. A., Chuprynin, V. I., Oreshko, A. P., Chapin, F. S., Reynolds, J. F., and Chapin, M. C.: Steppe-tundra transition: a herbivore-driven biome shift at the end of the Pleistocene, Am. Nat., 146, 765–794, https://doi.org/10.1086/285824, 1995.